- 1Retired, Greenland, NH, United States
- 2Retired, Philadelphia, PA, United States
- 3Global CO2 Initiative, Department of Mechanical Engineering, University of Michigan, Ann Arbor, MI, United States
Carbon dioxide capture, utilization, and sequestration (CCUS) is a collection of approaches needed to supplement other efforts to achieve net zero carbon emissions. The specific combination of CO2 sources and sinks (a “usage pathway”) determines the environmental impact, economic viability, overall role in climate change mitigation and continued availability of carbon-based products. Optimal deployment requires a clear understanding of the nature of carbon sources and the durability and economic value of downstream processes and materials. Rigorous life cycle and techno-economic assessments (LCA and TEA) are critical. This paper presents a CO2 sources and sinks matrix as the high-level basis for assessing a usage pathway's climate relevance and economics.
Introduction
Atmospheric and oceanic carbon dioxide concentrations are at an all-time high and causing well-documented damage to climate, habitats, and communities (Masson-Delmotte et al., 2018). Stabilizing the climate, and providing equitable access to carbon-based materials, is an urgent societal challenge. The use of fossil carbon (coal, oil, natural gas) needs to be phased out as quickly as possible, but without jeopardizing access to energy and carbon-based materials while alternatives are being developed and deployed. Business-as-usual, that continues to add CO2 to the atmosphere and oceans, is simply unsustainable. Rapid de-fossilization of the world's energy and materials systems is imperative. During the transition, CO2 from fossil carbon point sources must be eliminated or captured to prevent additional carbon from entering the atmosphere. However, this will not be adequate. At least some fraction of the CO2 that has already accumulated in the atmosphere and oceans must be removed (Masson-Delmotte et al., 2018; International Energy Agency, 2020, 2022; Ramanathan et al., 2021; Author Collective, 2022). Once CO2 has been captured from air or water, it needs to be handled in a manner that has no further adverse effect on climate, ecosystems or human health.
Connecting CO2 sources with sinks creates “usage pathways” that are often collectively called CCUS. Each pathway has a specific potential impact on the climate, ecosystems, economics, and communities. However, outcomes are uncertain and may be conflicting, leaving decision makers in a difficult position for action (Harvey, 2023a,b). Region-specific, multifactorial assessments are needed for any proposed usage pathway to determine the appropriate solutions, and these will differ from one location to another (Global CO2 Initiative, 2023).
In considering CCUS, it is important to understand the salient features of carbon capture, carbon sequestration, and carbon utilization:
Carbon capture is the process by which carbon oxides (CO and CO2) are isolated from dilute mixtures, such as air, water, or flue gas (Wilcox, 2012; Wilcox et al., 2017; Aines et al., 2021). Direct Air Capture (DAC) processes isolate CO2 from ambient air, Direct Ocean Capture (DOC) extracts CO2 from water (National Academies of Sciences Engineering, 2022), and flue gas capture removes CO2 from a point source emitter in a place such as a cement plant, natural gas plant, or ethanol fermentation plants (Rao and Rubin, 2002).
Carbon sequestration entails storage in geological, mostly underground reservoirs with or without mineralization to carbonates. Key attributes of CO2 sequestration include carbon removal at geological time scales and potential volumes exceeding 100,000s of gigatonnes of CO2 (Aines et al., 2021).
Sequestration from point sources of direct fossil CO2 emissions currently requires societal subsidies in the form of tax credits, or penalties, such as a tax on carbon, or increased prices for e. g., electricity from power plants to cover the expenses of operation.
Sequestration could generate revenue from the sale of carbon credits if the CO2 is taken from air or water.
Carbon utilization takes the carbon oxides as a raw material to convert them to products. This conversion into useful products could offer several advantages over sequestration in geologic formations. However, it is essential to note that not all utilization processes will constitute net-negative carbon performance as will be detailed in the remainder of this perspective. The framework that is described shows how the source of the carbon and the lifetime of the resulting products are amongst the key factors to determine the net impact on carbon balances (Sick et al., 2022b). A clear understanding of these connections will help decision makers to select best options.
