- 1Sorbonne University, CNRS, Laboratoire d'Océanographie de Villefranche, Villefranche-sur-Mer, France
- 2Institute for Sustainable Development and International Relations, Sciences Po, Paris, France
- 3Monaco Association on Ocean Acidification, Prince Albert II of Monaco Foundation, Monaco, Monaco
- 4School of Environmental Sciences, University of East Anglia, Norwich, United Kingdom
- 5King Abdullah University of Science and Technology (KAUST), Red Sea Research Center (RSRC), Thuwal, Saudi Arabia
- 6UMR LIENSs 7266, Université de La Rochelle-CNRS, La Rochelle, France
The effectiveness, feasibility, duration of effects, co-benefits, disbenefits, cost effectiveness and governability of four ocean-based negative emissions technologies (NETs) are assessed in comparison to eight other ocean-based measures. Their role in revising UNFCCC Parties' future Nationally Determined Contributions is discussed in the broad context of ocean-based actions for both mitigation and ecological adaptation. All measures are clustered in three policy-relevant categories (Decisive, Low Regret, Concept Stage). None of the ocean-based NETs assessed are identified as Decisive at this stage. One is Low Regret (Restoring and increasing coastal vegetation), and three are at Concept Stage, one with low to moderate potential disbenefits (Marine bioenergy with carbon capture and storage) and two with potentially high disbenefits (Enhancing open-ocean productivity and Enhancing weathering and alkalinization). Ocean-based NETs are uncertain but potentially highly effective. They have high priority for research and development.
1. Introduction
Implementing the Paris Agreement is a formidable challenge. In pathways limiting global warming to 1.5°C with no or limited overshoot as well as in pathways with a higher overshoot, CO2 emissions are reduced to net zero globally around 2050 (IPCC, 2018). This would require far-reaching and unprecedented transitions in all sectors, and also large-scale use of negative emissions technologies (NETs), that is removal of greenhouse gases from the atmosphere by deliberate human activities.Yet the trade-offs for food production and nature conservation limit carbon dioxide removal by most land-based approaches (Boysen et al., 2017).
In that context, it is timely to assess the opportunities offered by the ocean to reduce the causes and also the consequences of climate change, globally and locally. Recent papers and reports have reviewed several potential measures (Gattuso et al., 2018; National Academies of Sciences Engineering and Medicine, 2018; Because the Ocean, 2019; GESAMP, 2019; Hoegh-Guldberg et al., 2019). Countries have so far only made limited use of ocean-related measures for tackling climate change and its impacts through their Nationally Determined Contributions (NDC) under the Paris Agreement (Gallo et al., 2017). Among the 161 initial NDCs submitted in 2014–2015, 70% mentioned marine issues, most frequently as components of adaptation action or in regard to climate impacts. Just over a third (59) also included ocean-related mitigation measures. The only ocean-based negative emission approach specifically mentioned is the conservation and restoration of coastal blue carbon ecosystems (i.e., mangroves, saltmarshes, and seagrasses). The 5-year revisions of NDCs that need to be made before the re-scheduled COP26 in 2021 (26th Conference of the Parties of the United Nations Framework Convention on Climate Change, UNFCCC), offer an opportunity to adopt more ocean-inclusive mitigation and adaptation strategies.
Here we assess four ocean-based NETs involving carbon dioxide removal (Marine bioenergy with carbon capture and storage, Restoring and increasing coastal vegetation, Enhancing open-ocean productivity, Enhancing weathering and alkalinization) and put them in the broader context of ocean-based measures to support climate policies, especially greenhouse gas mitigation and ecological adaptation. These carbon dioxide removal pathways are the most documented in the literature. Other pathways are not evaluated here and it is likely that new methods or hybrids between the methods presented here will emerge. Building on recently published material (Gattuso et al., 2018), this Policy Brief brings two innovations. It assesses marine-biomass-fueled bioenergy with carbon capture and storage (Marine BECCS) and goes a step further by grouping these NETs and a wide range of other options into policy clusters with the aim of guiding decisions on priority areas of action.
2. Ocean Negative Emissions Technologies
Gattuso et al. (2018) published a comprehensive expert assessment of ocean-based measures to reduce climate change and its impacts based on a review of 862 publications and expert judgment. Here we focus on NETs and consider eight criteria: (1) effectiveness to increase net carbon uptake, (2) effectiveness to reduce ocean warming, ocean acidification, and sea level rise; (3) feasibility, covering both technological readiness and lead time until full potential effectiveness; (4) duration of effects; (5) cost effectiveness; (6) co-benefits; (7) disbenefits and (8) governability from an international perspective. Figure 1 summarizes the assessment. Full information is available in the Supplementary Material of Gattuso et al. (2018) and in Supplementary Material (SM3).
