- 1Key Laboratory of Prevention and Control Agents for Animal Bacteriosis (Ministry of Agriculture and Rural Affairs), Hubei Provincial Key Laboratory of Animal Pathogenic Microbiology, Institute of Animal Husbandry and Veterinary Sciences, Hubei Academy of Agricultural Sciences, Wuhan, China
- 2Anhui Province Key Laboratory of Veterinary Pathobiology and Disease Control, College of Animal Science and Technology, Anhui Agricultural University, Hefei, China
- 3Tianjin Key Laboratory of Agricultural Animal Breeding and Healthy Husbandry, College of Animal Science and Veterinary Medicine, Tianjin Agricultural University, Tianjin, China
Mycoplasma hyopneumoniae is a highly contagious pathogen causing porcine enzootic pneumonia, which elicits prolonged inflammatory response modulated by pattern recognition receptors (PRRs). Although significant advances have been achieved in understanding the Toll-Like receptors that recognize M. hyopneumoniae, the role of nucleotide-binding oligomerization domain 1 (NOD1) in M. hyopneumoniae infected cells remains poorly understood. This study revealed that M. hyopneumoniae activates the NOD1-RIP2 pathway and is co-localized with host NOD1 during infection. siRNA knockdown of NOD1 significantly impaired the TRIF and MYD88 pathway and blocked the activation of TNF-α. In contrast, NOD1 overexpression significantly suppressed M. hyopneumoniae proliferation. Furthermore, we for the first time investigated the interaction between M. hyopneumoniae mhp390 and NOD1 receptor, and the results suggested that mhp390 and NOD1 are possibly involved in the recognition of M. hyopneumoniae. These findings may improve our understanding of the interaction between PRRs and M. hyopneumoniae and the function of NOD1 in host defense against M. hyopneumoniae infection.
Introduction
Germline-encoded pattern recognition receptors (PRRs) can sense and rapidly respond to structures derived from distinct bacteria and viruses, which is known as the pathogen-associated molecular patterns (PAMPs) (Takeuchi and Akira, 2010). To date, four main PRR families have been documented, including Toll-like receptors (TLRs), retinoic acid-inducible gene (RIG)-I-like receptors (RLRs), C-type lectin receptors (CLRs) and NOD-like receptors (NLRs) (Walsh et al., 2013). TLRs and RLRs can recognize structurally conserved molecules derived from microbes and play a key role in the innate immune system (Lupfer et al., 2013). NLRs can trigger multiple signaling cascades such as the proinflammatory nuclear factor B (NF-κB) and the inflammasome pathways, as well as autophagy and cell death pathways (Philpott and Girardin, 2010). Nucleotide-binding oligomerization domain 1 (NOD1) and NOD2 are the most extensively studied members of the NLR family, which are responsible for triggering the host immune response (Murillo et al., 2003; Tourlomousis et al., 2020; Yoo et al., 2021).
Notable progress has been made in understanding the critical role of NLRs in eliciting immune responses against bacteria and virus (Moreira and Zamboni, 2012; McKernan, 2020). Previous studies have revealed a critical role of NOD1 in innate immune response and inflammatory regulation during infection by Pseudomonas aeruginosa (Travassos et al., 2005), Streptococcus pneumoniae (Opitz et al., 2004) and other intracellular bacteria such as Shigella flexneri (Girardin et al., 2001) or Listeria monocytogenes (Opitz et al., 2006). More recently, a number of studies have demonstrated that both RNA viruses (from the families of Orthomyxoviridae, Rhabdoviridae, Paramyxioviridae, Flaviviridae, and Picornaviridae) and DNA viruses (from the families of Poxviridae, Adenoviridae, Herpesviridae) can activate the NLRP3 inflammasome pathway (Lupfer and Kanneganti, 2013; da Costa et al., 2019; Boal-Carvalho et al., 2020; Choudhury et al., 2021). Inflammasomes are cytosolic multiprotein oligomers of the innate immune system, which are responsible for activating the inflammatory response. Moreover, NOD1 was shown to participate in the triggering of innate immunity signaling by Hepatitis C virus (Vegna et al., 2016) and be associated with the susceptibility of ducks to Duck Hepatitis A virus (Liang et al., 2021).
Porcine enzootic pneumonia (EP), which is caused by Mycoplasma hyopneumoniae (M. hyopneumoniae, Mhp), leads to persistent dry cough and chronic pneumonia in swine (Liu et al., 2011). M. hyopneumoniae is highly contagious and widely perceived as an important element of the porcine respiratory disease complex (PRDC), which causes significant economic losses to the global swine industry (Minion et al., 2004). This pathogen can destroy the ciliary barrier and cause immune suppression, increasing the possibility of secondary infection and severity of clinical symptoms (Bai et al., 2013; Liu et al., 2017; Nueangphuet et al., 2021). M. hyopneumoniae infection can also stimulate the host signal transduction pathway and induce the release of pro-inflammatory cytokines such as IL-1β, IL-6, IL-8 and TNF-α (Chae, 2016; Eddicks et al., 2021). Highly virulent M. hyopneumoniae strains have a higher proliferation titer in the lungs and induce more severe inflammation than lowly virulent strains (Meyns et al., 2007). TLR2 and TLR4 play crucial roles in mediating the IgA response induced by M. hyopneumoniae (Li et al., 2019). Treatment with anti-porcine TLR2 and TLR6 antibodies blocked the production of TNF-α from M. hyopneumoniae infected macrophages, indicating the involvement of TLR2/TLR6 in the recognition of M. hyopneumoniae (Okusawa et al., 2004). Although research on TLRs has greatly improved our understanding of this pathogen, the contribution of NOD1 to M. hyopneumoniae infection remains largely unknown. Clarification of the interaction between the pathogen and the host NOD1 can help to further elucidate the pathogenic mechanism of M. hyopneumoniae.