Conversion of CO2 creates products that generate revenue from their sales to help cover the increased cost of production from capturing and using CO2. Some carbon utilization products can simultaneously generate revenue from the sale of carbon credits. While of lower CO2 storage capacity than sequestration overall, the annual utilization potential is sufficient in the long term to more than adequately handle inevitable CO2 emissions from hard-to-abate industrial sectors and the use or decomposition of short-lived CO2 products at the several gigatonne/year level (Hepburn et al., 2019; International Energy Agency, 2020; Sick, 2021; Mertens et al., 2023; Newman et al., 2023).
Enhanced oil recovery without CO2 recycling could be a path to remove some CO2 while oil production is still necessary during the transition to a fossil-free future.
Some technologies for CO2 capture and conversion are ready for deployment, while others will require substantial R&D investments (Committee on Carbon Utilization Infrastructure, 2022). However, cost of installation and operation can be a significant barrier to rapid market introduction even for more mature technologies. As a result, projections for the CO2 utilization potential and the associated revenue cover a substantial range as summarized in Figure 1 (Sick et al., 2022a).
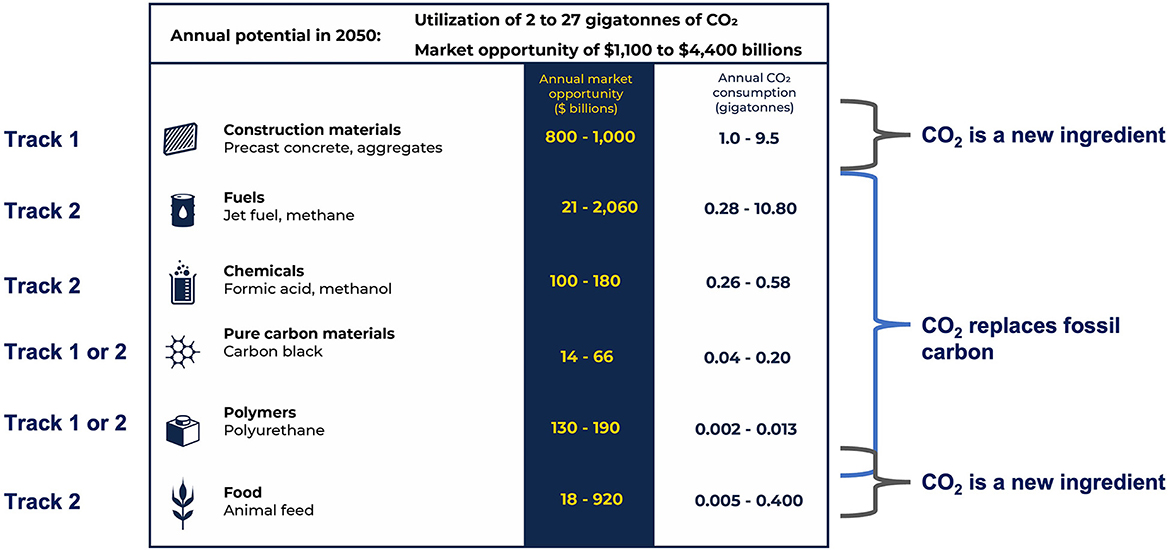
Figure 1. The environmental and economic potential for CO2-based products is very large. Data are taken from Sick et al. (2022a).
Understanding the potential climate impact of CO2-based products is aided by the concept of Track 1 and Track 2 products (Sick et al., 2022b). The distinction between Track 1 and Track 2 is in the anticipated product lifetime and associated time during which the underlying CO2 is removed from the environment. Track 1 products have lifetimes of at least 100 years, with potential lifetimes of thousands of years for some polymer materials and some construction materials. In contrast, Track 2 products are consumed or decompose in <100 years. They thus re-release CO2 on a time scale that has different climate implications (Sick et al., 2022b).
