- State Key Laboratory of Oral Diseases, National Clinical Research Center for Oral Diseases, Chengdu, Sichuan, China
Over the past decade, the association between oral health, intestinal microbiota, and systemic diseases has been further validated. Some oral microbial species have been isolated from pathological intestine mucosa or feces and identified as biomarkers for intestinal diseases. A small proportion of oral microbiome passes through or colonizes the lower gastrointestinal tract, even in healthy individuals. Opportunistic pathogens from the oral cavity may expand and participate in the occurrence and progression of intestinal diseases when the anatomical barrier is disrupted. These disruptors interact with the intestinal microbiota, disturbing indigenous microorganisms, and mucosal barriers through direct colonization, blood circulation, or derived metabolite pathways. While interacting with the host’s immune system, oral-derived pathogens stimulate inflammation responses and guide the transition of the intestinal microenvironment from a healthy state to a pre-disease state. Therefore, the oral-gut microbiome axis sheds light on new clinical therapy options, and gastrointestinal tract ecology balance necessitates simultaneous consideration of both oral and gut microbiomes. This review summarizes possible routes of oral microbes entering the intestine and the effects of certain oral bacteria on intestinal microbiota and the host’s immune responses.
1 Introduction
For human beings, the slow-flowing and weakly acidic colon dominate the largest, densest, and most diverse microbial community, with a capacity of up to 100 billion to one trillion cells per milliliter of intestinal contents, acting as a highly efficient bioreactor (Sender et al., 2016). Functional heterogeneity of each intestinal segment determines the diversity of microbial populations in different anatomical regions, and various intestinal microbiotas sustain intestinal physiological development and epithelial homeostasis. Thus, intestinal microbiota dysbiosis is deeply correlate with gut diseases such as irritable bowel syndrome (IBS), inflammatory bowel disease (IBD), colorectal cancer (CRC), and even systemic metabolism or inflammatory diseases (e.g., rheumatism, obesity, etc.) (Priya et al., 2022). Gut microbes-derived metabolite pathways disrupt intestinal homeostasis and proceed to intestinal diseases via regulating the production of short-chain fatty acids (SCFAs) and trimethylamine, or distantly signal to parenteral organs and the immune system through releasing microbes or metabolites into the bloodstream (McPherson et al., 2021).
The oral and intestine are anatomically contiguous in the gastrointestinal (GI) tract, which provides chances for expanded oral pathobionts being ingested and translocated to the gut, suggesting the role of oral microbes in regulating intestinal microecology. However, many current studies separately focus on these two regions in an organ-specific manner without considering microbial translocation. The oral contains various microhabitats that are exposed or not exposed to the air. Tooth surfaces, tongue, mucous membrane, and gums are habitats of aerobe microbes whereas periodontal pockets mainly resident facultative anaerobic and anaerobic microbes, potentially act as complementary reservoirs for opportunistic enteric pathogens. However, the prerequisites for ectopic colonization of oral microbes are harsh. The majority of oral pathobionts are inactivated by gastric acidity, bile acid, colonization resistance of native microbiotas, and the immune system. As a validation, proton pump inhibitors (PPIs) users and achlorhydria patients exhibit an abnormal increase in gut colonization by oral pathobionts (Jackson et al., 2016). In recent years, experimental and sequencing research have successively revealed biological homologies between oral and intestinal flora, indicating the potential value of the oral-gut axis in precise diagnosis, effective treatment, and prognosis of gut diseases (Park et al., 2021; Bao et al., 2022). For instance, saliva could be used as a biomarker to investigate gastrointestinal status conveniently and non-invasively (Wang et al., 2021b).
Hundreds of proteins and peptides released by oral microbes could be metabolized into a variety of bioactive by-products, many of which are toxic (Barbour et al., 2022). Consequently, these metabolites possibly explain the ability that oral-to-gut microbial transmissions to shape or reshape the intestinal microbial ecosystem and eventually modulate the pathogenesis. In the intestine, oral microorganisms participate in various immunoactivity and inflammatory pathways. Saliva translocated mice intestine model shows altered cytokines, chemokines, and tight junction protein compositions, indicating the induced intestinal inflammation and enhanced mucosa permeability (Tsuzuno et al., 2021; Bao et al., 2022). The damaged intestinal mucosa increases antigen exposure, which indirectly activates immune cells and upregulates systemic inflammatory factors. In parallel, the ectopic oral pathobionts, rather than gut-resident microbes, activate the periodontist-induced Th17-skewed T cells that are imprinted with gut tropism (Kitamoto et al., 2020). Hence, regarding clinical therapy, oral microbes shall be considered synchronously and comprehensively with intestinal native microbes in designing treatment regimens.
It is not fully understood the exact oral microbes that influence gut microbiota and the mechanism of these intruders participating in intestinal diseases, further elucidations are still required. As the oral microbiome can exhibit a stable state of at least 3 months, and the gut microbiome for up to 5 years, the crosstalk between oral and intestinal microecology is pathological and clinically valuable (Faith et al., 2013; Wei et al., 2021). In this review, we highlighted the interconnections and translocation route between the oral cavity and the intestine, the immune-resistant strategy from the host, and the mechanism of the oral microorganism interacting with the intestinal ecology to provide a reference for the research frontier concerning the impact of oral microbes on intestinal microbiota.
2 Pathways of oral microorganisms entering the intestine
2.1 Enteral route
Normal humans produce 0.75-1.5L saliva per day, which contains a considerable number of microbes. In mice experiments that establish oral microbial infection through oral administration or gavage of the saliva from periodontitis patients, an abnormal enrichment of oral pathobionts that were confirmed as secondary components of the salivary microbiota was detected in mice’s intestines (Atarashi et al., 2017; Bao et al., 2022). For human beings, most oral species (including core Soral taxa, Streptococcus, Veillonella, Actinomyces, and Haemophilus) that result in endogenous infection emerged in the GI tract in health and disease status, with high levels of variation across individuals (Schmidt et al., 2019) (Figure 1).
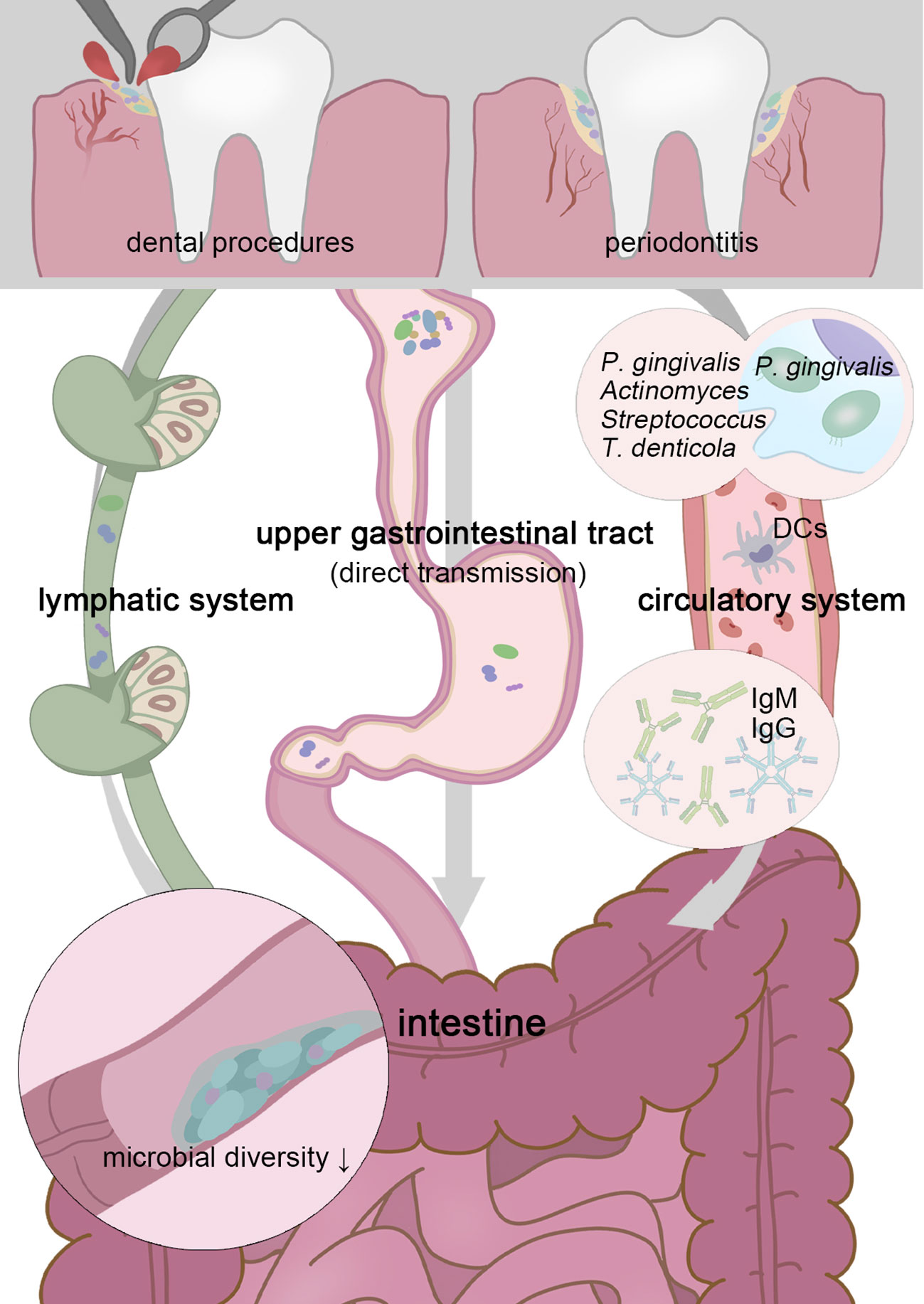
Figure 1 Transient physiological bacteremia triggered by periodontitis and dental procedures permits oral pathogens to stretch themselves systemically. Patients with periodontitis possess a less diverse gut microbiota, and higher possession of oral taxa in the intestine gives rise to more serious gut inflammation (Lourenvarsigmao et al., 2018). To tolerate the inactivation of stomach acid and bile acid, these unexpected “visitors” are expected to be highly acid-resistant. Moreover, P. gingivalis and F. nucleatum can be parasitic on dendritic cells or macrophages and disrupt extraoral tissues (Carrion et al., 2012; Xue et al., 2018). Oral microbes, including P. gingivalis, Actinomyces, Streptococcus, and Treponema denticola, have been confirmed as sources of non-oral organs in a colonizing manner (Figuero et al., 2014; Zhang et al., 2021; Deppe et al., 2022). Chronic oral infection establishes F. nucleatum colonization that significantly induces systemic humoral IgG and IgM antibody responses (Velsko et al., 2015).
2.1.1 Duodenum
High-throughput DNA sequencing of oral, duodenal, and pancreatic samples reveals distinct strain-level clustering patterns for each anatomical region and microbiome overlaps between them (Barlow et al., 2021). This similarity is further extended in the context of GI diseases, and absolute loads of ectopic colonized microbes are positively correlate with more severe GI symptoms. Dyspepsia patients’ duodenum components behave oral-like, including organisms from the HACEK group, Klebsiella, Escherichia, Enterococcus, and Clostridium, replace the ordinary strict anaerobes (Barlow et al., 2021; Chung et al., 2021). Moreover, automatically connected as the gate towards the pancreas, the oral bacterium in the duodenum may be capable of entering the pancreas. For instance, the periodontal microbes (Porphyromonas gingivalis and Aggregatibacter actinomycetemcomitans) have been proven positively related to pancreatic cancer risk (Fan et al., 2018; Nagata et al., 2022; Tan et al., 2022).