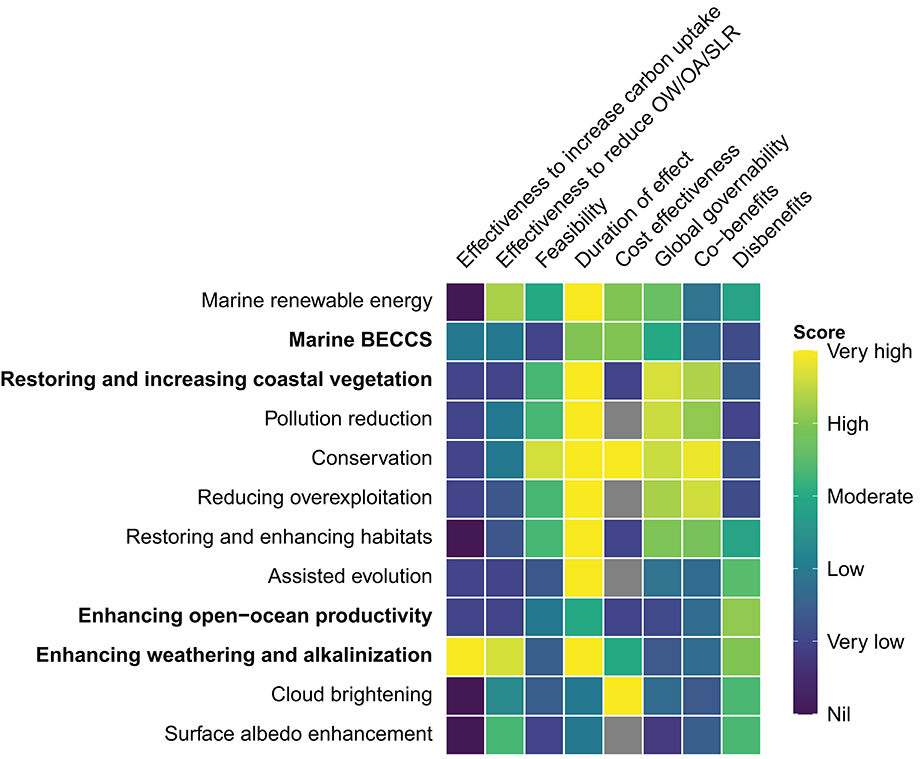
Figure 1. Assessment of ocean negative emissions approaches (in bold) compared with other ocean-based measures. Based on Gattuso et al. (2018), except for marine BECCS (see SM3 in Supplementary Material), with the following changes: Vegetation and Alkalinization are only considered at global scale except the co-benefits and disbenefits which are considered at local scale. Two criteria are newly defined by combining criteria assessed earlier. Feasibility is the mean of Technological readiness and Lead time until full potential effectiveness. Effectiveness to reduce OW/OA/SLR (ocean warming, ocean acidification and sea level rise) is the mean of the effectiveness to Moderate warming, Moderate acidification and Moderate sea level rise. Gray tiles indicate criteria not assessed for the corresponding measure. Further information on the measures and criteria are available in Supplementary Material (SM1 and SM2).
2.1. Marine Bioenergy With Carbon Capture and Storage
Considering the area, water and nutrient requirements leads to the tentative conclusion that macroalgae (seaweed) based BECCS could be a better long-term option than woody biomass energy crops, because achievable productivity is higher, freshwater demands are eliminated, and nutrient demands may be lower if efficient recycling systems can be developed (Lenton, 2014). Here we do not consider hybrid bioenergy with carbon capture and storage approaches to focus on marine-biomass-fueled BECCS. The latter is expected to involve three overarching steps: increasing carbon uptake by macroalgal aquaculture; converting the biomass to biofuel; and deploying carbon capture (at power stations) and long-term geological storage. Capron et al. (2020) review recent progress by US researchers in using macroalgae for biofuel.
The effectiveness of Marine BECCS to increase carbon uptake is scored high. Its effectiveness to moderate ocean climate drivers is also scored high given the close relationship between CO2 and ocean warming, acidification and sea level rise.
However, feasibility is considered low because components of this approach have not all been demonstrated at scale and may require a decade or more of research and development to prove capacity, cost effectiveness and safety. Cost effectiveness (based on a single estimate) is high, at 26 US$ per t CO2 removed for marine biomass fueled BECCS.
Climate-relevant alternatives to using macroalgae for BECCS would be to develop methods for deep water disposal of the additional biomass generated through macroalgal aquaculture (Williamson et al., 2021) and the manufacturing of long-lived products, such as plastic, that displace products generating emissions, thereby avoiding emissions, or enhancing soil fertility while contributing to carbon sequestration through use as biochar.
2.2. Restoring and Increasing Coastal Vegetation
Restoring and increasing coastal vegetation (“blue carbon” ecosystems) aims at enhancing CO2 uptake and avoiding further emissions. Here we look at the implementation of this measure at the global scale, by restoring a high proportion of the human-degraded salt marsh, mangrove and seagrass habitats of the planet.
Conservation and restoration of blue carbon ecosystems support and enhance CO2 sequestration, whilst also reducing emissions associated with habitat degradation and loss (McLeod et al., 2011; Pendleton et al., 2012; Duarte et al., 2013; Marbà et al., 2015; Howard et al., 2017; Hamilton and Friess, 2018). The theoretical effectiveness of such measures at the global scale is limited by the maximum area that can be occupied by these habitats, with their maximum global carbon burial estimated to be 234 Tg C per year for salt marshes, mangroves, and seagrasses combined (McLeod et al., 2011). Assuming that historic losses of blue carbon ecosystems (50% for mangroves since the 1940s, 29% for seagrass since 1879, 25% for salt marshes since the 1800s; Waycott et al., 2009; McLeod et al., 2011) are rapidly reversed through restoration, the maximum cumulative carbon burial would be 26 Pg C until 2100, equivalent to about 2.5 years of current anthropogenic emissions. This is a theoretical upper estimate because of likely constraints on implementation. The effectiveness to increase carbon uptake is therefore scored very low, noting also that increased methane (CH4) emissions may fully or partly negate the climatic benefits of CO2 removal (Al-Haj and Fulweiler, 2020; Rosentreter and Williamson, 2020). Carbonate formation or dissolution in blue carbon habitats will also affect the capacity of these habitats to remove atmospheric carbon; although dissolution may dominate, thereby increasing removal (Saderne et al., 2019), these processes have not yet been explored sufficiently to provide global estimates.