Figure 1 also indicates the role of CO2 for a particular product category. For example, traditionally, concrete or aggregates production did not entail mineralization of CO2. CO2-based concrete and aggregates are therefore a new carbon removal opportunity that durably stores the carbon similar to geologic storage, but with the benefit that these products have intrinsic commercial value. The financial viability of this pathway is thus not solely dependent on revenues from tax credits and/or the sale of carbon credits. CO2-based construction materials must overcome several market entrance barriers, as product costs and material properties will differ from the incumbent products. In contrast, material properties for Track 2 products (e.g., chemicals, fuels and other materials) will be identical to the incumbent products. Market adoption will thus likely be a function of availability and price competitiveness. Direct conversion of CO2 to food, not using plants, and other agricultural products represent a new set of opportunities for CO2 use (Mishra et al., 2002; Pander et al., 2002; Meessen, 2014; Sillman et al., 2019; Ruuskanen et al., 2021). Competitive costs and satisfactory performance (e.g., taste) will be key to market acceptance. CO2-based food for human consumption may face additional consumer acceptance challenges (Lutzke and Árvai, 2021).
CO2 sources and sinks matrix
CCUS technologies include a wide range of options, and this creates a complex and confusing situation even for experts. Tools such as life cycle assessments (LCA) and techno-economic assessments (TEA) are available and are essential for evaluating the climate impact, technical feasibility, and economic viability of these technologies. Enhancements of these tools are under development and will permit rigorous assessments and guidance for societal acceptance (Zimmermann et al., 2018; National Energy Technology Laboratory, 2022; Global CO2 Initiative, 2023; Sick et al., 2023). Apart from the technologies, specific combinations of sources and sinks play a key role in determining the effects on climate, ecosystems, economics, and communities. Therefore, it is useful to compile a higher-level matrix that provides insights into figures of merit at a qualitative level. The key figures of merit in this discussion are climate benefits, i.e., the impact on ambient CO2 levels, and the economic outcomes. The matrix provides a starting point for technology considerations, clarity in terms of maximum potential or risk, and a common framework for decision makers. It must be noted that the matrix assumes cost-competitive fossil-free energy will ultimately be available for any of the processes or products. This will also need to include “green” or “pink” hydrogen to avoid carbon emissions from hydrogen production, which is a requirement for the production of many Track 2 products, e.g., sustainable aviation fuels made from CO2. During the transition to fossil-free energy, the potential favorable climate impact might not be fully achieved. These assumptions highlight the need to rigorously apply LCA to guide research, development, and deployment, and to preclude greenwashing (Hepburn et al., 2019; Tanzer and Ramírez, 2019; Naims, 2020; Garcia-Garcia et al., 2021; Committee on Carbon Utilization Infrastructure, 2022; Faber and Sick, 2022; Global CO2 Initiative, 2023; Mertens et al., 2023).
Sources of CO2 that relate to human activity can be grouped into four categories: (1) Emissions from the use of fossil fuels1 (oil, natural gas, coal); (2) process emissions from other (non-fuel) fossil carbon sources (limestone); (3) CO2 that is bio-captured via plants; and (4) CO2 taken from ambient air or water. The former two categories will always lead to additional carbon in the atmosphere unless the CO2 is captured and sequestered in underground formations, or converted into long-lived (Track 1) products. CO2 from the latter two categories can lead to net-negative carbon fluxes via sequestration, or conversion to long-lived products. For underground sequestration, it is assumed that the geological storage security exceeds 10,000 years (Alcalde et al., 2018). It is essential in this context to highlight that the matrix overview of CO2 source and sink combinations will showcase the best possible outcome but that success is not guaranteed unless a detailed lifecycle assessment shows the overall environmental impact (Tanzer and Ramírez, 2019). Here it will be important to conduct a cradle-to-grave assessment unless the product are drop-in replacements for incumbent materials. It is not sufficient to simply investigate how much carbon is bound in a product, but it is critical to analyze any potential carbon emissions at any step of the production process since these could negate the removal function of the product. For commercial products made with CO2, such as inorganic carbonates (CaCO3, MgCO3), as natural rock formations demonstrate, the lifetime could exceed the 10,000-year timeframe that is projected for geological sequestration. For many polymer materials, the lifetime can exceed 1,000s of years equaling the CO2 removal time they can provide (Celina, 2013; Blanco, 2018). The Track 1 categorization of CO2-based materials uses a minimum lifetime of 100 years, consistent with UNFCCC estimations (United Nations Framework Convention on Climate Change, 2023). Short-lived (Track 2) products with lifetimes below 100 years due to decomposition during use or by natural processes will end up as CO2 emissions that can be recaptured and reused as part of a circular carbon economy.