2.1.2 Intestinal canal
The oral microflora owns the highest level of variation and the most diverse library of unique sequences, whereas this diversity gradually decreases from the oral to the distal gut, indicating that the microbiome undergoes selective pressure (Richardson et al., 2020). If successfully reaching the intestine, these intruders are usually pathogenic. In the intestine, oral-derived microbiotas create an inflammatory and immunosuppressive microenvironment suitable for tumorigenesis. It is detected the existence of oral-originated bacteria (Fusobacterium nucleatum, P. gingivalis, and Parvimonas micra) in CRC tissues, while butyrate-producing and anti-inflammatory microbes that originally lived in the intestine decreased correspondingly (Gao et al., 2021; Zhao et al., 2021). Ectopic oral microbes also participate in the pathogenic process of IBS and IBD (Liu et al., 2018; Baumgartner et al., 2021; Xia et al., 2021). Sequencing and endoscopies of IBS and ulcerative colitis (UC) patients’ intestines reveal a unique and characteristic ecological dysbiosis of dense bacterial biofilms and several biofilm-forming microbial species of dental plaques (Baumgartner et al., 2021; Xia et al., 2021). According to the sequencing of diseased full-thickness colon specimens including the neuromuscular region, the dominance of putative oral pathogens such as Firmicutes (Streptococcus, Staphylococcus, Peptostreptococcus) and Fusobacteria (Fusobacterium) potentially modulate colon motility (Dinakaran et al., 2018). Additionally, despite the shortage of research illustrating oral microbes colonizing in IBS patients’ intestines, the altered composition of oral microbes correlates with the severity of IBS (Fourie et al., 2016).
2.1.3 Accessory organs of the digestive tract
The liver is one of the most-affected organs by biological network fluctuations, and the gut-liver axis is crucial in linking intestinal microbes and extraintestinal organs (Albillos et al., 2020). There are two possible clues for the involvement of oral microbes in the liver-gut axis. During periodontitis, the liver is constantly exposed to pathogenic factors from the oral, periodontitis-related systemic hypo-inflammation and chemokine potentially affect hepatic metabolic pathways. Both fecal and salivary microbiota have independent prognostic value for cirrhosis progress, specifically, via impaired salivary defense (Yonezawa et al., 2021; Jia et al., 2022; Saboo et al., 2022). The subgingival microbiota of cirrhotic patients consists of an uncommon bacterial community, indicating that its origin is from ecological dysregulation by immune system impairment (Jensen et al., 2018). Moreover, gut microbiota dysbiosis induced by the enteral translocation of periodontopathic bacteria weakens the mucous barrier, thus promoting the enterohepatic transfer of hepatotoxins and enterobacteria (Kuraji et al., 2021). Via disturbing intestinal metabolic and immune pathways, Porphyromonas gingivalis accelerates the progression of non-alcoholic fatty liver disease (NAFLD) (Albuquerque-Souza and Sahingur, 2022; Wang et al., 2022). Primary sclerosing cholangitis (PSC) with or without coexisting IBD is another typical manifestation of the altered gut-liver axis (Ruhlemann et al., 2019). Even in the early stages, PSC patients’ stool and mucosa significantly enrich oral pathogenic bacteria such as Streptococcus, Veillonella, and Actinomyces (Streptococcus salivarius is an independent predictor of liver-related three-year mortality) (Ruhlemann et al., 2019; Jia et al., 2022).
2.2 Hematogenous route
Particularly for periodontitis and periapical inflammation patients, transient physiological bacteremia triggered by tooth extraction and periodontal procedures permits oral pathogens to stretch themselves systemically via the circulatory system (Olsen and Yamazaki, 2019)(Figure 1). For severe periodontitis, the subgingival plaque has direct access to the damaged periodontal pocket, bacteria are more likely to infiltrate periodontal blood vessels and gingival epithelium in the context of the increased mucosa permeability, through which, P. gingivalis and F. nucleatum can be parasitic on dendritic cells or macrophages and disrupt extraoral tissues (Carrion et al., 2012; Xue et al., 2018). It is widely acknowledged the linkage (not causation) between periodontal disease and atherosclerotic vascular disease. Oral microbes, including P. gingivalis, Actinomyces, Streptococcus, and Treponema denticola, have been confirmed as sources of infected heart valves, atherosclerotic plaques, and rheumatic heart valves (Figuero et al., 2014; Zhang et al., 2021; Deppe et al., 2022). In this way, pathogens and their metabolites may be disseminated to the intestine similarly. Periodontal patients possess a less diverse gut microbiota, and higher possession of oral taxa in the intestine, which gives rise to more serious gut inflammation (Lourenvarsigmao et al., 2018). A retrospective analysis of individuals’ blood samples indicates that patients with F. nucleatum or Streptococcus pepticus bacteremia are more likely to suffer from subsequent colorectal cancer (Kwong et al., 2018). For clinical CRC diagnosis, plasma is more advantageous than stool in sensitivity and holds a more unique and heterogeneous microbial community, the vast majority of circulating bacterial DNA could retrospect to the gastrointestinal tract and the oral cavity (Xiao et al., 2021).
However, the accurate location of oral microbes and the probability of colonization in the distal intestine remain controversial. Precise amplicon sequence variants analysis accomplished by Armin Rashidi et al. excluded a significant distinction of microorganisms between oral and gut (66 healthy adults from two countries), indicating more clinical pathologic examinations are still required (Rashidi et al., 2021). Vasapolli et al.’ s sequencing regarding healthy subjects’ saliva, mucosa, and stool samples from each segment of the GI tract displayed an approximate absence of major species of the upper GI tract in the lower GI tract (Vasapolli et al., 2019). Similarly, the correlation between oral taxa and pancreatic cancer did not present among an African-American population as previously speculated (Petrick et al., 2022). Via symbiotic analysis, Carr et al. discovered contrasting ARG-species correlations between saliva and stool samples (Carr et al., 2020). Whereas most 16S rRNA gene sequencing analyses only reliably classify taxa to the genus level and do not report species and strains usually. Using shotgun metagenomic sequencing, oral and gut bacterial communities show greater similarity than earlier studies suggested (de Cena et al., 2021; Maki et al., 2021). Moreover, diurnal variations, processing methods, race, and diet are irrelevant interference with measurement results, which requires further consideration in future studies (Superdock and Poole, 2022).
3 Host’s immune strategy for resisting translocation
The gingiva consists of epithelium, blood vessels, lymphatic vessels, etc., which provides a physical and chemical barrier to separate the external and the internal. However, periodontal tissue is highly susceptible to dysbiosis and inflammation induced by opportunistic pathogens that reside in gingival crevicular fluid and periodontal pockets. Pathological periodontal tissues activate immune responses as the regional accumulation of leukocytes and B cells and the increase of IL-1β, IL-6, and TNF (Lu et al., 2016). Lipopolysaccharide (LPS) produced by oral microbes activates the toll-like receptors (TLR)-nuclearfactorkappa-B (NF-κB) pathway, a key factor for innate and adaptive immune responses. Moreover, it is widely acknowledged the correlation between periodontitis and systemic low-grade inflammation (e.g., Diabetes, rheumatoid arthritis, IBD, obesity, and cirrhosis) (D'Aiuto et al., 2018; Kroese et al., 2021; Moskovitz et al., 2021). During periodontitis, systemic immunity feeds back on the pathological periodontal region and affects the intestine nonspecifically. Chronic oral infection establishes ectopic oral F. nucleatum colonization in the intestine and significantly induces systemic humoral IgG and IgM antibody responses (Velsko et al., 2015). In mice models that are orally administrated with saliva pathogens, endotoxemia and intestinal dysbiosis are induced (Kobayashi et al., 2020). Consistent with this, periodontal therapy effectively proves the dysbiosis in stool and saliva, endotoxin, and salivary or serum inflammation factors in cirrhotics (Bajaj et al., 2018). Long-term chronic inflammation even results in an immunization training effect. Myeloid skewing of hematopoietic progenitor cell differentiation which guides the expression of various microbial product receptors, if chronically activated, potentially sustains chronicity of inflammatory pathologies (Table 1) (Hajishengallis and Chavakis, 2021).
The gut has physiologically formulated multiple strategies to resist colonization by non-native bacteria. Complete mucosa protection contains the joint efforts of symbiotic microbiota, surface liquids, and junctional components (Caballero and Pamer, 2015). Via the gingival-intestinal axis, the swallowed saliva transmits enzymes, effector cytokines bacteria, and bioactive inflammatory cell subsets (neutrophils, lymphocytes, and macrophages) to distant locations (Byrd and Gulati, 2021). Macrophages fulfill sentinel functions as the first responder for ectopic microbes (Hausmann et al., 2021). Cells of Paneth autonomously sense LPS via MyD88-dependent TLR, then stimulate antimicrobial cytokines and trigger the downstream NF-κB pathway, which subsequently regulates the production of Th17 cells or Tregs (LPS from P. gingivalis induces a stronger Th2 response while Klebsiella pneumoniae induces a stronger Th1 response) (Vaishnava et al., 2008; Qi et al., 2022). Proinflammatory pathogens and metabolites gradually predispose the gut to an inflammatory state, manifesting as an enhanced expression of IL-1, IL-6, TNF-α, and vascular endothelial growth factor (VEGF), stimulating the release of collagenase, prostaglandin, and thromboxane (Akdis, 2021) (Figure 2). In IBD, whose major pathogenic processes involve the overreaction (hypersensitivity) of the immune response toward commensal intestinal bacteria, the mutation of the nucleotide-binding oligomerization domain 2 (NOD2) gene results in the reduction of antimicrobial peptides produced by Paneth cells (Larabi et al., 2020).
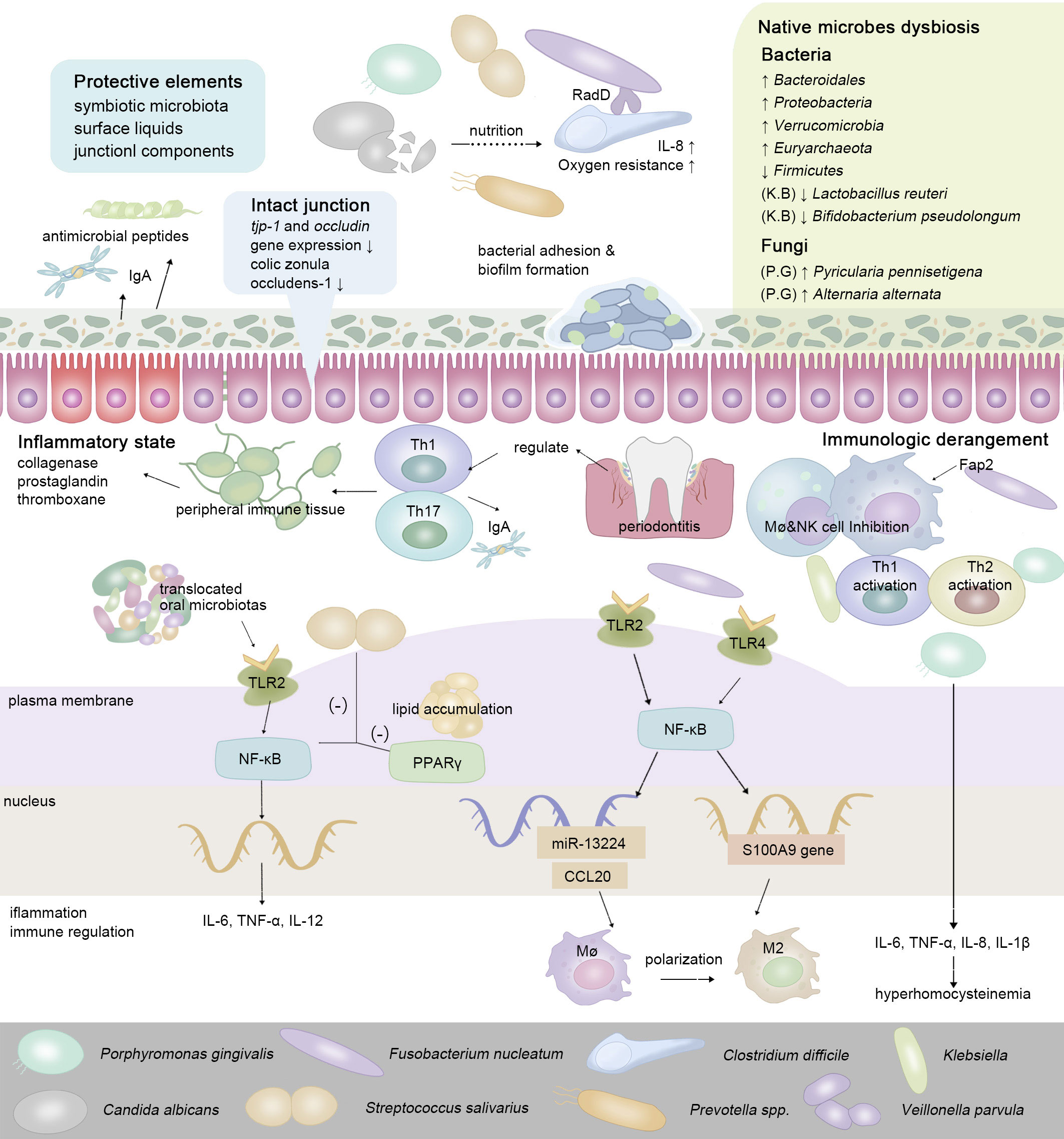
Figure 2 To maintain ecological balance, intestinal protective elements resist extra-intestinal microbes in the oral-derived microbiome dysbiosis. In the intestine, the enteral translocation of periodontopathic bacteria brings about gut microbiota dysbiosis and intestinal inflammation, thus weakening the intestinal surface liquids and junctional components (Nakajima et al., 2015; Feng et al., 2020). While interacting with the host’s immune system, via enteral route or hematogenous route, oral-derived pathogens regulate the constitution and transformation of immune cells, guiding the transition of the intestinal microenvironment from a healthy state to a pre-disease state. In regard to cell signaling pathways, cells of Paneth autonomously sense a series of oral metabolites via MyD88-dependent toll-like receptor (TLR), then stimulate antimicrobial cytokines and trigger the downstream NF-kB pathway, which subsequently regulates the production of a series of inflammatory cytokines (Vaishnava et al., 2008; Qi et al., 2022).