Like carbon uptake, the effectiveness to moderate warming, acidification and sea level rise is limited by the maximum area possibly occupied by these habitats, hence it is scored very low. Feasibility is high as blue carbon approaches are well tested and deployed around the world (Howard et al., 2014) but the full delivery of the benefits at their maximum global capacity will require years to decades to be achieved. Protection of blue carbon habitats must be multi-decadal in order to be effective, and successful restoration also requires a long term commitment (e.g., Duarte et al., 2013; Marbà et al., 2015; Reynolds et al., 2016)—with the assumption that future temperature increases can be kept below 2°C (under higher warming, blue carbon ecosystems are at risk, and their stored carbon is likely to be lost; Bindoff et al., 2019). The duration of the effect is scored permanent, with those provisos. At median costs of 240, 30,000, and 7,800 US$ per t CO2, respectively for mangroves, salt marsh and seagrass habitats, the cost effectiveness (for climate mitigation) is collectively scored very low (Siikamaki et al., 2012; Bayraktarov et al., 2016; Narayan et al., 2016), recognizing that there are large differences in restoration costs between the three ecosystems (and at the local level), and that the cost effectiveness of restoration for the delivery of other ecosystem services could nevertheless be very high.
2.3. Enhancing Open-Ocean Productivity
Enhancing open-ocean productivity (hereafter Fertilization) is based on nutrients addition directly or indirectly to increase CO2 drawdown by phytoplankton. Such methods may appear technologically feasible, as it has been shown for iron/macronutrient addition, as well as for artificial upwellings (Pan et al., 2016).
For iron addition, modeling studies show a maximum effect on atmospheric CO2 of 15–45 ppmv in 2100 (Zeebe and Archer, 2005; Aumont and Bopp, 2006; Keller et al., 2014). Ocean fertilization with macro-nutrients (N, P) has a higher theoretical potential of 1.5 PgC yr-1 (Harrison, 2017), about 50 times more than the maximum simulated effects of Southern Ocean iron fertilization (Oschlies et al., 2010); however, very large quantities of macronutrients are needed and the proposed scaling of this technique seems unrealistic (Williamson and Bodle, 2016). For artificial upwelling (upward pumping of nutrient-rich deep waters), the intended carbon removal by increased productivity (Lovelock and Rapley, 2007) may be matched by the undesirable release of CO2 from the deeper water (Shepherd et al., 2007; Dutreuil et al., 2009; Yool et al., 2009). The potential impact of artificial upwellings on nitrogen fixation, and hence on natural carbon sequestration is controversial (Fennel, 2008; Karl and Letelier, 2008). Some modeling studies indicate that net CO2 drawdown is theoretically possible if upwelling rates are increased in appropriate locations in 2100 for RCP8.5. Because of the many associated uncertainties, the overall effectiveness of Fertilization to increase carbon uptake is scored low.
Fertilization has no direct effect on sea level rise nor on ocean warming hence its effectiveness to reduce those drivers is low. Acidification could be ameliorated in the short term in the upper ocean (by CO2 removal), but enhanced in the long term in the ocean interior (by CO2 release); the overall effectiveness is considered to be low. Effects would happen through increased carbon uptake but there is limited scope for enhanced ocean productivity and increased carbon uptake due to biological and physical-chemical constraints (Williamson and Bodle, 2016). Mesoscale variability in the strength of different processes responsible for long-term carbon removal (Boyd and Vivian, 2019) make carbon accounting highly uncertain. Furthermore, once Fertilization is started, it would need to be done continuously; if not, a large part of the sequestered carbon will be returned to the atmosphere on decadal timescales (e.g., Aumont and Bopp, 2006). Ocean Fertilization is considered to have negative consequences for 8 Sustainable Development Goals (SDGs), and a combination of both positive and negative consequences for 7 SDGs (Honegger et al., 2020). The cost of iron fertilization has been estimated at 22–119 and 457 US$ per t CO2 sequestered (Harrison, 2013). There are lower cost estimates for Fertilization using macronutrients, at around US$ 20 per t CO2 (Jones, 2014), but scalability is questionable and monitoring costs are excluded. The median of all estimates is 230 US$ per t CO2, indicating very low cost effectiveness.
2.4. Enhancing Weathering and Alkalinization
Enhancing weathering and alkalinization (hereafter Alkalinization) is the addition of natural or man-made alkalinity (ground carbonate or silicate rocks, such as olivine or basalt), to enhance CO2 removal and/or carbon storage. Adding huge amounts of alkalinity globally could, in theory, substantially mitigate atmospheric CO2 without elevating biogeochemical properties significantly beyond naturally occurring levels (Ilyina et al., 2013; Keller et al., 2014).
Since the carbon uptake capacity of this approach is very high, without a determined limit (Ciais et al., 2013), its effectiveness to reduce ocean warming and acidification is also very high, with impacts on sea level via surface air temperature (Gonzalez and Ilyina, 2016). Regional Alkalinization could be effective in protecting coral reefs against acidification (but not warming; Feng et al., 2016). There is technological readiness to add alkalinity to the ocean, at least at small scales. What is needed is research and testing to acquire adequate knowledge about the environmental co-benefits and disbenefits. Feasibility is also impeded by lack of infrastructure to mine or produce, process and distribute alkalinity at large scales. Feasibility is therefore scored very low.
A unit of alkalinity added would sequester CO2 essentially permanently, hence the duration of the effect is scored very high. Some of the inorganic carbon could precipitate as CaCO3, releasing CO2 and reducing the carbon storage and alkalinity co-benefits afforded. The addition of alkalinity would probably have to be continued for decades to centuries (or longer) to have a substantial impact on atmospheric CO2 (Keller et al., 2014).