Figure 2 illustrates the relationship between sources and sinks of CO2 for CCUS and the resulting best-case scenario for CO2 removal. Color-coded pathways illustrate the desirability of the various capture-use combinations from the combined perspective of contribution to net-zero greenhouse gas emissions and economic value created independent of taxes and sales of credits.
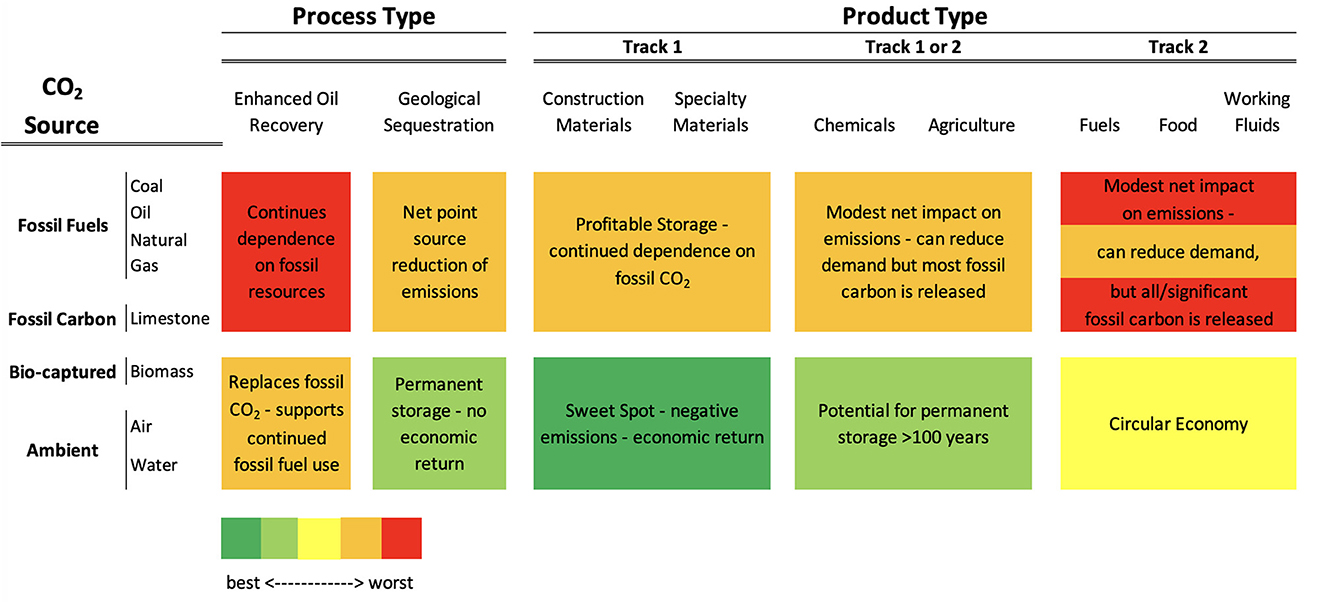
Figure 2. The value or risk of capturing and utilizing or sequestering CO2 strongly depends on combination of source and downstream process or product.2
The impact of CO2 source-sink combinations on atmospheric CO2 levels—which are the ones that matter for global temperatures—can be illustrated by looking at three process and product categories as examples for the use of this source- sink matrix: Geological Sequestration, Construction Materials, and Aviation Fuels.