4 Mechanisms of oral microbiome affecting intestinal microbiota
4.1 Porphyromonas gingivalis
In general knowledge, P. gingivalis is a major pathogen for periodontitis and extensively involves in systemic diseases. To disrupt host-microbial homeostasis of the oral cavity, P. gingivalis only needs a microscopic colonization level (<0.01% of the total microbiota presented) (Hajishengallis et al., 2011). Extracellular translocation of P. gingivalis-nucleotide-diphosphate-kinase enzyme (NDKs) promotes microbial molecules to disrupt cytoplasm and establishes continuous infection in mucosal epithelial tissues (Atanasova et al., 2016). In mice experiments, a series of symptoms appeared after P. gingivalis infection (oral administration, gavage, or intravenous injection), including endotoxemia, intestinal inflammation, and systemic hypo-inflammation (Ohtsu et al., 2019; Kashiwagi et al., 2021). Regarding the intestine, P. gingivalis infection gives rise to microbiome dysbiosis. It increases serum endotoxin levels, allowing opportunistic pathogens to colonize in the oral cavity and stimulating the overgrowth of commensal microbes in the intestine (generally upregulates Bacteroidetes and Deferribacteres, and downregulates Firmicutes) (Nakajima et al., 2015; Ohtsu et al., 2019). Pyricularia pennisetigena and Alternaria alternata positively correlated with P. gingivalis administration in the fungal community. Serum metabolites are modified correspondingly with microbial alteration, especially lipid and tryptophan metabolic pathways (Chen et al., 2022). In P. gingivalis-administered mice, the biosynthesis of phenylalanine, tyrosine, and tryptophan in the intestinal microbiota are upregulated, with alanine, glutamine, histidine, tyrosine, and phenylalanine in serum promoted considerably in the serum (Kato et al., 2018). In terms of mechanism, P. gingivalis and its metabolites (e.g., gingipains and extracellular vesicles) activate antigen-presenting cells, initiating intestinal inflammation and immune reactions. Via stimulating TLR2/4 and inducing Th17 cells, P. gingivalis and its metabolites heighten the mRNA expression of TNF-α and IL-1β in the colon (Feng et al., 2020; Tang et al., 2021). Regional inflammation impairs the permeability of the intestinal barrier, specifically, downregulates tjp-1 and occludin gene expression, and decreases colic zonula occludens-1 (Zo-1) level (Nakajima et al., 2015; Feng et al., 2020). Next, the altered proinflammatory cytokines mentioned above modify methionine (Met) and homocysteine (Hcy) metabolism (i.e., 1-carbon metabolism), which potentially stimulates hyperhomocysteinemia (HHcy), another mechanism participating in intestinal dysbiosis (Stanisic et al., 2021). In terms of Th17 cells, a recent study reported a noticeable synergy between periodontal tissue and gut microbes. The intestinal translocation of P. gingivalis enhances Th17 cell differentiation, and mature Th17 cells systemically spread to peripheral immune tissue and then exacerbate intestinal inflammation (Sato et al., 2017; Nagao et al., 2022). Consistent with this, the Th17/Treg imbalance induced by disordered intestinal microbial metabolism directly generates NAFLD in P. gingivalis administration mice (Yao et al., 2022). Compared to normal individuals, CRC patients’ intestines are pathologically colonized with abnormally abundant P. gingivalis, whose infection gives rise to a poor CRC prognosis (Gao et al., 2021). Via activating NLRP3 inflammatory vesicles (activated hematopoietic NOD-like receptor protein 3 inflammasomes) in vitro and in vivo, P. gingivalis triggers low-grade systemic inflammation and intestinal dysbiosis, promoting CRC neoplasia progression (Wang et al., 2021a). Moreover, the peptidoglycan from P. gingivalis molecules induces programmed cell death 1 ligand 1 (PD-L1) up-regulation in colon carcinoma cells and mediates deep inhibition of T cells (involving activation of RIP2 and MAPK signaling pathways, and NOD1 and NOD2) (Adel-Khattab et al., 2021).
4.2 Fusobacterium nucleatum
F. nucleatum normally resides in the intestine but increases synchronously with common oral microorganisms during CRC. 40% of the CRC patients detected identical F. nucleatum strains in both tumor tissue and saliva (Komiya et al., 2019). Moreover, successful periodontitis treatment reduces stool F. nucleatum levels (Yoshihara et al., 2021). Therefore, intestinal pathological F. nucleatum may derive from the oral. The continuum of its species and abundance in the GI tract could be an explanation. Transferring from saliva to the lower GI tract, the species diversity and abundance of F. nucleatum gradually dropped, indicating they resisted the selective stomach pressure (Richardson et al., 2020). Several F. nucleatum subspecies (nucleatum, animalis, vincentii, polymorphum) and potential new subspecies are isolated from the intestine of colorectal cancer patients, and the major encoding virulence factors for F. uncleatum showed evidence of horizontal gene transfer (Tran et al., 2022). Furthermore, intraperitoneal injection successfully established colonization of F. nucleatum in CRC tissue, which suggested the translocation of F. nucleatum through hematogenous route (Abed et al., 2020). Ponath et al. report FoxI, a conserved F. nucleatum oxygen-inducible picornavirus, acting as a post-transcriptional blocker of the major outer membrane porin FomA, is favorable for explicating how F. nucleatum colonizing in diverse segments in the intestine (Ponath et al., 2021).
In mucosal tissues, the adhered F. nucleatum promotes inflammation and stimulates immunoreaction. F. nucleatum and its outer membrane vesicles (OMVs) activate TLR/MyD88/NF-κB pathway, promoting the secretion of a series of proinflammatory cytokines, including IL-8, TNF, keratinocyte-derived chemokine (KC), IL-6, IFN-γ, and monocyte chemoattractant protein-1 (MCP-1) (Chen et al., 2020b; Engevik et al., 2021b). The colonic structure and mucus layers are damaged and infiltrated with immune cells. When regarding UC, this inflammatory process involves a molecular network including caspase activation, recruitment domain 3 (CARD3), and IL-17F (Cao et al., 2020). In addition, the TLR4-dependent mechanism (TLR4/NF-κB/S100A9 axis and NF-κB/miR-1322/CCL20 axis) promotes the M2 polarization of macrophages (M2-Mφ) and immunosuppressive effect, suggesting that oral F. nucleatum evolves more powerful virulence after colonization (Chen et al., 2018). Another virulence factor Fusobacterium produced that participates in immune regulation is the Fap2 protein, which directly interacts with TIGIT, mediates NK cell and T cell inhibition, while T-cell regulates inflammatory factors IL-10, IL-1β, and IL-6 (Gur et al., 2015; Li et al., 2019). Regarding CRC, F. nucleatum and its main pathogenic factors (FadA (binding E-cadherin), Fap2 (a galactose-sensitive hemagglutinin and adhesin binding TIGHT receptors), RadD (autotransporter) and FomA) recruit tumor-infiltrating immune cells, generating tumor microenvironment and participating in immunosuppression and tumorigenesis (Walencik, 2022). F. nucleatum modifies colonic Th17 cell frequency and IL-17 expression recombinant free fatty acid receptor 2 (FFAR2)-dependently (Brennan et al., 2021). Moreover, it positively correlated with the expression of Wnt-β-catenin (activated by FadA─E-cadherin) and REG Iα signaling, both of which effectively promotes the proliferation of sessile serrated adenoma/polyp (Rubinstein et al., 2013; Nishimura et al., 2021). ENO1 gene and ANGPTL4 protein enhance the high glycolysis of colorectal cancer cells, and these two regulatory mechanisms could be upregulated by F. nucleatum either, which further support F. nucleatum colonization and positively collated with poor prognosis (Hong et al., 2021; Zheng et al., 2021). Besides, it is a notable phenomenon in periodontal disease that a variety of bacteria, including F. nucleatum and P. gingivalis, mutually coordinate for coexistence. Presumably, this symbiosis continues to the intestine. In the intestine, F. nucleatum coexists with Clostridium through adhesin RadD, encouraging the intestinal mucus layer’s bacterial biofilm formation in IBD patients. This symbiotic relationship accelerates the extracellular polysaccharides-producing process and chemotaxis level of C. difficile (Engevik et al., 2021a).
4.3 Klebsiella
Klebsiella is an opportunistic pathogen in the GI and respiratory tracts. Although a few Klebsiella physiologically colonizes in the intestine, in pathological states such as periodontitis, it demonstrates signs of ectopic colonization from the oral. Researchers found that saliva K. pneumonia of IBD patients rapidly establishes colonization in mice’s intestines, triggering a strong inflammatory response (upregulates IFN-γ, TH1 in the lamina propria, raises epithelial TNF-α mRNA expression in the proximal colon, and significantly induces IL-17, IFN-γ and CD4+ T cells level) (Atarashi et al., 2017). In mice models that were orally administrated with K. pneumoniae, the hypervirulent strain could even translocate from the intestine and lead to hepatic infections (Young et al., 2020). Th17 cell induction acts as a critical step in IBD pathogenesis and involves in regulating intestinal immunity and inflammation. Oral pathobiont-reactive Th17 cells (Klebsiella and Enterobacter species), rather than intestinal native microbes-reactive, translocate into the intestine and affect colitis progress (Kitamoto et al., 2020). Moreover, translocated Klebsiella promotes the secretion of IL-1β (mainly from inflammatory macrophages) via cysteine-11-mediated inflammatory vesicles, activating intestinal inflammasome (Kitamoto et al., 2020). For the intestine-native microbiome, in the K. pneumoniae infection mice model, gut microbial composition (down-regulates Lactobacillus reuteri and Bifidobacterium pseudolongum) and cecal metabolome (SCFAs) are altered (Wu et al., 2020). Whereas sufficient symbiotic microbe protection from Bacteroidetes could prevent the host-to-host transmission and intestinal colonization of K. pneumoniae via IL-36 signaling (Sequeira et al., 2020).