The cost of various ocean alkalinity carbon storage technologies is largely speculative at this stage. Renforth et al. (2013) indicated a range of 72–159 US$ per t CO2 taken up. This range reflects the extraction, calcination, hydration, and surface ocean dispersion costs at a global scale (including transportation). In the case of direct addition of alkaline minerals to the ocean (i.e., without calcination), the cost is estimated to be 20–50 US$ per t CO2 (Harvey, 2008; Köhler et al., 2013; Renforth and Henderson, 2017). Overall, at 10–190 US$ per t CO2, the cost effectiveness is moderate.
3. Policy-Relevance of Negative Emission Technologies
Understanding the policy relevance of NETs requires putting them into a broader context of global and/or local climate action. Here we consider 12 ocean-related measures to enhance both global mitigation and local coastal ecological adaptation1 (Figure 2). These options can be clustered into three overarching policy-relevant clusters (Decisive, Low Regret, Concept Stage) that are defined in Figure 2A according to their state of implementation, effectiveness to reduce climate-related ocean drivers globally, effectiveness to reduce impacts/risks locally, and potential co-benefits and disbenefits (i.e., associated adverse impacts and other undesirable consequences, including opportunity costs).
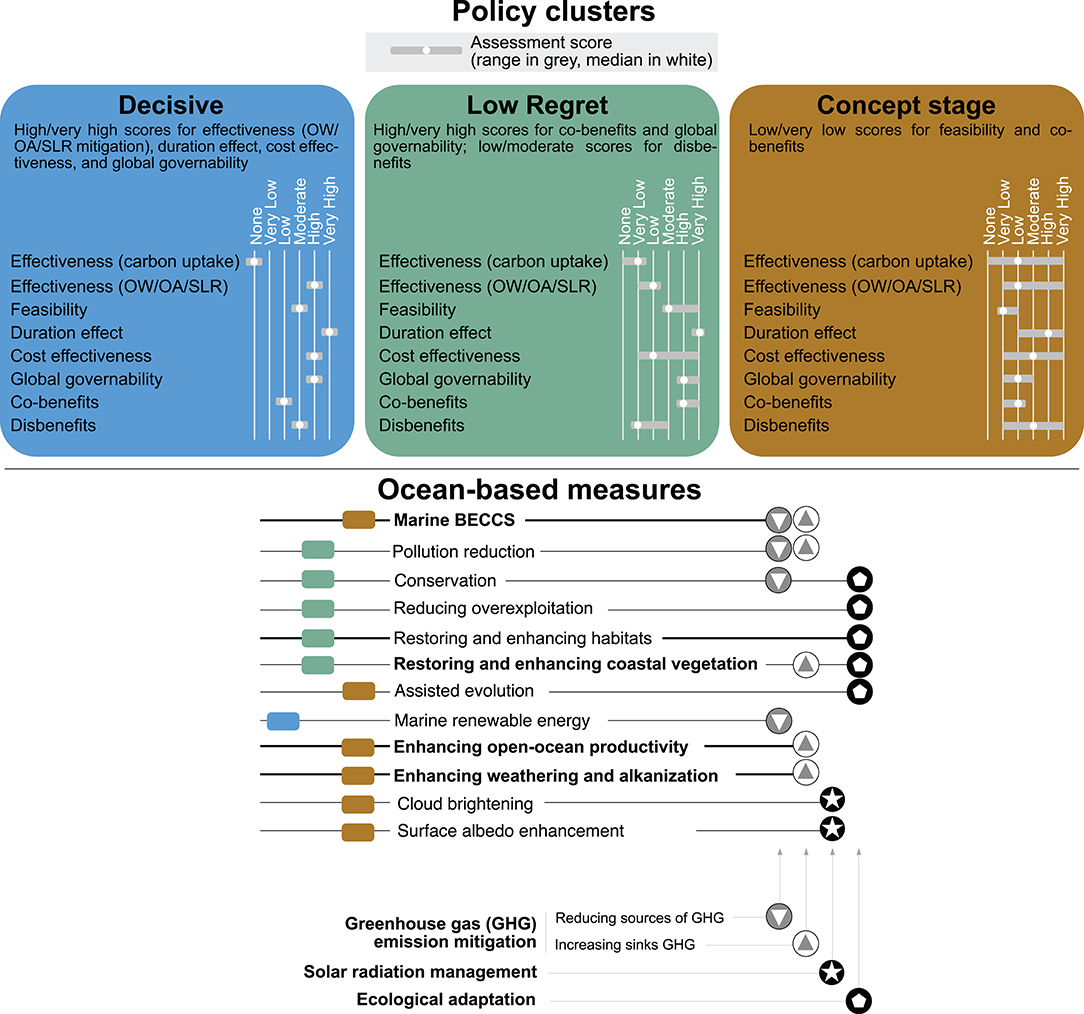
Figure 2. Policy clusters of ocean-based climate action. The measures in bold are the four NETs given detailed attention in this paper, others are modified from Gattuso et al. (2018).
3.1. Cluster 1
The only ocean-based Decisive measure that we have identified addresses the causes of climate change: Marine renewable energy. It includes energy from offshore winds, tides, waves, ocean currents as well as thermal gradients. This action has the theoretical potential to meet all global electricity requirements, although requiring massive infrastructure development. Whilst some local adverse impacts are inevitable, these can be minimized.