Geological sequestration
Captured CO2 from direct fossil origin is sequestered, i.e., durably removed by underground storage, underground mineralization, or above-ground mineralization where the resulting material is not used as a commercial product. Since CO2 capture rates are below 100%, and due to some CO2 leakage during handling and transport, the theoretical upper limit for geological sequestration cannot be achieved. The result is a net amount of new CO2 is inevitably added to the atmosphere. In contrast to this, geological sequestration can result in a durable net removal of CO2 when CO2 is captured from biological materials processing (e.g., biomass combustion or corn fermentation to produce ethanol), air or bodies of water. It is required, though, that any process involved in the capture and sequestration effort does not lead to CO2 emissions that are greater than the amount that is being sequestered. Any such emissions would count against the overall carbon balance and reduce the carbon removal rate in practical terms until we have a fully carbon-emission-free energy system. The end result constitutes net-negative carbon dioxide removal (CDR) (Aines et al., 2021). This creates the potential to produce verifiable, meaningful carbon offsets that can be used to increase the economic viability and in the long run should allow financial sustainability without public subsidies.
Construction materials
Mineralization of fossil CO2 via curing of concrete and the production of carbonated aggregates offers opportunities akin to geological sequestration in terms of durability of the storage as well as the near-neutral but not negative carbon balance. As these processes that use fossil CO2 do not create carbon offsets, revenue generation is dependent on tax incentives (e.g., 45Q in the United States), procurement mandates for low-embodied carbon products, and sales of the materials. Using bio-captured or ambient CO2 and converting it to construction materials fundamentally offers the best option for carbon removal. Again, it must be shown in rigorous life cycle assessments, that peripheral CO2 emissions do not counter the removal in the mineralized product. Potential volumes are substantial, e.g., by 2050 over 100 gigatonnes of aggregates will be needed annually (Sick et al., 2022a).
Aviation fuels
Compared to concrete and aggregate production for which CO2 utilization is a new use case for carbon, the production of sustainable aviation fuels (SAF) is not creating a new product rather it replaces a fossil-based incumbent. Although SAF is energy-intensive to produce from CO2, it is necessary because biomass-based SAF cannot meet global demand. In contrast to ground vehicle propulsion, electrification and hydrogen cannot meet technical requirements for long-haul flights in the foreseeable future (Committee on Carbon Utilization Infrastructure, 2022). CO2 from direct fossil origin should not be used in SAF production since it will simply be passed through to the atmosphere with no reduction in atmospheric CO2. In contrast to this, bio-captured and ambient CO2 will lead to a circular fuel economy that can become carbon-neutral within the constraints of potential fossil CO2 emissions, e.g., embodied in the materials that are used to build the production facilities. One could imagine systems where e.g., fossil CO2 and green or pink hydrogen are used to produce methane, a Track 2 material. Capturing CO2 from combustion of that methane to synthesize methane again would add some level of circularity (minus the losses from incomplete CO2 capture).
Discussion
Under the premise of, and with the understanding that carbon-negative processes or products are those that durably remove CO2 from air or bodies of water, a matrix was developed to help understand how the combination of a CO2 source, and the disposition of the CO2 once it is captured, determines a set of outcomes. It is then apparent that the often-used term “carbon capture” alone is not a useful term—the combination of CO2 source type and downstream fate is critically important, and thus must be clearly presented and discussed. Key takeaways are as follows.
1. Fossil and non-fossil sources of CO2 need to be viewed differently. For non-fossil sources the critical consideration is the cost of CO2 delivered to the point of disposition. While cost of capture from fossil point sources is important, from the perspective of climate impact, the critical consideration is the fraction of emitted CO2 that is captured.
2. Track 2 (short-lived) materials are very important due to the dependency of modern society on carbon-based materials. The success of a circular economy based on non-fossil carbon capture may well-depend on its ability to use existing fossil-carbon infrastructure and meet volume needs. For fossil CO2, Track 2 usage simply delays the release of fossil CO2 into the atmosphere and should be avoided.