4.4 Streptococcus salivarius
Two-group pheromone-based subsystem BlpRH is the core node coordinating bacteriocin production and integrating signals of competence activation cascade, typically for S. pneumoniae and S. mutans. Whereas human co-salivary Streptococcus lacks functional BlpRH pairs, the couples bacteriocin production and competence commitment is directly guided by the competence signaling system ComRS, which is the undetermined basis for its optimal adaptation, potentially explaining the high prevalence of S. salivarius in the human GI tract (Mignolet et al., 2018). Aside from benefiting oral health, in terms of its effect on intestinal function, the S. salivarius in the lower gastrointestinal tract is crucial for inhabiting intestinal inflammation response. It positively adjusts microbiome composition and intestinal immunity, even in healthy individuals, the predominant intestinal Streptococcus species is S. salivarius (Kaci et al., 2014; Li et al., 2022). It is confirmed that S. salivarius downregulates NF-κB pathway that triggered by LPS and inhibits peroxisome proliferator-activated receptor γ (PPARγ) activation in small intestinal epithelial cells in vitro (Couvigny et al., 2015). Likewise, both mice experiment and metabolic gene sequencing (I-FABP and Angptl4, these gene products regulate intracellular lipid accumulation) revealed S. salivarius’s protective ability to diminish intestinal epithelial inflammation (Kaci et al., 2014; Couvigny et al., 2015). For non-small cell lung cancer patients, S. salivarius and S. agalactiae react upon gastrointestinal-derived CD4+ T cells and effectively activate monocytes to secrete higher levels of IL-6, IL-12 and TNF, or so-called, the Th1- and Th17-skewed cytokines (Ma et al., 2017). However, S. salivarius is also reported to disturb intestinal microbiota composition. Oral S. salivarius was found to be enriched and of concordant strains in both intestinal and oral microbiota in Crohn’s disease patients, and the species diversity of the infected intestine was considerably depleted (Hu et al., 2021).
4.5 Prevotella
Although most Prevotella species physiologically colonize in oral and gut mucosa, a small number of Prevotella show pathobiontic properties and have been confirmed to participate in opportunistic infections. Distinct from intestinal native microbes, Prevotella owns a unique nitrogen assimilation metabolic network that rarely limits their growth. Jong Nam Kim et al. observed different patterns of transcript abundances in genes involving ammonium assimilation between the classical “enteric paradigm” and Pretovella (Kim et al., 2017). Pretovella act as a vital keystone bacterium of the gut. Binding or attaching to other bacteria and epithelium, Pretovella creates a larger infection than in previously infected areas. A higher abundance of P. intermedia and P. nigrescens (Pretovella species that are commonly resident in the oral cavity) was observed in the intestine of CRC patients (Wirbel et al., 2019). In mice experiments, oral administration of P. intermedia induces NAFLD (Yamazaki et al., 2021). In terms of IBD, Prevotella is a potential alternative biomarker for UC, tightly matched with feces and other oral areas (Xun et al., 2018). Moreover, Prevotella predominates in the intestinal microbiome in the preclinical phase of rheumatoid arthritis, promoting pathological progression in a TH17 cell-dependent manner (Alpizar-Rodriguez et al., 2019; Maeda and Takeda, 2019). In a metagenome-wide association study (MWAS) of the gut microbiome from rheumatoid arthritis patients, Toshihiro Kishikawa et al. identified five species that belong to the Prevotella (i.e., P. denticola, P. marshii, P. disiens, P. corporis, and P. amnii) (Kishikawa et al., 2020). Additionally, the major task for intestinal Prevotella is metabolizing various proteins and carbohydrates. Consequently, high animal protein and saturated fat diet western people own higher diversity of inflammation-associated Prevotella in the oral cavity, with intestinal microbes demonstrating upregulated virulence factor and antibiotic resistance gene levels. (Prasoodanan et al., 2021).
4.6 Veillonella
V. parvula is an anaerobic commensal and opportunistic pathogen that plays a crucial role in bacteria adhesion and biofilm formation for oral and gut microbiomes. Type V secreted autotransporters that typically exist in diderm bacteria determine this character. Whereas inactivation of the gene coding for a poorly characterized metal-dependent phosphohydrolase HD domain protein in Firmicutes could inhibit autotransporter-mediated biofilm formation (Bechon et al., 2020). Intestinal Veillonella attaches to the mucosa of the distal gut and positively coexists with Clostridioides in C. difficile colonization (CDC) patients (Crobach et al., 2020). Besides, it was detected the existence of Veillonella and Streptococcus in atherosclerotic plaques, whose bacterial abundances positively correlated with their abundance in the oral cavity, indicating that oral Veillonella probably spread to extraoral organs via the circulatory system (Koren et al., 2011). Veillonella extensively participates in a range of diseases associated with gastrointestinal microbial dysbiosis, for instance, hepatic and gall diseases (autoimmune hepatitis, alcoholic hepatitis, biliary atresia, and PSC) (Kummen et al., 2017; Song et al., 2021). Actually, Veillonella is acid-sensitive, the abundance of Veillonella heightens when aldafermin-mediated suppressions of bile acid synthesis, particularly toxic bile acids, are inhibited (Loomba et al., 2021). When regarding IBD, the enrichment of adhesive bacteria (involving Veillonella) increases Th17 cell activation and luminal secretory IgA (Chen et al., 2020a; Gonzalez-Soltero et al., 2020). In periodontitis-associated type 2 diabetes (T2D), Streptococcus and Veillonella are dominant in the symbiosis network, and microbial shifts may contribute to systemic inflammation and metabolic dysfunction (Li et al., 2020; Letchumanan et al., 2022).
4.7 Candida albicans
It is almost impossible for fungi to survive in healthy people’s intestines, and C. Albicans dominate among the cultivable mycobiota that longitudinally persists. Intestinal C. Albicans probably as commensals constantly supplemented from the oral cavity, frequent teeth cleaning dramatically reduces fecal C. Albicans abundance (Auchtung et al., 2018; Raimondi et al., 2019). Whereas for immunocompromised individuals, intestinal fungi and fungal molecules could result in serious infections and produce severe bacterial sepsis via cytokine storm induction or macrophage-killing activity. C. Albicans gavage worsens the colitis in mice experiments, gut-leakage induces pro-inflammatory cytokines in the intestine and blood, which was hypothesized as a more severe translocation of LPS, serum (1→3)-β-D-glucan (BG) and bacteria from the gut into the systemic circulation (Panpetch et al., 2020). Regarding colonization and dissemination, in the gut, dead fungi are nutrients for bacterial fermentation. In the presence of C. Albicans, the opportunistic bacterium C. difficile can tolerate aerobic conditions, and the p-cresol produced by C. difficile inhibit C. Albian’s hypha formation, biofilm formation, and virulence (van Leeuwen et al., 2016). This symbiotic relationship leads to more severe infection of C. difficile in mice models and increases IL-8 production in experimental intestinal epithelial cell-lines (Panpetch et al., 2019). Regarding the susceptibility to C. Albicans, C. Albicans infection could be promoted by intestinal taurocholic acid (TCA) that weakens mucosal innate and adaptive immune responses (Datta et al., 2022). Besides, the host’s high iron level enhances C. Albicans infection severity and its dissemination from the oral to the gut (Tripathi et al., 2022). However, endogenous invasion by C. Albicans could be prevented by intestinal TLR2, and intravenous immunoglobulin (IVIg) has been proven useful for treating C. Albicans infection and maintaining intestinal homeostasis, which negatively regulates PPARγ and TLR-4 (Prieto et al., 2016; Charlet et al., 2019).
4.8 Other pathogens
The intestinal microecological disorder is a process affecting multi-bacterial composition. In addition to common oral pathogens mentioned above, for instance, Peptostreptococcus stomatis engages in gastrointestinal cancers and is of significant centralities in the gastric carcinoma (GC) ecological network, which mediates the pathogenesis of precancerous gastric atrophy (GA) and intestinal metaplasia (IM) (Coker et al., 2018; Wu et al., 2022). Regarding IBD and CRC, P. stomatis is among the oral-derived biomarker panel of CRC either (Yu et al., 2017; Osman et al., 2021). Gemella enriches in the intestinal mucosa of IBD and CRC, while Mogibacterium is significantly more abundant in patients with adenomatous polyps (Hale et al., 2017; Rengarajan et al., 2020; Avuthu and Guda, 2022). Intestinal bacteriophages could communicate with gut-oral bacterial commensals, which promotes individuals’ virome profile differentiation at the early and late stages of CRC (Nakatsu et al., 2018). In terms of gastrointestinal motility disorders, a polybacterial infection (P. gingivalis, T. denticola, and Tannerella forsythia) is discovered to alter vascular and colonic BH4/nNOS/NRF2 pathways and to damage vascular relaxation and colonic motility (Gangula et al., 2015). In addition, oral lantibiotics (it has a considerable antimicrobial effect on intestinal gram-positive bacteria) produced by S. mutans decrease the diversity of intestinal microbes and reduce the abundance of Firmicutes (Yonezawa et al., 2021).
5 Conclusion and perspectives
Growing evidence supports the associations between oral microbes and intestinal diseases. The prevalence and severity of oral diseases (e.g., periodontitis) are closely linked with the intestinal healthy state. In recent years, several intestinal pathobionts (P. gingivalis, F. nucleatum, P. intermedia, P. nigrescens, C. albicans, etc.) showed potential origin from the oral cavity via direct enteral translocation or through the circulatory system. Using deep learning algorithms, the fecal microbiome could be predicted by the oral microbiome of the same individual in a new bioinformatic tool (Rampelli et al., 2021). For enteral ectopic translocation, oral microbes have to overcome the harsh protective elements (e.g., gastric acid, bile acid, and native microbes). Consequently, the detected oral microorganisms in the lower GI tract are usually low-abundant. With the development of next-generation sequencing (NGS), the consistency between the two sites is more detectable and demonstrates further correlations than in earlier studies. In mice experiments, oral administration of oral pathobionts leads to intestinal dysbiosis, regional intestinal inflammation, and low-grade endotoxemia. Although the amount and method of intake differ from the natural state, it provides references to the possible effect of oral pathobionts on the gut, and more experiments are required to determine the species and proportion of pathobionts, the precise site of their colonization, the time of their presence, and the crosstalk with native microbes and the immune system. The circulatory system, as a channel for bacterial expansion, also facilitates the widespread dissemination of oral pathobionts. Periodontitis is generally accepted associating with systemic low-grade inflammatory diseases. The footprint of the oral-origin microbiome has been detected in extraoral organs including the heart and aortic valves. Oral microbes and their products induce immune responses via the bloodstream, which regulates the host’s overall inflammatory state, stimulating TLR-NF-κB pathway or regulating TH17/Treg ratio. In addition, it is a notable phenomenon for pathogenic microbes such as F. nucleatum, C. difficile, and P. gingivalis communicating and adhering with each other. In mucosal biofilms, interspecies interactions that influence spatial organization modify microbial communities’ formation and function. Hence, it is also interesting to investigate how these oral microbes influence the survival of native microorganisms.
16S rRNA sequencing and conventional bacterial identification and quantification methods are suitable for identifying well-characterized and abundant genera. However, frequently using 1% as the threshold for excluding the low-abundant organisms, some oral-origin microbiome species in the intestine may escape detection unconsciously. The SourceTracker results showed that the percentage of intestinal bacteria from the tongue-coated microflora was within 1% (Guo et al., 2022). Moreover, diet and diurnal variation are independent variables that affect the composition of microbes in the GI tract, which needs further consideration while analyzing microbial communities.
The study of oral-gut microbes has gained popularity, not only for its role in intestinal and systemic diseases that have gradually been explored but also for its offering new approaches to conveniently detectable biomarkers and clinical therapy methods. Various probiotics, prebiotics, and even herbal medicines that work by modifying gut microbes’ variations have been proven of positive therapeutic outcomes. Both saliva and gut microbes are potential biomarkers for diagnosis. This clue indicates an overall idea to manage oral health and intestinal diseases coordinatingly in the future, and this approach is still in its infancy. As the attention to oral health grows, studying the effect of oral microbes on the intestine opens up new insight into preventing and controlling intestinal diseases and discovering new therapies.