3.2. Cluster 2
Low Regret measures provide both climatic and non-climatic benefits, with few disbenefits. For example, Conservation measures can protect carbon-rich coastal ecosystems from direct human disturbance and loss, and play an important role in limiting local climate impacts. Similarly, Restoring and enhancing coastal vegetation supports ecological adaptation whilst providing storm protection, contributing to food security, and enhancing biodiversity. It can also increase carbon uptake (i.e., addressing the causes of climate change), at levels that may be locally and/or nationally significant. Nevertheless, because of the limited total area for restoring such blue carbon ecosystems and also associated methane emissions, this action can only make a very small contribution to climate mitigation at the global scale (IPCC, 2019; Al-Haj and Fulweiler, 2020). Pollution reduction in coastal waters removes contaminants and excess nutrients that impair ecosystem function, thereby supporting ecosystem-based adaptation. Reducing atmospheric pollution from shipping can also, to a limited degree, address the causes of climate change. However, Low Regret measures are not cost-free, requiring well-informed planning and effective coordination over a wide range of spatial and temporal scales.
3.3. Cluster 3
Concept Stage measures are illustrated by the NET Marine BECCS, and some forms of Assisted evolution (alterations to species and genetics). The former would use cultivated macroalgae (or possibly microalgae) as the biomass source for bioenergy. Such measures have potential but their feasibility and cost-effectiveness for climatic benefits have yet to be demonstrated. Several measures have potentially high disbenefits, as identified for Fertilization, Alkalinization and the sunlight reflection techniques of marine Cloud brightening and Surface albedo enhancement (e.g., covering the ocean surface with reflective foam). Whilst all these approaches have a very large theoretical potential to address climate change globally, only Fertilization has been investigated through field experiments (although not carried out for climate mitigation purposes), with limited success. Much more attention needs to be given to their governance and public acceptability before they can be considered for implementation as climate policy responses. This agrees with and extends the conclusion of Boyd and Vivian (2019) for marine geoengineering.
The effectiveness of measures such as Assisted evolution to support ecological adaptation critically depends on the environmental and societal contexts of their implementation. For example, while some Assisted evolution tools could have local benefits, others such as the spread of genetically alien populations have serious risks.
3.4. Global Governability
The global governability of NETs refers in this study to the potential capability of the international community (both nation states and international non-state actors) to implement them, managing associated conflicts and harnessing mutual benefits, It is therefore a key dimension to be considered when discussing the potential role of NETs within a broader range of climate actions. In our assessment, global governability is considered moderate to very high for more than half of the measures considered (Figure 1), but low to very low for Fertilization and Alkalinization as well as for Assisted evolution and the two SRM options (Cloud brightening, Surface Albedo enhancement). Marine BECCS score is moderate: although this measure could have high co-benefits and could be implemented at modest scale without having to rely on international cooperation, wider scale implementation could raise serious national and international complications, hence potentially requiring new governing arrangements and accounting, monitoring, and verification entities. Alkalinization and Fertilization score very low, but for different reasons: concerns regarding their potential adverse environmental impacts result in low public acceptability, reflected in political rejection of such methods (and legal constraints) by the London Convention/London Protocol2, the UN Convention on Biological Diversity, and other bodies. These findings align with recent conclusions that “marine geoengineering” challenges the ability of the international law system, as it stands now, to govern the implementation of these ideas (McGee et al., 2018).
4. Conclusion and Actionable Recommendations
Other than by eliminating nearly all anthropogenic greenhouse gas emissions, there is no single measure to dramatically accelerate progress in achieving the net zero target of the Paris Agreement. Nevertheless, opportunities exist for all coastal countries to implement a wide range of ocean-based climate actions, including negative emission approaches. Guidelines and actionable recommendations are required to address the urgency of climate action. Marine geoengineering techniques (e.g., Boyd and Vivian, 2019) and other ocean-based measures need to be assessed and ranked (e.g., Gattuso et al., 2018). Here we build on previous assessments to provide a first attempt to define policy-relevant clusters for climate action, both global mitigation and local ecological adaptation.
None of the four ocean-based NETs assessed are Decisive, one is Low Regret (Restoring and increasing coastal vegetation), and three are at Concept Stage with either low to moderate potential disbenefits (Marine bioenergy with carbon capture and storage) or with high potential disbenefits (Enhancing open-ocean productivity and Enhancing weathering and alkalinization). The next iteration of more ambitious NDCs should scale up ocean-based climate action by prioritizing the implementation of Decisive and Low Regret measures, and urgently improving knowledge on Concept Stage measures. Decisive and Low Regret measures are both key priorities for implementation because, on the one hand, the full implementation of Decisive measures will not completely eliminate coastal risks while, on the other hand, the effectiveness and security of Low Regret conservation/nature-based solutions are being put at risk by increasing climate change and rising sea level. Concept Stage measures require further scientific investigations because the full implementation of proven measures runs the risk of falling short of providing enough cost effective NETs capacity.
Balancing ocean-based measures also calls for securing robust national-to-local enabling conditions and enhanced international support for climate action. This is important because the majority of ocean-inclusive NDCs are conditional on external financing and support. They were discussed at COP25, referred to the “Blue COP,” and could be, with nature-based solutions, one of the priority concerns for COP26 and the subsequent Global Stocktake in 2023. Enhancing the enabling conditions can be achieved by (i) strengthening the formal recognition of the ocean-climate nexus and the ocean as a provider of solutions for climate change, and (ii) facilitating the UNFCCC Party delegations' understanding of the role of ocean-related measures and how to include them in the next generation of NDCs.
Author Contributions
All authors contributed the ideas. J-PG wrote the first draft which was then finalized by all authors.