3. Geologic sequestration is unavoidable but much more work is needed to ensure suitable storage space is available and acceptable.
4. LCA and TEA, standardized and rigorously executed, are essential for all use cases. The carbon credit markets and governmental policy makers need to be absolute in requiring that they be done.
5. Useful and profitable products based on capture of non-fossil CO2 are an obvious contributor to CO2 management, but only if they can be delivered at scale and in the near term.
Figure 2 presents a view of climate relevance and economic opportunities for CO2 source-sink combinations. Similar approaches can be taken to highlight the respective cost, dependence on subsidies, potential revenue, CO2 removal or CO2 utilization potentials, and more.
Data availability statement
Publicly available datasets were analyzed in this study. This data can be found here: https://dx.doi.org/10.7302/5825.
Author contributions
FM: Conceptualization, Writing—original draft, Writing—review and editing. GS: Writing—original draft, Writing—review and editing. SF: Writing—original draft, Writing—review and editing. VS: Writing—original draft, Writing—review and editing.
Funding
The author(s) declare financial support was received for the research, authorship, and/or publication of this article. This work was funded by the Global CO2 Initiative at the University of Michigan. The authors also acknowledge funding from the BlueSky program of the College of Engineering at the University of Michigan.
Conflict of interest
The authors declare that the research was conducted in the absence of any commercial or financial relationships that could be construed as a potential conflict of interest.
The author(s) declared that they were an editorial board member of Frontiers, at the time of submission. This had no impact on the peer review process and the final decision.
Publisher's note
All claims expressed in this article are solely those of the authors and do not necessarily represent those of their affiliated organizations, or those of the publisher, the editors and the reviewers. Any product that may be evaluated in this article, or claim that may be made by its manufacturer, is not guaranteed or endorsed by the publisher.
Footnotes
1. ^It is noted that fossil fuels category includes their use as the raw materials, e.g., for chemicals and polymer production.
2. ^Note that no CO2 capture process captures 100% of the emissions and therefore the implications shown in Figure 2 are upper theoretical limits.
References
Aines, R. D., Amador, G., Anderegg, W., Belmont, W., Bergman, A., Buck, H. J., et al. (2021). “Carbon dioxide removal primer,” in eds J. Wilcox, B. Kolosz, and J. Freeman. Available online at: www.cdrprimer.org
Alcalde, J., Flude, S., Wilkinson, M., Johnson, G., Edlmann, K., Bond, C. E., et al. (2018). Estimating geological CO2 storage security to deliver on climate mitigation. Nat. Commun. (2018) 9, 2201. doi: 10.1038/s41467-018-04423-1
Author Collective (2022). Climate Change 2022: Mitigation of Climate Change. Working Group III Contribution to the IPCC Sixth Assessment Report. Intergovernmental Panel on Climate Change Working Group III. Available online at: https://www.ipcc.ch/report/sixth-assessment-report-working-group-3/
Blanco, I. (2018). Lifetime prediction of polymers: to bet, or not to bet-is this the question? Materials 11, 1383. doi: 10.3390/ma11081383
Celina, M. C. (2013). Review of polymer oxidation and its relationship with materials performance and lifetime prediction. Polym. Degrad. Stabil. 98, 2419–2429. doi: 10.1016/j.polymdegradstab.2013.06.024
Committee on Carbon Utilization Infrastructure, Markets, Research and Development, Board on Energy and Environmental Systems, Board on Chemical Sciences and Technology, Division on Engineering and Physical Sciences, Division on Earth and Life Studies, National Academies of Sciences, Engineering, and Medicine. (2022). Carbon Dioxide Utilization Markets and Infrastructure: Status and Opportunities: A First Report. Washington, DC: National Academies Press, 26703.
Faber, G., and Sick, V. (2022). Identifying and Mitigating Greenwashing of Carbon Utilization Products. Ann Arbor, MI: University of Michigan.