Author contributions
YL, ZL, and XP wrote and edited the manuscript. ZL and XP critically reviewed the manuscript and provided insightful discussions and ideas. YL and ZL contributed equally to this work and share first authorship. All authors contributed to the article and approved the submitted version.
Funding
This work is supported by grant 32070120 from the National Natural Science Foundation of China.
Conflict of interest
The authors declare that the research was conducted in the absence of any commercial or financial relationships that could be construed as a potential conflict of interest.
Publisher’s note
All claims expressed in this article are solely those of the authors and do not necessarily represent those of their affiliated organizations, or those of the publisher, the editors and the reviewers. Any product that may be evaluated in this article, or claim that may be made by its manufacturer, is not guaranteed or endorsed by the publisher.
References
Abed, J., Maalouf, N., Manson, A. L., Earl, A. M., Parhi, L., Emgard, J. E. M., et al. (2020). Colon cancer-associated fusobacterium nucleatum may originate from the oral cavity and reach colon tumors via the circulatory system. Front. Cell Infect. Microbiol. 10. doi: 10.3389/fcimb.2020.00400
Adel-Khattab, D., Groeger, S., Domann, E., Chakraborty, T., Lochnit, G., Meyle, J. (2021). Porphyromonas gingivalis induced up-regulation of PD-L1 in colon carcinoma cells. Mol. Oral. Microbiol. 36 (3), 172–181. doi: 10.1111/omi.12332
Akdis, C. A. (2021). Does the epithelial barrier hypothesis explain the increase in allergy, autoimmunity and other chronic conditions? Nat. Rev. Immunol. 21 (11), 739–751. doi: 10.1038/s41577-021-00538-7
Albillos, A., de Gottardi, A., Rescigno, M. (2020). The gut-liver axis in liver disease: Pathophysiological basis for therapy. J. Hepatol. 72 (3), 558–577. doi: 10.1016/j.jhep.2019.10.003
Albuquerque-Souza, E., Sahingur, S. E. (2022). Periodontitis, chronic liver diseases, and the emerging oral-gut-liver axis. Periodontol 2000 89 (1), 125–141. doi: 10.1111/prd.12427
Alpizar-Rodriguez, D., Lesker, T. R., Gronow, A., Gilbert, B., Raemy, E., Lamacchia, C., et al. (2019). Prevotella copri in individuals at risk for rheumatoid arthritis. Ann. Rheum Dis. 78 (5), 590–593. doi: 10.1136/annrheumdis-2018-214514
Atanasova, K., Lee, J., Roberts, J., Lee, K., Ojcius, D. M., Yilmaz, O. (2016). Nucleoside-Diphosphate-Kinase of p. gingivalis is secreted from epithelial cells in the absence of a leader sequence through a pannexin-1 interactome. Sci. Rep. 6, 37643. doi: 10.1038/srep37643
Atarashi, K., Suda, W., Luo, C., Kawaguchi, T., Motoo, I., Narushima, S., et al. (2017). Ectopic colonization of oral bacteria in the intestine drives TH1 cell induction and inflammation. Science 358 (6361), 359–365. doi: 10.1126/science.aan4526
Auchtung, T. A., Fofanova, T. Y., Stewart, C. J., Nash, A. K., Wong, M. C., Gesell, J. R., et al. (2018). Investigating colonization of the healthy adult gastrointestinal tract by fungi. mSphere 3 (2), e00092-18. doi: 10.1128/mSphere.00092-18
Avuthu, N., Guda, C. (2022). Meta-analysis of altered gut microbiota reveals microbial and metabolic biomarkers for colorectal cancer. Microbiol. Spectr. 10 (4), e0001322. doi: 10.1128/spectrum.00013-22
Bajaj, J. S., Matin, P., White, M. B., Fagan, A., Deeb, J. G., Acharya, C., et al. (2018). Periodontal therapy favorably modulates the oral-gut-hepatic axis in cirrhosis. Am. J. Physiol. Gastrointest Liver Physiol. 315 (5), G824–G837. doi: 10.1152/ajpgi.00230.2018
Bao, J., Li, L., Zhang, Y., Wang, M., Chen, F., Ge, S., et al. (2022). Periodontitis may induce gut microbiota dysbiosis via salivary microbiota. Int. J. Oral. Sci. 14 (1), 32. doi: 10.1038/s41368-022-00183-3
Barbour, A., Elebyary, O., Fine, N., Oveisi, M., Glogauer, M. (2022). Metabolites of the oral microbiome: Important mediators of multikingdom interactions. FEMS Microbiol. Rev. 46 (1), fuab039. doi: 10.1093/femsre/fuab039
Barlow, J. T., Leite, G., Romano, A. E., Sedighi, R., Chang, C., Celly, S., et al. (2021). Quantitative sequencing clarifies the role of disruptor taxa, oral microbiota, and strict anaerobes in the human small-intestine microbiome. Microbiome 9 (1), 214. doi: 10.1186/s40168-021-01162-2
Baumgartner, M., Lang, M., Holley, H., Crepaz, D., Hausmann, B., Pjevac, P., et al. (2021). Mucosal biofilms are an endoscopic feature of irritable bowel syndrome and ulcerative colitis. Gastroenterology 161 (4), 1245–1256.e1220. doi: 10.1053/j.gastro.2021.06.024
Bechon, N., Jimenez-Fernandez, A., Witwinowski, J., Bierque, E., Taib, N., Cokelaer, T., et al. (2020). Autotransporters drive biofilm formation and autoaggregation in the diderm firmicute veillonella parvula. J. Bacteriol 202 (21), e00461-20. doi: 10.1128/JB.00461-20
Brennan, C. A., Clay, S. L., Lavoie, S. L., Bae, S., Lang, J. K., Fonseca-Pereira, D., et al. (2021). Fusobacterium nucleatum drives a pro-inflammatory intestinal microenvironment through metabolite receptor-dependent modulation of IL-17 expression. Gut Microbes 13 (1), 1987780. doi: 10.1080/19490976.2021.1987780
Byrd, K. M., Gulati, A. S. (2021). The "Gum-gut" axis in inflammatory bowel diseases: A hypothesis-driven review of associations and advances. Front. Immunol. 12. doi: 10.3389/fimmu.2021.620124
Caballero, S., Pamer, E. G. (2015). Microbiota-mediated inflammation and antimicrobial defense in the intestine. Annu. Rev. Immunol. 33, 227–256. doi: 10.1146/annurev-immunol-032713-120238
Cao, P., Chen, Y., Guo, X., Chen, Y., Su, W., Zhan, N., et al. (2020). Fusobacterium nucleatum activates endoplasmic reticulum stress to promote crohn's disease development via the upregulation of CARD3 expression. Front. Pharmacol. 11. doi: 10.3389/fphar.2020.00106
Carrion, J., Scisci, E., Miles, B., Sabino, G. J., Zeituni, A. E., Gu, Y., et al. (2012). Microbial carriage state of peripheral blood dendritic cells (DCs) in chronic periodontitis influences DC differentiation, atherogenic potential. J. Immunol. 189 (6), 3178–3187. doi: 10.4049/jimmunol.1201053
Carr, V. R., Witherden, E. A., Lee, S., Shoaie, S., Mullany, P., Proctor, G. B., et al. (2020). Abundance and diversity of resistomes differ between healthy human oral cavities and gut. Nat. Commun. 11 (1), 693. doi: 10.1038/s41467-020-14422-w
Charlet, R., Sendid, B., Kaveri, S. V., Poulain, D., Bayry, J., Jawhara, S. (2019). Intravenous immunoglobulin therapy eliminates candida albicans and maintains intestinal homeostasis in a murine model of dextran sulfate sodium-induced colitis. Int. J. Mol. Sci. 20 (6), 1473. doi: 10.3390/ijms20061473
Chen, Y., Chen, Y., Cao, P., Su, W., Zhan, N., Dong, W. (2020b). Fusobacterium nucleatum facilitates ulcerative colitis through activating IL-17F signaling to NF-kappaB via the upregulation of CARD3 expression. J. Pathol. 250 (2), 170–182. doi: 10.1002/path.5358
Chen, T., Li, Q., Wu, J., Wu, Y., Peng, W., Li, H., et al. (2018). Fusobacterium nucleatum promotes M2 polarization of macrophages in the microenvironment of colorectal tumours via a TLR4-dependent mechanism. Cancer Immunol. Immunother. 67 (10), 1635–1646. doi: 10.1007/s00262-018-2233-x
Chen, S., Niu, C., Lv, W. (2022). Multi-omics insights reveal the remodeling of gut mycobiome with p. gingivalis. Front. Cell Infect. Microbiol. 12. doi: 10.3389/fcimb.2022.937725
Chen, B., Ye, D., Luo, L., Liu, W., Peng, K., Shu, X., et al. (2020a). Adhesive bacteria in the terminal ileum of children correlates with increasing Th17 cell activation. Front. Pharmacol. 11. doi: 10.3389/fphar.2020.588560
Chung, M., Zhao, N., Meier, R., Koestler, D. C., Wu, G., de Castillo, E., et al. (2021). Comparisons of oral, intestinal, and pancreatic bacterial microbiomes in patients with pancreatic cancer and other gastrointestinal diseases. J. Oral. Microbiol. 13 (1), 1887680. doi: 10.1080/20002297.2021.1887680
Coker, O. O., Dai, Z., Nie, Y., Zhao, G., Cao, L., Nakatsu, G., et al. (2018). Mucosal microbiome dysbiosis in gastric carcinogenesis. Gut 67 (6), 1024–1032. doi: 10.1136/gutjnl-2017-314281
Couvigny, B., de Wouters, T., Kaci, G., Jacouton, E., Delorme, C., Dore, J., et al. (2015). Commensal streptococcus salivarius modulates PPARgamma transcriptional activity in human intestinal epithelial cells. PloS One 10 (5), e0125371. doi: 10.1371/journal.pone.0125371
Crobach, M. J. T., Ducarmon, Q. R., Terveer, E. M., Harmanus, C., Sanders, I., Verduin, K. M., et al. (2020). The bacterial gut microbiota of adult patients infected, colonized or noncolonized by clostridioides difficile. Microorganisms 8 (5), 677. doi: 10.3390/microorganisms8050677
D'Aiuto, F., Gkranias, N., Bhowruth, D., Khan, T., Orlandi, M., Suvan, J., et al. (2018). Systemic effects of periodontitis treatment in patients with type 2 diabetes: A 12 month, single-centre, investigator-masked, randomised trial. Lancet Diabetes Endocrinol. 6 (12), 954–965. doi: 10.1016/S2213-8587(18)30038-X
Datta, A., Hernandez-Franco, J. F., Park, S., Olson, M. R., HogenEsch, H., Thangamani, S. (2022). Bile acid regulates mononuclear phagocytes and T helper 17 cells to control candida albicans in the intestine. J. Fungi (Basel) 8 (6), 610. doi: 10.3390/jof8060610
de Cena, J. A., Zhang, J., Deng, D., Dame-Teixeira, N., Do, T. (2021). Low-abundant microorganisms: The human microbiome's dark matter, a scoping review. Front. Cell Infect. Microbiol. 11. doi: 10.3389/fcimb.2021.689197
Deppe, H., Reitberger, J., Behr, A. V., Vitanova, K., Lange, R., Wantia, N., et al. (2022). Oral bacteria in infective endocarditis requiring surgery: A retrospective analysis of 134 patients. Clin. Oral. Investig. 26 (7), 4977–4985. doi: 10.1007/s00784-022-04465-2
Dinakaran, V., Mandape, S. N., Shuba, K., Pratap, S., Sakhare, S. S., Tabatabai, M. A., et al. (2018). Identification of specific oral and gut pathogens in full thickness colon of colitis patients: Implications for colon motility. Front. Microbiol. 9. doi: 10.3389/fmicb.2018.03220
Engevik, M. A., Danhof, H. A., Auchtung, J., Endres, B. T., Ruan, W., Basseres, E., et al. (2021a). Fusobacteriumnucleatum adheres to clostridioides difficile via the RadD adhesin to enhance biofilm formation in intestinal mucus. Gastroenterology 160 (4), 1301–1314.e1308. doi: 10.1053/j.gastro.2020.11.034
Engevik, M. A., Danhof, H. A., Ruan, W., Engevik, A. C., Chang-Graham, A. L., Engevik, K. A., et al. (2021b). Fusobacterium nucleatum secretes outer membrane vesicles and promotes intestinal inflammation. mBio 12 (2), e02706-20. doi: 10.1128/mBio.02706-20