Funding
The Ocean Solutions Initiative was financially supported by the Prince Albert II of Monaco Foundation, the Veolia Foundation, the IAEA Ocean Acidification International Coordination Centre, the French Facility for Global Environment, and the STORISK project (French National Research Agency, ANR-15-CE03-0003). The ANR programme Investissements d'avenir (ANR-10-LABX-14-01) provides support to Iddri.
Conflict of Interest
The authors declare that the research was conducted in the absence of any commercial or financial relationships that could be construed as a potential conflict of interest.
The reviewer CB declared a past co-authorship with one of the authors CD.
Acknowledgments
Thanks are due to the coauthors of the papers by Gattuso et al. (2018) and Gattuso et al. (2019). This work is a product of The Ocean Solutions Initiative (http://bit.ly/2xJ3EV6) and the STORISK project (https://bit.ly/2YMhfZX). Comments from L. Kapsenberg and three reviewers have significantly improved an earlier version of this paper.
Supplementary Material
The Supplementary Material for this article can be found online at: https://www.frontiersin.org/articles/10.3389/fclim.2020.575716/full#supplementary-material
Footnotes
1. ^Societal adaptation measures such as infrastructure-based or community-based adaptation, risk reduction policies improvement, and the relocation of people, assets and economic activities are not included.
2. ^As regulated by the London Protocol, with an amendment prohibiting such action unless constituting legitimate scientific research authorized under permit. That amendment has not yet legally entered force.
References
Al-Haj, A., and Fulweiler, R. (2020). A synthesis of methane emissions from shallow vegetated coastal ecosystems. Glob. Change Biol. 26, 2988–3005. doi: 10.1111/gcb.15046
Aumont, O., and Bopp, L. (2006). Globalizing results from ocean in situ iron fertilization studies. Glob. Biogeochem. Cycles 20:GB2017. doi: 10.1029/2005GB002591
Bayraktarov, E., Saunders, M., Abdullah, S., Mills, M., Beher, J., Possingham, H., et al. (2016). The cost and feasibility of marine coastal restoration. Ecol. Appl. 26, 1055–1074. doi: 10.1890/15-1077
Because the Ocean (2019). Ocean for Climate. Because the Ocean. Available online at: https://www.becausetheocean.org/wp-content/uploads/2019/10/Ocean_for_Climate_Because_the_Ocean.pdf
Bindoff, N., Cheung, W., Kairo, J., Aristegui, J., Guinder, V., Hallberg, R., et al. (2019). “Changing ocean, marine ecosystems, and dependent communities,” in Special Report on Ocean and Cryosphere in a Changing Climate, H. O. Portner, D. Roberts, V. Masson-Delmotte, and P. Zhai (Geneva: Intergovernmental Panel on Climate Change), 447–587.
Boyd, P., and Vivian, C. (2019). Should we fertilize oceans or seed clouds? no one knows. Nature 570, 155–157. doi: 10.1038/d41586-019-01790-7
Boysen, L., Lucht, W., and Gerten, D. (2017). Trade-offs for food production, nature conservation and climate limit the terrestrial carbon dioxide removal potential. Glob. Change Biol. 23, 4303–4317. doi: 10.1111/gcb.13745
Capron, M. E., Stewart, J. R., de Ramon N'Yeurt, A., Chambers, M. D., Kim, J. K., Yarish, C., et al. (2020). Restoring pre-industrial CO2 levels while achieving sustainable development goals. Energies 13:4972. doi: 10.3390/en13184972
Ciais, P., Sabine, C., Bala, G., Bopp, L., Brovkin, V., Canadell, J., et al. (2013). “Carbon and other biogeochemical cycles,” in Climate Change 2013: The Physical Science Basis. Contribution of Working Group I to the Fifth Assessment Report of the Intergovernmental Panel on Climate Change, eds T. Stocker, D. Qin, G. K. Plattner, M. Tignor, S. Allen, J. Boschung, A. Nauels, Y. Xia, V. Bex, and P. Midgley (Cambridge: Cambridge University Press), 465–570.
Duarte, C., Sintes, T., and Marba, N. (2013). Assessing the co|2| capture potential of seagrass restoration projects. J. App. Ecol. 50, 1341–1349. doi: 10.1111/1365-2664.12155
Dutreuil, S., Bopp, L., and Tagliabue, A. (2009). Impact of enhanced vertical mixing on marine biogeochemistry: lessons for geo-engineering and natural variability. Biogeosciences 6, 901–912. doi: 10.5194/bg-6-901-2009
Feng, E., Keller, D., Koeve, W., and Oschlies, A. (2016). Could artificial ocean alkalinization protect tropical coral ecosystems from ocean acidification? Environ. Res. Lett. 11:074008. doi: 10.1088/1748-9326/11/7/074008
Fennel, K. (2008). Widespread implementation of controlled upwelling in the north pacific subtropical gyre would counteract diazotrophic n2 fixation. Mar. Ecol. Prog. Ser. 371, 301–303. doi: 10.3354/meps07772
Gallo, N., Victor, D., and Levin, L. (2017). Ocean commitments under the paris agreement. Nat. Clim. Change 7, 833–838. doi: 10.1038/nclimate3422
Gattuso, J.-P., Magnan, A., Bopp, L., Cheung, W., Duarte, C., Hinkel, J., et al. (2018). Ocean solutions to address climate change and its effects on marine ecosystems. Front. Mar. Sci. 5:337. doi: 10.3389/fmars.2018.00337
Gattuso, J.-P., Magnan, A. K., Gallo, N., Herr, D., Rochette, J., Vallejo, L., et al. (2019). Opportunities for Increasing Ocean Action in Climate Strategies. Iddri Policy Brief 02/19:1-4. Available online at: https://www.iddri.org/en/publications-and-events/policy-brief/opportunities-increasing-ocean-action-climate-strategies
GESAMP (2019). High level review of a wide range of proposed marine geoengineering techniques. GESAMP Rep. Stud. 98, 1–143.