Garcia-Garcia, G., Fernandez, M. C., Armstrong, K., Woolass, S., and Styring, P. (2021). Analytical review of life-cycle environmental impacts of carbon capture and utilization technologies. ChemSusChem 14, 995–1015. doi: 10.1002/cssc.202002126
Global CO2 Initiative (2023). AssessCCUS: Techno-Economic and Life Cycle Assessment for Carbon Capture, Utilization, and Storage. Available online at: https://assessccus.globalco2initiative.org/ (accessed August 28, 2023).
Harvey, F. (2023a). Carbon Capture and Storage Is “No Free Lunch”, Warns Climate Chief . The Guardian. Available online at: https://www.theguardian.com/environment/2023/jun/06/carbon-capture-and-storage-is-no-free-lunch-warns-climate-chief-hoesung-lee (accessed June 9, 2023).
Harvey, F. (2023b). Carbon Dioxide Removal: The Tech That Is Polarising Climate Science. The Guardian. Available online at: https://www.theguardian.com/environment/2023/apr/25/carbon-dioxide-removal-tech-polarising-climate-science (accessed May 12, 2023).
Hepburn, C., Adlen, E., Beddington, J., Carter, E. A., Fuss, S., Mac Dowell, N., et al. (2019). The technological and economic prospects for CO2 utilization and removal. Nature 575, 87–97. doi: 10.1038/s41586-019-1681-6
International Energy Agency (2020). CCUS in Clean Energy Transitions., Energy Technology Perspectives. Paris.
Lutzke, L., and Árvai, J. (2021). Consumer acceptance of products from carbon capture and utilization. Clim. Change 166, 15. doi: 10.1007/s10584-021-03110-3
Masson-Delmotte, C., Zhai, P., Pörtner, H. -O., Roberts, D., Skea, J., Shukla, P. R., et al. (eds.). (2018). Global Warming of 1.5°C. An IPCC Special Report on the Impacts of Global Warming of 1.5°C above Pre-Industrial Levels and Related Global Greenhouse Gas Emission Pathways, in the Context of Strengthening the Global Response to the Threat of Climate Change, Sustainable Development, and Efforts to Eradicate Poverty. Geneva: Intergovernmental Panel on Climate Change.
Meessen, J. (2014). Urea synthesis. Chem. Ingenieur Tech. 86, 2180–2189. doi: 10.1002/cite.201400064
Mertens, J., Breyer, C., Arning, K., Bardow, A., Belmans, R., Dibenedetto, A., et al. (2023). Carbon capture and utilization: more than hiding CO2 for some time. Joule 7, 442–449. doi: 10.1016/j.joule.2023.01.005
Mishra, A., Ntihuga, J. N., Molitor, B., and Angenent, L. T. (2002). Power-to-protein: carbon fixation with renewable electric power to feed the world. Joule 4, 1142–1147. doi: 10.1016/j.joule.2020.04.008
Naims, H. (2020). Economic aspirations connected to innovations in carbon capture and utilization value chains. J. Ind. Ecol. 24, 1126–1139. doi: 10.1111/jiec.13003
National Academies of Sciences and Engineering (2022). A Research Strategy for Ocean-Based Carbon Dioxide Removal and Sequestration. Washington, DC.