Faith, J. J., Guruge, J. L., Charbonneau, M., Subramanian, S., Seedorf, H., Goodman, A. L., et al. (2013). The long-term stability of the human gut microbiota. Science 341 (6141), 1237439. doi: 10.1126/science.1237439
Fan, X., Alekseyenko, A. V., Wu, J., Peters, B. A., Jacobs, E. J., Gapstur, S. M., et al. (2018). Human oral microbiome and prospective risk for pancreatic cancer: A population-based nested case-control study. Gut 67 (1), 120–127. doi: 10.1136/gutjnl-2016-312580
Feng, Y. K., Wu, Q. L., Peng, Y. W., Liang, F. Y., You, H. J., Feng, Y. W., et al. (2020). Oral p. gingivalis impairs gut permeability and mediates immune responses associated with neurodegeneration in LRRK2 R1441G mice. J. Neuroinflamm. 17 (1), 347. doi: 10.1186/s12974-020-02027-5
Figuero, E., Lindahl, C., Marin, M. J., Renvert, S., Herrera, D., Ohlsson, O., et al. (2014). Quantification of periodontal pathogens in vascular, blood, and subgingival samples from patients with peripheral arterial disease or abdominal aortic aneurysms. J. Periodontol 85 (9), 1182–1193. doi: 10.1902/jop.2014.130604
Fourie, N. H., Wang, D., Abey, S. K., Sherwin, L. B., Joseph, P. V., Rahim-Williams, B., et al. (2016). The microbiome of the oral mucosa in irritable bowel syndrome. Gut Microbes 7 (4), 286–301. doi: 10.1080/19490976.2016.1162363
Gangula, P., Ravella, K., Chukkapalli, S., Rivera, M., Srinivasan, S., Hale, A., et al. (2015). Polybacterial periodontal pathogens alter vascular and gut BH4/nNOS/NRF2-phase II enzyme expression. PloS One 10 (6), e0129885. doi: 10.1371/journal.pone.0129885
Gao, R., Zhu, Y., Kong, C., Xia, K., Li, H., Zhu, Y., et al. (2021). Alterations, interactions, and diagnostic potential of gut bacteria and viruses in colorectal cancer. Front. Cell Infect. Microbiol. 11. doi: 10.3389/fcimb.2021.657867
Gonzalez-Soltero, R., Bailen, M., de Lucas, B., Ramirez-Goercke, M. I., Pareja-Galeano, H., Larrosa, M. (2020). Role of oral and gut microbiota in dietary nitrate metabolism and its impact on sports performance. Nutrients 12 (12), 3611. doi: 10.3390/nu12123611
Guo, X. J., Jiang, T., Ma, X. X., Hu, X. J., Huang, J. B., Cui, L. T., et al. (2022). Relationships between diurnal changes of tongue coating microbiota and intestinal microbiota. Front. Cell Infect. Microbiol. 12. doi: 10.3389/fcimb.2022.813790
Gur, C., Ibrahim, Y., Isaacson, B., Yamin, R., Abed, J., Gamliel, M., et al. (2015). Binding of the Fap2 protein of fusobacterium nucleatum to human inhibitory receptor TIGIT protects tumors from immune cell attack. Immunity 42 (2), 344–355. doi: 10.1016/j.immuni.2015.01.010
Hajishengallis, G., Chavakis, T. (2021). Local and systemic mechanisms linking periodontal disease and inflammatory comorbidities. Nat. Rev. Immunol. 21 (7), 426–440. doi: 10.1038/s41577-020-00488-6
Hajishengallis, G., Liang, S., Payne, M. A., Hashim, A., Jotwani, R., Eskan, M. A., et al. (2011). Low-abundance biofilm species orchestrates inflammatory periodontal disease through the commensal microbiota and complement. Cell Host Microbe 10 (5), 497–506. doi: 10.1016/j.chom.2011.10.006
Hale, V. L., Chen, J., Johnson, S., Harrington, S. C., Yab, T. C., Smyrk, T. C., et al. (2017). Shifts in the fecal microbiota associated with adenomatous polyps. Cancer Epidemiol. Biomarkers Prev. 26 (1), 85–94. doi: 10.1158/1055-9965.EPI-16-0337
Hausmann, A., Felmy, B., Kunz, L., Kroon, S., Berthold, D. L., Ganz, G., et al. (2021). Intercrypt sentinel macrophages tune antibacterial NF-kappaB responses in gut epithelial cells via TNF. J. Exp. Med. 218 (11), e20210862. doi: 10.1084/jem.20210862
Hong, J., Guo, F., Lu, S. Y., Shen, C., Ma, D., Zhang, X., et al. (2021). F. nucleatum targets lncRNA ENO1-IT1 to promote glycolysis and oncogenesis in colorectal cancer. Gut 70 (11), 2123–2137. doi: 10.1136/gutjnl-2020-322780
Hu, S., Png, E., Gowans, M., Ong, D. E. H., de Sessions, P. F., Song, J., et al. (2021). Ectopic gut colonization: A metagenomic study of the oral and gut microbiome in crohn's disease. Gut Pathog. 13 (1), 13. doi: 10.1186/s13099-021-00409-5
Jackson, M. A., Goodrich, J. K., Maxan, M. E., Freedberg, D. E., Abrams, J. A., Poole, A. C., et al. (2016). Proton pump inhibitors alter the composition of the gut microbiota. Gut 65 (5), 749–756. doi: 10.1136/gutjnl-2015-310861
Jensen, A., Ladegaard Gronkjaer, L., Holmstrup, P., Vilstrup, H., Kilian, M. (2018). Unique subgingival microbiota associated with periodontitis in cirrhosis patients. Sci. Rep. 8 (1), 10718. doi: 10.1038/s41598-018-28905-w
Jia, B., Kim, K. H., Ruan, W., Kim, H. M., Jeon, C. O. (2022). Lantibiotic-encoding streptococcus in the human microbiome are underlying risk factors for liver diseases. J. Infect. 84 (5), e70–e72. doi: 10.1016/j.jinf.2022.02.020
Kaci, G., Goudercourt, D., Dennin, V., Pot, B., Dore, J., Ehrlich, S. D., et al. (2014). Anti-inflammatory properties of streptococcus salivarius, a commensal bacterium of the oral cavity and digestive tract. Appl. Environ. Microbiol. 80 (3), 928–934. doi: 10.1128/AEM.03133-13
Kashiwagi, Y., Aburaya, S., Sugiyama, N., Narukawa, Y., Sakamoto, Y., Takahashi, M., et al. (2021). Porphyromonas gingivalis induces entero-hepatic metabolic derangements with alteration of gut microbiota in a type 2 diabetes mouse model. Sci. Rep. 11 (1), 18398. doi: 10.1038/s41598-021-97868-2
Kato, T., Yamazaki, K., Nakajima, M., Date, Y., Kikuchi, J., Hase, K., et al. (2018). Oral administration of porphyromonas gingivalis alters the gut microbiome and serum metabolome. mSphere 3 (5), e00460-18. doi: 10.1128/mSphere.00460-18
Kim, J. N., Mendez-Garcia, C., Geier, R. R., Iakiviak, M., Chang, J., Cann, I., et al. (2017). Metabolic networks for nitrogen utilization in prevotella ruminicola 23. Sci. Rep. 7 (1), 7851. doi: 10.1038/s41598-017-08463-3
Kishikawa, T., Maeda, Y., Nii, T., Motooka, D., Matsumoto, Y., Matsushita, M., et al. (2020). Metagenome-wide association study of gut microbiome revealed novel aetiology of rheumatoid arthritis in the Japanese population. Ann. Rheum Dis. 79 (1), 103–111. doi: 10.1136/annrheumdis-2019-215743
Kitamoto, S., Nagao-Kitamoto, H., Jiao, Y., Gillilland, M. G., 3rd, Hayashi, A., Imai, J., et al. (2020). The intermucosal connection between the mouth and gut in commensal pathobiont-driven colitis. Cell 182 (2), 447–462.e414. doi: 10.1016/j.cell.2020.05.048
Kobayashi, R., Ogawa, Y., Hashizume-Takizawa, T., Kurita-Ochiai, T. (2020). Oral bacteria affect the gut microbiome and intestinal immunity. Pathog. Dis. 78 (3), ftaa024. doi: 10.1093/femspd/ftaa024
Komiya, Y., Shimomura, Y., Higurashi, T., Sugi, Y., Arimoto, J., Umezawa, S., et al. (2019). Patients with colorectal cancer have identical strains of fusobacterium nucleatum in their colorectal cancer and oral cavity. Gut 68 (7), 1335–1337. doi: 10.1136/gutjnl-2018-316661
Koren, O., Spor, A., Felin, J., Fak, F., Stombaugh, J., Tremaroli, V., et al. (2011). Human oral, gut, and plaque microbiota in patients with atherosclerosis. Proc. Natl. Acad. Sci. U.S.A. 108 Suppl 1 (Suppl 1), 4592–4598. doi: 10.1073/pnas.1011383107
Kroese, J. M., Brandt, B. W., Buijs, M. J., Crielaard, W., Lobbezoo, F., Loos, B. G., et al. (2021). Differences in the oral microbiome in patients with early rheumatoid arthritis and individuals at risk of rheumatoid arthritis compared to healthy individuals. Arthritis Rheumatol 73 (11), 1986–1993. doi: 10.1002/art.41780
Kummen, M., Holm, K., Anmarkrud, J. A., Nygard, S., Vesterhus, M., Hoivik, M. L., et al. (2017). The gut microbial profile in patients with primary sclerosing cholangitis is distinct from patients with ulcerative colitis without biliary disease and healthy controls. Gut 66 (4), 611–619. doi: 10.1136/gutjnl-2015-310500
Kuraji, R., Sekino, S., Kapila, Y., Numabe, Y. (2021). Periodontal disease-related nonalcoholic fatty liver disease and nonalcoholic steatohepatitis: An emerging concept of oral-liver axis. Periodontol 2000 87 (1), 204–240. doi: 10.1111/prd.12387
Kwong, T. N. Y., Wang, X., Nakatsu, G., Chow, T. C., Tipoe, T., Dai, R. Z. W., et al. (2018). Association between bacteremia from specific microbes and subsequent diagnosis of colorectal cancer. Gastroenterology 155 (2), 383–390.e388. doi: 10.1053/j.gastro.2018.04.028
Larabi, A., Barnich, N., Nguyen, H. T. T. (2020). New insights into the interplay between autophagy, gut microbiota and inflammatory responses in IBD. Autophagy 16 (1), 38–51. doi: 10.1080/15548627.2019.1635384
Letchumanan, G., Abdullah, N., Marlini, M., Baharom, N., Lawley, B., Omar, M. R., et al. (2022). Gut microbiota composition in prediabetes and newly diagnosed type 2 diabetes: A systematic review of observational studies. Front. Cell Infect. Microbiol. 12. doi: 10.3389/fcimb.2022.943427
Li, S., Li, N., Wang, C., Zhao, Y., Cao, J., Li, X., et al. (2022). Gut microbiota and immune modulatory properties of human breast milk streptococcus salivarius and s. parasanguinis strains. Front. Nutr. 9. doi: 10.3389/fnut.2022.798403
Li, J., Lu, H., Wu, H., Huang, S., Chen, L., Gui, Q., et al. (2020). Periodontitis in elderly patients with type 2 diabetes mellitus: impact on gut microbiota and systemic inflammation. Aging (Albany NY) 12 (24), 25956–25980. doi: 10.18632/aging.202174
Li, Q., Peng, W., Wu, J., Wang, X., Ren, Y., Li, H., et al. (2019). Autoinducer-2 of gut microbiota, a potential novel marker for human colorectal cancer, is associated with the activation of TNFSF9 signaling in macrophages. Oncoimmunology 8 (10), e1626192. doi: 10.1080/2162402X.2019.1626192
Liu, F., Ma, R., Tay, C. Y. A., Octavia, S., Lan, R., Chung, H. K. L., et al. (2018). Genomic analysis of oral campylobacter concisus strains identified a potential bacterial molecular marker associated with active crohn's disease. Emerg. Microbes Infect. 7 (1), 64. doi: 10.1038/s41426-018-0065-6
Loomba, R., Ling, L., Dinh, D. M., DePaoli, A. M., Lieu, H. D., Harrison, S. A., et al. (2021). The commensal microbe veillonella as a marker for response to an FGF19 analog in NASH. Hepatology 73 (1), 126–143. doi: 10.1002/hep.31523
Lourenvarsigmao, T. G. B., Spencer, S. J., Alm, E. J., Colombo, A. P. V. (2018). Defining the gut microbiota in individuals with periodontal diseases: an exploratory study. J. Oral. Microbiol. 10 (1), 1487741. doi: 10.1080/20002297.2018.1487741
Lu, H., Xu, M., Wang, F., Liu, S., Gu, J., Lin, S., et al. (2016). Chronic stress accelerates ligature-induced periodontitis by suppressing glucocorticoid receptor-alpha signaling. Exp. Mol. Med. 48 (3), e223. doi: 10.1038/emm.2015.127