Gonzalez, M., and Ilyina, T. (2016). Impacts of artificial ocean alkalinization on the carbon cycle and climate in earth system simulations. Geophys. Res. Lett. 43, 6493–6502. doi: 10.1002/2016GL068576
Hamilton, S., and Friess, D. (2018). Global carbon stocks and potential emissions due to mangrove deforestation from 2000 to 2012. Nat. Clim. Change 8, 240–244. doi: 10.1038/s41558-018-0090-4
Harrison, D. (2013). A method for estimating the cost to sequester carbon dioxide by delivering iron to the ocean. Int. J. Glob. Warming 5, 231–254. doi: 10.1504/IJGW.2013.055360
Harrison, D. (2017). Global negative emissions capacity of ocean macronutrient fertilization. Environ. Res. Lett. 12:035001. doi: 10.1088/1748-9326/aa5ef5
Harvey, L. (2008). Mitigating the atmospheric co|2| increase and ocean acidification by adding limestone powder to upwelling regions. J. Geophys. Res. 113:C04028. doi: 10.1029/2007JC004373
Hoegh-Guldberg, O., Caldeira, K., Chopin, T., Gaines, S., Haugan, P., Hemer, M., et al. (2019). The Ocean as a Solution to Climate Change: Five Opportunities for Action. World Resources Institute, Washington, DC.
Honegger, M., Michaelowa, A., and Roy, J. (2020). Potential implications of carbon dioxide removal for the sustainable development goals. Climate Policy. doi: 10.1080/14693062.2020.1843388
Howard, J., Hoyt, S., Isensee, K., Telszewski, M., and Pidgeon, E., (eds.). (2014). Coastal Blue Carbon: Methods for Assessing Carbon Stocks and Emissions Factors in Mangroves, Tidal Salt Marshes, and Seagrasses. Conservation International, Intergovernmental Oceanographic Commission of UNESCO, International Union for Conservation of Nature, Arlington, VA.
Howard, J., Sutton-Grier, A., Herr, D., Kleypas, J., Landis, E., McLeod, E., et al. (2017). Clarifying the role of coastal and marine systems in climate mitigation. Front. Ecol. Environ. 15, 42–50. doi: 10.1002/fee.1451
Ilyina, T., Wolf-Gladrow, D., Munhoven, G., and Heinze, C. (2013). Assessing the potential of calcium-based artificial ocean alkalinization to mitigate rising atmospheric co|2| and ocean acidification. Geophys. Res. Lett. 40, 5909–5914. doi: 10.1002/2013GL057981
IPCC (2018). “Summary for policymakers,” in Global warming of 1.5°C. An IPCC Special Report on the Impacts of Global Warming of 1.5°C Above Pre-Industrial Levels and Related Global Greenhouse Gas Emission Pathways, in the Context of Strengthening the Global Response to the Threat of Climate Change, Sustainable Development, and Efforts to Eradicate Poverty, eds V. Masson-Delmotte, P. Zhai, H. O. Portner, D. Roberts, J. Skea, P. Shukla, A. Pirani, W. Moufouma-Okia, C. Pan, R. Pidcock, S. Connors, J. Matthews, Y. Chen, X. Zhou, M. Gomis, E. Lonnoy, T. Maycock, M. Tignor, and T. Waterfield (Geneva: Intergovernmental Panel on Climate Change), 32.
IPCC (2019). “Summary for policymakers,” in IPCC Special Report on the Ocean and Cryosphere in a Changing Climate, eds H. O. Portner, D. Roberts, V. Masson-Delmotte, P. Zhai, E. Poloczanska, K. Mintenbeck, M. Nicolai, A. Okem, and J. Petzold (Geneva: Intergovernmental Panel on Climate Change), 3–35.
Jones, I. (2014). The cost of carbon management using ocean nourishment. Int. J. Clim. Change Strat. Manag. 6, 391–400. doi: 10.1108/IJCCSM-11-2012-0063
Karl, D., and Letelier, R. (2008). Nitrogen fixation-enhanced carbon sequestration in low nitrate, low chlorophyll seascapes. Mar. Ecol. Prog. Ser. 364, 257–268. doi: 10.3354/meps07547
Keller, D., Feng, E., and Oschlies, A. (2014). Potential climate engineering effectiveness and side effects during a high carbon dioxide-emission scenario. Nat. Commun. 5:3304. doi: 10.1038/ncomms4304
Köhler, P., Abrams, J., Völker, C., Hauck, J., and Wolf-Gladrow, D. (2013). Geoengineering impact of open ocean dissolution of olivine on atmospheric CO2, surface ocean pH and marine biology. Environ. Res. Lett. 8:014009. doi: 10.1088/1748-9326/8/1/014009
Lenton, T. (2014). The global potential for carbon dioxide removal. Iss. Environ. Sci. Technol. 38, 52–79. doi: 10.1039/9781782621225-00052
Lovelock, J., and Rapley, C. (2007). Ocean pipes could help the earth to cure itself. Nature 449, 403–403. doi: 10.1038/449403a
Marbà, N., Arias-Ortiz, A., Masqué, P., Kendrick, G., Mazarrasa, I., Bastyan, G., et al. (2015). Impact of seagrass loss and subsequent revegetation on carbon sequestration and stocks. J. Ecol. 103, 296–302. doi: 10.1111/1365-2745.12370
McGee, J., Brent, K., and Burns, W. (2018). Geoengineering the oceans: an emerging frontier in international climate change governance. Aust. J. of Marit. Ocean Affairs 10, 67–80. doi: 10.1080/18366503.2017.1400899
McLeod, E., Chmura, G., Bouillon, S., Salm, R., Björk, M., Duarte, C., et al. (2011). A blueprint for blue carbon: toward an improved understanding of the role of vegetated coastal habitats in sequestering co|2|. Front. Ecol. Environ. 9, 552–560. doi: 10.1890/110004
Narayan, S., Beck, M., Wilson, P., Thomas, C., Guerrero, A., Shepard, C., et al. (2016). Coastal Wetlands and Flood Damage Reduction: Using Risk Industry-Based Models to Assess Natural Defenses in the Northeastern USA. Lloyd's Tercentenary Research Foundation, London.