National Energy Technology Laboratory (2022). Carbon Dioxide Utilization Life Cycle Analysis Guidance Toolkit. Available online at: https://www.netl.doe.gov/LCA/CO2U
Newman, A. J. K., Dowson, G. R. M., Platt, E. G., Handford-Styring, H. J., and Styring, P. (2023). Custodians of carbon: creating a circular carbon economy. Front. Energy Res. 11, 1124072. doi: 10.3389/fenrg.2023.1124072
Pander, B., Mortimer, Z., Woods, C., McGregor, C., Dempster, A., Thomas, L., et al. (2002). Hydrogen oxidising bacteria for production of single-cell protein and other food and feed ingredients. Eng. Biol. 4, 21–24. doi: 10.1049/enb.2020.0005
Ramanathan, V., Aines, R., Auffhammer, M., Barth, M., Cole, J., Forman, F., et al (2021). “Bending the curve: climate change solutions,” ed Ramanathan, V., Series Edn. (Regents of the Univ of California). Available online at: https://escholarship.org/uc/item/6kr8p5rq
Rao, A. B., and Rubin, E. S. (2002). A technical, economic, and environmental assessment of amine-based CO2 capture technology for power plant greenhouse gas control. Environ. Sci. Technol. 36, 4467–4475. doi: 10.1021/es0158861
Ruuskanen, V., Givirovskiy, G., Elfving, J., Kokkonen, P., Karvinen, A., Järvinen, L., et al. (2021). Neo-carbon food concept: a pilot-scale hybrid biological–inorganic system with direct air capture of carbon dioxide. J. Clean. Prod. 278, 123423. doi: 10.1016/j.jclepro.2020.123423
Sick, V. (2021). Spiers memorial lecture: co2 utilization: why, why now, and how? Faraday Discuss. 230, 9–29. doi: 10.1039/D1FD00029B
Sick, V., Armstrong, K., and Moni, S. (2023). Editorial: harmonizing life cycle analysis (LCA) and techno-economic analysis (TEA) guidelines: a common framework for consistent conduct and transparent reporting of carbon dioxide removal and CCU technology appraisal. Front. Clim. 5, 1204840. doi: 10.3389/fclim.2023.1204840
Sick, V., Stokes, G., and Mason, F. (2022a). Implementing CO2 Capture and Utilization at Scale and Speed: The Path to Achieving Its Potential. Ann Arbor, MI: Global CO2 Initiative.
Sick, V., Stokes, G., and Mason, F. C. (2022b). CO2 utilization and market size projection for CO2-treated construction materials. Front. Clim. 4, 878756. doi: 10.3389/fclim.2022.878756
Sillman, J., Nygren, L., Kahiluoto, H., Ruuskanen, V., Tamminen, A., Bajamundi, C., et al. (2019). Bacterial protein for food and feed generated via renewable energy and direct air capture of CO2: can it reduce land and water use? Glob. Food Sec. 22, 25–32. doi: 10.1016/j.gfs.2019.09.007
Tanzer, S. E., and Ramírez, A. (2019). When are negative emissions negative emissions? Energy Environ. Sci. 12, 1210–1218. doi: 10.1039/C8EE03338B
United Nations Framework Convention on Climate Change (2023). Common Metrics. UNFCCC. Available online at: https://unfccc.int/process-and-meetings/transparency-and-reporting/methods-for-climate-change-transparency/common-metrics (accessed August 28, 2023).
Wilcox, J., Psarras, P. C., and Liguori, S. (2017). Assessment of reasonable opportunities for direct air capture. Environ. Res. Lett. 12, 065001. doi: 10.1088/1748-9326/aa6de5
Keywords: CO2 sources, CO2 sinks, CCUS, CCU, LCA, TEA, CO2 sequestration, CO2 conversion
Citation: Mason F, Stokes G, Fancy S and Sick V (2023) Implications of the downstream handling of captured CO2. Front. Clim. 5:1286588. doi: 10.3389/fclim.2023.1286588
Received: 31 August 2023; Accepted: 13 September 2023;
Published: 29 September 2023.
Edited by:
Ben W. Kolosz, University of Hull, United KingdomReviewed by:
Jani Sillman, Lappeenranta University of Technology, FinlandCopyright © 2023 Mason, Stokes, Fancy and Sick. This is an open-access article distributed under the terms of the Creative Commons Attribution License (CC BY). The use, distribution or reproduction in other forums is permitted, provided the original author(s) and the copyright owner(s) are credited and that the original publication in this journal is cited, in accordance with accepted academic practice. No use, distribution or reproduction is permitted which does not comply with these terms.
*Correspondence: Volker Sick, dnNpY2smI3gwMDA0MDt1bWljaC5lZHU=