Maeda, Y., Takeda, K. (2019). Host-microbiota interactions in rheumatoid arthritis. Exp. Mol. Med. 51 (12), 1–6. doi: 10.1038/s12276-019-0283-6
Ma, Q. Y., Huang, D. Y., Zhang, H. J., Wang, S., Chen, X. F. (2017). Upregulation of bacterial-specific Th1 and Th17 responses that are enriched in CXCR5(+)CD4(+) T cells in non-small cell lung cancer. Int. Immunopharmacol 52, 305–309. doi: 10.1016/j.intimp.2017.09.024
Maki, K. A., Kazmi, N., Barb, J. J., Ames, N. (2021). The oral and gut bacterial microbiomes: Similarities, differences, and connections. Biol. Res. Nurs. 23 (1), 7–20. doi: 10.1177/1099800420941606
McPherson, A. C., Pandey, S. P., Bender, M. J., Meisel, M. (2021). Systemic immunoregulatory consequences of gut commensal translocation. Trends Immunol. 42 (2), 137–150. doi: 10.1016/j.it.2020.12.005
Mignolet, J., Fontaine, L., Sass, A., Nannan, C., Mahillon, J., Coenye, T., et al. (2018). Circuitry rewiring directly couples competence to predation in the gut dweller streptococcus salivarius. Cell Rep. 22 (7), 1627–1638. doi: 10.1016/j.celrep.2018.01.055
Moskovitz, M., Nassar, M., Moriel, N., Cher, A., Faibis, S., Ram, D., et al. (2021). Characterization of the oral microbiome among children with type 1 diabetes compared with healthy children. Front. Microbiol. 12. doi: 10.3389/fmicb.2021.756808
Nagao, J. I., Kishikawa, S., Tanaka, H., Toyonaga, K., Narita, Y., Negoro-Yasumatsu, K., et al. (2022). Pathobiont-responsive Th17 cells in gut-mouth axis provoke inflammatory oral disease and are modulated by intestinal microbiome. Cell Rep. 40 (10), 111314. doi: 10.1016/j.celrep.2022.111314
Nagata, N., Nishijima, S., Kojima, Y., Hisada, Y., Imbe, K., Miyoshi-Akiyama, T., et al. (2022). Metagenomic identification of microbial signatures predicting pancreatic cancer from a multinational study. Gastroenterology 163 (1), 222–238. doi: 10.1053/j.gastro.2022.03.054
Nakajima, M., Arimatsu, K., Kato, T., Matsuda, Y., Minagawa, T., Takahashi, N., et al. (2015). Oral administration of p. gingivalis induces dysbiosis of gut microbiota and impaired barrier function leading to dissemination of enterobacteria to the liver. PloS One 10 (7), e0134234. doi: 10.1371/journal.pone.0134234
Nakatsu, G., Zhou, H., Wu, W. K. K., Wong, S. H., Coker, O. O., Dai, Z., et al. (2018). Alterations in enteric virome are associated with colorectal cancer and survival outcomes. Gastroenterology 155 (2), 529–541.e525. doi: 10.1053/j.gastro.2018.04.018
Nishimura, H., Fukui, H., Wang, X., Ebisutani, N., Nakanishi, T., Tomita, T., et al. (2021). Role of the beta-Catenin/REG ialpha axis in the proliferation of sessile serrated Adenoma/Polyps associated with fusobacterium nucleatum. Pathogens 10 (4), 434. doi: 10.3390/pathogens10040434
Ohtsu, A., Takeuchi, Y., Katagiri, S., Suda, W., Maekawa, S., Shiba, T., et al. (2019). Influence of porphyromonas gingivalis in gut microbiota of streptozotocin-induced diabetic mice. Oral. Dis. 25 (3), 868–880. doi: 10.1111/odi.13044
Olsen, I., Yamazaki, K. (2019). Can oral bacteria affect the microbiome of the gut? J. Oral. Microbiol. 11 (1), 1586422. doi: 10.1080/20002297.2019.1586422
Osman, M. A., Neoh, H. M., Ab Mutalib, N. S., Chin, S. F., Mazlan, L., Raja Ali, R. A., et al. (2021). Parvimonas micra, peptostreptococcus stomatis, fusobacterium nucleatum and akkermansia muciniphila as a four-bacteria biomarker panel of colorectal cancer. Sci. Rep. 11 (1), 2925. doi: 10.1038/s41598-021-82465-0
Panpetch, W., Hiengrach, P., Nilgate, S., Tumwasorn, S., Somboonna, N., Wilantho, A., et al. (2020). Additional candida albicans administration enhances the severity of dextran sulfate solution induced colitis mouse model through leaky gut-enhanced systemic inflammation and gut-dysbiosis but attenuated by lactobacillus rhamnosus L34. Gut Microbes 11 (3), 465–480. doi: 10.1080/19490976.2019.1662712
Panpetch, W., Somboonna, N., Palasuk, M., Hiengrach, P., Finkelman, M., Tumwasorn, S., et al. (2019). Oral candida administration in a clostridium difficile mouse model worsens disease severity but is attenuated by bifidobacterium. PloS One 14 (1), e0210798. doi: 10.1371/journal.pone.0210798
Park, S. Y., Hwang, B. O., Lim, M., Ok, S. H., Lee, S. K., Chun, K. S., et al. (2021). Oral-gut microbiome axis in gastrointestinal disease and cancer. Cancers (Basel) 13 (9), 2124. doi: 10.3390/cancers13092124
Petrick, J. L., Wilkinson, J. E., Michaud, D. S., Cai, Q., Gerlovin, H., Signorello, L. B., et al. (2022). The oral microbiome in relation to pancreatic cancer risk in African americans. Br. J. Cancer 126 (2), 287–296. doi: 10.1038/s41416-021-01578-5
Ponath, F., Tawk, C., Zhu, Y., Barquist, L., Faber, F., Vogel, J. (2021). RNA Landscape of the emerging cancer-associated microbe fusobacterium nucleatum. Nat. Microbiol. 6 (8), 1007–1020. doi: 10.1038/s41564-021-00927-7
Prasoodanan, P. K. V., Sharma, A. K., Mahajan, S., Dhakan, D. B., Maji, A., Scaria, J., et al. (2021). Western And non-western gut microbiomes reveal new roles of prevotella in carbohydrate metabolism and mouth-gut axis. NPJ Biofilms Microbiomes 7 (1), 77. doi: 10.1038/s41522-021-00248-x
Prieto, D., Carpena, N., Maneu, V., Gil, M. L., Pla, J., Gozalbo, D. (2016). TLR2 modulates gut colonization and dissemination of candida albicans in a murine model. Microbes Infect. 18 (10), 656–660. doi: 10.1016/j.micinf.2016.05.005
Priya, S., Burns, M. B., Ward, T., Mars, R. A. T., Adamowicz, B., Lock, E. F., et al. (2022). Identification of shared and disease-specific host gene-microbiome associations across human diseases using multi-omic integration. Nat. Microbiol. 7 (6), 780–795. doi: 10.1038/s41564-022-01121-z
Qi, Y., Wu, H. M., Yang, Z., Zhou, Y. F., Jin, L., Yang, M. F., et al. (2022). New insights into the role of oral microbiota dysbiosis in the pathogenesis of inflammatory bowel disease. Dig Dis. Sci. 67 (1), 42–55. doi: 10.1007/s10620-021-06837-2
Raimondi, S., Amaretti, A., Gozzoli, C., Simone, M., Righini, L., Candeliere, F., et al. (2019). Longitudinal survey of fungi in the human gut: ITS profiling, phenotyping, and colonization. Front. Microbiol. 10. doi: 10.3389/fmicb.2019.01575
Rampelli, S., Fabbrini, M., Candela, M., Biagi, E., Brigidi, P., Turroni, S. (2021). G2S: A new deep learning tool for predicting stool microbiome structure from oral microbiome data. Front. Genet. 12. doi: 10.3389/fgene.2021.644516
Rashidi, A., Ebadi, M., Weisdorf, D. J., Costalonga, M., Staley, C. (2021). No evidence for colonization of oral bacteria in the distal gut in healthy adults. Proc. Natl. Acad. Sci. U.S.A. 118 (42), e2114152118. doi: 10.1073/pnas.2114152118
Rengarajan, S., Vivio, E. E., Parkes, M., Peterson, D. A., Roberson, E. D. O., Newberry, R. D., et al. (2020). Dynamic immunoglobulin responses to gut bacteria during inflammatory bowel disease. Gut Microbes 11 (3), 405–420. doi: 10.1080/19490976.2019.1626683
Richardson, M., Ren, J., Rubinstein, M. R., Taylor, J. A., Friedman, R. A., Shen, B., et al. (2020). Analysis of 16S rRNA genes reveals reduced fusobacterial community diversity when translocating from saliva to GI sites. Gut Microbes 12 (1), 1–13. doi: 10.1080/19490976.2020.1814120
Rubinstein, M. R., Wang, X., Liu, W., Hao, Y., Cai, G., Han, Y. W. (2013). Fusobacterium nucleatum promotes colorectal carcinogenesis by modulating e-cadherin/beta-catenin signaling via its FadA adhesin. Cell Host Microbe 14 (2), 195–206. doi: 10.1016/j.chom.2013.07.012
Ruhlemann, M., Liwinski, T., Heinsen, F. A., Bang, C., Zenouzi, R., Kummen, M., et al. (2019). Consistent alterations in faecal microbiomes of patients with primary sclerosing cholangitis independent of associated colitis. Aliment Pharmacol. Ther. 50 (5), 580–589. doi: 10.1111/apt.15375
Saboo, K., Petrakov, N. V., Shamsaddini, A., Fagan, A., Gavis, E. A., Sikaroodi, M., et al. (2022). Stool microbiota are superior to saliva in distinguishing cirrhosis and hepatic encephalopathy using machine learning. J. Hepatol. 76 (3), 600–607. doi: 10.1016/j.jhep.2021.11.011
Sato, K., Takahashi, N., Kato, T., Matsuda, Y., Yokoji, M., Yamada, M., et al. (2017). Aggravation of collagen-induced arthritis by orally administered porphyromonas gingivalis through modulation of the gut microbiota and gut immune system. Sci. Rep. 7 (1), 6955. doi: 10.1038/s41598-017-07196-7
Schmidt, T. S., Hayward, M. R., Coelho, L. P., Li, S. S., Costea, P. I., Voigt, A. Y., et al. (2019). Extensive transmission of microbes along the gastrointestinal tract. Elife 8, e42693. doi: 10.7554/eLife.42693
Sender, R., Fuchs, S., Milo, R. (2016). Revised estimates for the number of human and bacteria cells in the body. PloS Biol. 14 (8), e1002533. doi: 10.1371/journal.pbio.1002533
Sequeira, R. P., McDonald, J. A. K., Marchesi, J. R., Clarke, T. B. (2020). Commensal bacteroidetes protect against klebsiella pneumoniae colonization and transmission through IL-36 signalling. Nat. Microbiol. 5 (2), 304–313. doi: 10.1038/s41564-019-0640-1
Song, W., Sun, L. Y., Zhu, Z. J., Wei, L., Qu, W., Zeng, Z. G., et al. (2021). Association of gut microbiota and metabolites with disease progression in children with biliary atresia. Front. Immunol. 12. doi: 10.3389/fimmu.2021.698900
Stanisic, D., Jovanovic, M., George, A. K., Homme, R. P., Tyagi, N., Singh, M., et al. (2021). Gut microbiota and the periodontal disease: role of hyperhomocysteinemia. Can. J. Physiol. Pharmacol. 99 (1), 9–17. doi: 10.1139/cjpp-2020-0215
Superdock, D., Poole, A. (2022). Processing method affects oral and gut microbiome composition. Curr. Developments Nutr. 6 (Supplement_1), 779–779. doi: 10.1093/cdn/nzac063.021
Tang, X., Tangkham, T., Aljahdali, B., Lee, S., Su, M., Dibart, S. (2021). The role of TNF-alpha induced protein 1 in the activation of pro-apoptotic proteins. Hum. Cell 34 (4), 1123–1129. doi: 10.1007/s13577-021-00529-9
Tan, Q., Ma, X., Yang, B., Liu, Y., Xie, Y., Wang, X., et al. (2022). Periodontitis pathogen porphyromonas gingivalis promotes pancreatic tumorigenesis via neutrophil elastase from tumor-associated neutrophils. Gut Microbes 14 (1), 2073785. doi: 10.1080/19490976.2022.2073785
Tran, H. N. H., Hoang Thu, T. N., Nguyen, P. H., Vo, C. N., Van Doan, K., Ngoc Minh, C. N., et al. (2022). Mucosal microbiomes and fusobacterium genomics in Vietnamese colorectal cancer patients. J. bioRxiv 8 (1), 87. doi: 10.1101/2022.02.25.481918. 2022.2002.2025.481918.