National Academies of Sciences Engineering and Medicine (2018). Negative Emissions Technologies and Reliable Sequestration. The National Academies Press, Washington, DC.
Oschlies, A., Koeve, W., Rickels, W., and Rehdanz, K. (2010). Side effects and accounting aspects of hypothetical large-scale southern ocean iron fertilization. Biogeosciences 7, 4017–4035. doi: 10.5194/bg-7-4017-2010
Pan, Y., Fan, W., Zhang, D., Chen, J., Huang, H., Liu, S., et al. (2016). Research progress in artificial upwelling and its potential environmental effects. Sci. China Earth Sci. 59, 236–248. doi: 10.1007/s11430-015-5195-2
Pendleton, L., Donato, D., Murray, B., Crooks, S., Jenkins, W., Sifleet, S., et al. (2012). Estimating global “blue carbon” emissions from conversion and degradation of vegetated coastal ecosystems. PLoS ONE 7:e43542. doi: 10.1371/journal.pone.0043542
Renforth, P., and Henderson, G. (2017). Assessing ocean alkalinity for carbon sequestration. Rev. Geophys. 55, 636–674. doi: 10.1002/2016RG000533
Renforth, P., Jenkins, B., and Kruger, T. (2013). Engineering challenges of ocean liming. Energy 60, 442–452. doi: 10.1016/j.energy.2013.08.006
Reynolds, L., Waycott, M., McGlathery, K., and Orth, R. (2016). Ecosystem services returned through seagrass restoration. Restor. Ecol. 24, 583–588. doi: 10.1111/rec.12360
Rosentreter, J. A., and Williamson, P. (2020). Concerns and uncertainties relating to methane emissions synthesis for vegetated coastal ecosystems. Glob. Change Biol. 26, 5351–5352. doi: 10.1111/gcb.15201
Saderne, V., Geraldi, N., Macreadie, P., Maher, D., Middelburg, J., Serrano, O., et al. (2019). Role of carbonate burial in blue carbon budgets. Nat. Commun. 10:1106. doi: 10.1038/s41467-019-08842-6
Shepherd, J., Iglesias-Rodriguez, D., and Yool, A. (2007). Geo-engineering might cause, not cure, problems. Nature 449, 781–781. doi: 10.1038/449781a
Siikamaki, J., Sanchirico, J., and Jardine, S. (2012). Global economic potential for reducing carbon dioxide emissions from mangrove loss. Proc. Nat. Acad. Sci. U.S.A. 109, 14369–14374. doi: 10.1073/pnas.1200519109
Waycott, M., Duarte, C., Carruthers, T., Orth, R., Dennison, W., Olyarnik, S., et al. (2009). Accelerating loss of seagrasses across the globe threatens coastal ecosystems. Proc. Nat. Acad. Sci. U.S.A. 106:12377. doi: 10.1073/pnas.0905620106
Williamson, P., and Bodle, R. (2016). Update on Climate Geoengineering in Relation to the Convention on Biological Diversity: Potential Impacts and Regulatory Framework, CBD Tech. Ser. 84. Secretariat of the Convention on Biological Diversity, Montreal.
Williamson, P., Boyd, P., Harrison, D., Reynard, N., and Mashayekhi, A. (2021). “Biologically-based negative emissions in the open ocean and coastal seas,” in Negative Emission Technologies, eds M. Bui and N. Mac Dowell (London: Royal Society of Chemistry).
Yool, A., Shepherd, J., Bryden, H., and Oschlies, A. (2009). Low efficiency of nutrient translocation for enhancing oceanic uptake of carbon dioxide. J. Geophys. Res. 114:C08009. doi: 10.1029/2008JC004792
Keywords: ocean-based solutions, blue carbon, carbon capture and storage, ocean fertilization, ocean alkalinization, climate action
Citation: Gattuso J-P, Williamson P, Duarte CM and Magnan AK (2021) The Potential for Ocean-Based Climate Action: Negative Emissions Technologies and Beyond. Front. Clim. 2:575716. doi: 10.3389/fclim.2020.575716
Received: 24 June 2020; Accepted: 23 December 2020;
Published: 25 January 2021.
Edited by:
Wilfried Rickels, Christian Albrechts University Kiel, GermanyReviewed by:
Greg H. Rau, University of California, Santa Cruz, United StatesChristine Bertram, Christian Albrechts University Kiel, Germany
Copyright © 2021 Gattuso, Williamson, Duarte and Magnan. This is an open-access article distributed under the terms of the Creative Commons Attribution License (CC BY). The use, distribution or reproduction in other forums is permitted, provided the original author(s) and the copyright owner(s) are credited and that the original publication in this journal is cited, in accordance with accepted academic practice. No use, distribution or reproduction is permitted which does not comply with these terms.
*Correspondence: Jean-Pierre Gattuso, Z2F0dHVzbyYjeDAwMDQwO29icy12bGZyLmZy