Tripathi, A., Nahar, A., Sharma, R., Kanaskie, T., Al-Hebshi, N., Puri, S. (2022). High iron-mediated increased oral fungal burden, oral-to-gut transmission, and changes to pathogenicity of candida albicans in oropharyngeal candidiasis. J. Oral. Microbiol. 14 (1), 2044110. doi: 10.1080/20002297.2022.2044110
Tsuzuno, T., Takahashi, N., Yamada-Hara, M., Yokoji-Takeuchi, M., Sulijaya, B., Aoki-Nonaka, Y., et al. (2021). Ingestion of porphyromonas gingivalis exacerbates colitis via intestinal epithelial barrier disruption in mice. J. Periodontal Res. 56 (2), 275–288. doi: 10.1111/jre.12816
Vaishnava, S., Behrendt, C. L., Ismail, A. S., Eckmann, L., Hooper, L. V. (2008). Paneth cells directly sense gut commensals and maintain homeostasis at the intestinal host-microbial interface. Proc. Natl. Acad. Sci. U.S.A. 105 (52), 20858–20863. doi: 10.1073/pnas.0808723105
van Leeuwen, P. T., van der Peet, J. M., Bikker, F. J., Hoogenkamp, M. A., Oliveira Paiva, A. M., Kostidis, S., et al. (2016). Interspecies interactions between clostridium difficile and candida albicans. mSphere 1 (6), e00187-16. doi: 10.1128/mSphere.00187-16
Vasapolli, R., Schutte, K., Schulz, C., Vital, M., Schomburg, D., Pieper, D. H., et al. (2019). Analysis of transcriptionally active bacteria throughout the gastrointestinal tract of healthy individuals. Gastroenterology 157 (4), 1081–1092 e1083. doi: 10.1053/j.gastro.2019.05.068
Velsko, I. M., Chukkapalli, S. S., Rivera-Kweh, M. F., Chen, H., Zheng, D., Bhattacharyya, I., et al. (2015). Fusobacterium nucleatum alters atherosclerosis risk factors and enhances inflammatory markers with an atheroprotective immune response in ApoE(null) mice. PloS One 10 (6), e0129795. doi: 10.1371/journal.pone.0129795
Walencik, P. K. (2022). The redox-active Cu-FomA complex: the mode that provides coordination of Cu(II)/Cu(I) ions during the reduction/oxidation cycle. Dalton Trans. 51 (40), 15515–15529. doi: 10.1039/d2dt02398a
Wang, T., Ishikawa, T., Sasaki, M., Chiba, T. (2022). Oral and gut microbial dysbiosis and non-alcoholic fatty liver disease: The central role of porphyromonas gingivalis. Front. Med. (Lausanne) 9. doi: 10.3389/fmed.2022.822190
Wang, X., Jia, Y., Wen, L., Mu, W., Wu, X., Liu, T., et al. (2021a). Porphyromonas gingivalis promotes colorectal carcinoma by activating the hematopoietic NLRP3 inflammasome. Cancer Res. 81 (10), 2745–2759. doi: 10.1158/0008-5472.CAN-20-3827
Wang, Y., Zhang, Y., Qian, Y., Xie, Y. H., Jiang, S. S., Kang, Z. R., et al. (2021b). Alterations in the oral and gut microbiome of colorectal cancer patients and association with host clinical factors. Int. J. Cancer. doi: 10.1002/ijc.33596
Wei, F., Sun, X., Gao, Y., Dou, H., Liu, Y., Su, L., et al. (2021). Is oral microbiome of children able to maintain resistance and functional stability in response to short-term interference of ingesta? Protein Cell 12 (6), 502–510. doi: 10.1007/s13238-020-00774-y
Wirbel, J., Pyl, P. T., Kartal, E., Zych, K., Kashani, A., Milanese, A., et al. (2019). Meta-analysis of fecal metagenomes reveals global microbial signatures that are specific for colorectal cancer. Nat. Med. 25 (4), 679–689. doi: 10.1038/s41591-019-0406-6
Wu, T., Xu, F., Su, C., Li, H., Lv, N., Liu, Y., et al. (2020). Alterations in the gut microbiome and cecal metabolome during klebsiella pneumoniae-induced pneumosepsis. Front. Immunol. 11. doi: 10.3389/fimmu.2020.01331
Wu, F., Yang, L., Hao, Y., Zhou, B., Hu, J., Yang, Y., et al. (2022). Oral and gastric microbiome in relation to gastric intestinal metaplasia. Int. J. Cancer 150 (6), 928–940. doi: 10.1002/ijc.33848
Xiao, Q., Lu, W., Kong, X., Shao, Y. W., Hu, Y., Wang, A., et al. (2021). Alterations of circulating bacterial DNA in colorectal cancer and adenoma: A proof-of-concept study. Cancer Lett. 499, 201–208. doi: 10.1016/j.canlet.2020.11.030
Xia, B., Yang, M., Nguyen, L. H., He, Q., Zhen, J., Yu, Y., et al. (2021). Regular use of proton pump inhibitor and the risk of inflammatory bowel disease: Pooled analysis of 3 prospective cohorts. Gastroenterology 161 (6), 1842–1852.e1810. doi: 10.1053/j.gastro.2021.08.005
Xue, Y., Xiao, H., Guo, S., Xu, B., Liao, Y., Wu, Y., et al. (2018). Indoleamine 2,3-dioxygenase expression regulates the survival and proliferation of fusobacterium nucleatum in THP-1-derived macrophages. Cell Death Dis. 9 (3), 355. doi: 10.1038/s41419-018-0389-0
Xun, Z., Zhang, Q., Xu, T., Chen, N., Chen, F. (2018). Dysbiosis and ecotypes of the salivary microbiome associated with inflammatory bowel diseases and the assistance in diagnosis of diseases using oral bacterial profiles. Front. Microbiol. 9. doi: 10.3389/fmicb.2018.01136
Yamazaki, K., Kato, T., Tsuboi, Y., Miyauchi, E., Suda, W., Sato, K., et al. (2021). Oral pathobiont-induced changes in gut microbiota aggravate the pathology of nonalcoholic fatty liver disease in mice. Front. Immunol. 12. doi: 10.3389/fimmu.2021.766170
Yao, C., Lan, D., Li, X., Wang, Y., Qi, S., Liu, Y. (2022). Porphyromonas gingivalis is a risk factor for the development of nonalcoholic fatty liver disease via ferroptosis. Microbes Infect. 25 (1-2), 105040. doi: 10.1016/j.micinf.2022.105040
Yonezawa, H., Motegi, M., Oishi, A., Hojo, F., Higashi, S., Nozaki, E., et al. (2021). Lantibiotics produced by oral inhabitants as a trigger for dysbiosis of human intestinal microbiota. Int. J. Mol. Sci. 22 (7), 3343. doi: 10.3390/ijms22073343
Yoshihara, T., Kioi, M., Baba, J., Usuda, H., Kessoku, T., Iwaki, M., et al. (2021). A prospective interventional trial on the effect of periodontal treatment on fusobacterium nucleatum abundance in patients with colorectal tumours. Sci. Rep. 11 (1), 23719. doi: 10.1038/s41598-021-03083-4
Young, T. M., Bray, A. S., Nagpal, R. K., Caudell, D. L., Yadav, H., Zafar, M. A. (2020). Animal model to study klebsiella pneumoniae gastrointestinal colonization and host-to-Host transmission. Infect. Immun. 88 (11), e00071-20. doi: 10.1128/IAI.00071-20
Yu, J., Feng, Q., Wong, S. H., Zhang, D., Liang, Q. Y., Qin, Y., et al. (2017). Metagenomic analysis of faecal microbiome as a tool towards targeted non-invasive biomarkers for colorectal cancer. Gut 66 (1), 70–78. doi: 10.1136/gutjnl-2015-309800
Zhang, J., Xie, M., Huang, X., Chen, G., Yin, Y., Lu, X., et al. (2021). The effects of porphyromonas gingivalis on atherosclerosis-related cells. Front. Immunol. 12. doi: 10.3389/fimmu.2021.766560
Zhao, L., Cho, W. C., Nicolls, M. R. (2021). Colorectal cancer-associated microbiome patterns and signatures. Front. Genet. 12. doi: 10.3389/fgene.2021.787176
Keywords: oral microbe, intestinal microbe, gut diseases, microbiota, immunity, oral-gut axis
Citation: Lu Y, Li Z and Peng X (2023) Regulatory effects of oral microbe on intestinal microbiota and the illness. Front. Cell. Infect. Microbiol. 13:1093967. doi: 10.3389/fcimb.2023.1093967
Received: 09 November 2022; Accepted: 16 January 2023;
Published: 01 February 2023.
Edited by:
Feng Chen, Peking University, ChinaReviewed by:
Tomoko Hanawa, Kyorin University, JapanSantosh K. Ghosh, Case Western Reserve University, United States
Copyright © 2023 Lu, Li and Peng. This is an open-access article distributed under the terms of the Creative Commons Attribution License (CC BY). The use, distribution or reproduction in other forums is permitted, provided the original author(s) and the copyright owner(s) are credited and that the original publication in this journal is cited, in accordance with accepted academic practice. No use, distribution or reproduction is permitted which does not comply with these terms.
*Correspondence: Xian Peng, cGVuZ3hAc2N1LmVkdS5jbg==
†These authors have contributed equally to this work and share first authorship