This study explored the expression dynamics of NOD1 upon M. hyopneumoniae infection, and revealed that M. hyopneumoniae activates the NOD1-RIP2 pathway to induce inflammatory cytokine production and therefore inhibit M. hyopneumoniae proliferation. We also demonstrated the interaction between M. hyopneumoniae adhesin mhp390 and NOD1 receptor, and the results indicated that they are possibly involved in the recognition of M. hyopneumoniae.
Materials and Methods
Cells, Bacterial Strains, Growth Conditions and Regents
Porcine alveolar macrophages (PAM, swine 3D4/21 cell lines) were cultured in Roswell Park Memorial Institute 1640 Medium (RPMI 1640) supplemented with 10% heat-inactivated fetal bovine serum (FBS), 100 U/mL penicillin, and 100 μg/mL streptomycin at 37°C in a humidified 5% CO2 incubator. The medium used for maintenance of PAMs was RPMI-1640 (Life Technology, USA) supplemented with 2% heated-inactivated FBS, 100 U/mL penicillin, and 100 μg/mL streptomycin. Escherichia coli strains DH5a and BL21 (DE3) were cultured in Luria Bertani (LB) medium supplemented with ampicillin (100 mg/mL) or kanamycin (50 mg/mL) when needed. M. hyopneumoniae strain 168 was isolated from the Er-hua-nian pig with typical clinical and pathogenic characteristics of mycoplasmal pneumonia of swine (Liu et al., 2011), which was kindly provided by Guoqing Shao (Jiangsu Academy of Agricultural Sciences, Nanjing, China). The strain 168 was grown in KM2 cell-free medium (modified Friis’ medium supplemented with 20% pig serum) in a humidified 5% CO2 incubator at 37°C.
Plasmids and Antibodies
The P68 (pET30a-mhp390) and P97 expression plasmids were prepared in our laboratory as described previously (Liu et al., 2019b). The cDNA of NOD1 was cloned from swine PK15 cells and inserted into pCMV-HA vector (Clontech) to generate HA-NOD1 expression plasmid (EcoRI and XhoI restriction sites, primers listed in Table S1), which expressed HA-tagged proteins. The constructed plasmids were verified by nucleotide sequencing analysis. The commercial antibodies used in this study included polyclonal antibody against LC3 purchased from Proteintech (Cat No. 14600-1-AP, China). NOD1 monoclonal antibody was purchased from Santa Cruz (Cat No. sc-398696, USA). Anti-β-actin was obtained from Beyotime (Cat No. AF0003, China). Anti-mhp390 or anti-mhp384 polyclonal antibody was produced in rabbits through immunization with mhp390 or mhp384 protein respectively in our laboratory (Liu et al., 2019b).
Expression and Purification of mhp390 and NOD1
The plasmids pET30a-mhp390 and pET30a-NOD1 (EcoRI and XhoI restriction sites, primers listed in Table S1) were transformed into E. coli BL21 (DE3) to express the recombinant protein. The resultant recombinant protein tagged with 6×His was purified with Ni-NTA agarose (Bio-Rad, Shanghai, China). The concentration of the purified protein was measured by using the BCA Protein Assay Kit (Beyotime, China).
RNA Exaction and Quantitative Real-Time PCR
PAM cells grown in 6-well plates (Corning, Inc., USA) were infected with M. hyopneumoniae (107 Color Change Unit, CCU) or mock-infected. Total RNA was isolated at the indicated time points using the TRIzol reagent (Invitrogen). Then, the RNA was reverse transcribed into cDNA through TransScript One Step RT-PCR SuperMix (Trans, Beijing, China). qRT-PCR was performed using PerfectStart green qPCR super Mix (TransGen Biotech, China) in the LightCycler 96 (Roche Molecular Biochemicals). The PCR conditions were as follows: pre-denaturing at 95°C for 5 min, denaturing at 95°C for 15 s, annealing at 58°C for 30 s, extension at 72°C for 20 s, and a total of 40 cycles of amplification. The fold change in gene expression relative to the normal was calculated using the delta delta cycles to threshold (ΔΔCt) method. Primers (Table S1) were designed with the Primer Express software (version 3.0; Applied Biosystems, CA). The experiments were performed independently for three times.
Transfection
Transient transfection was performed by using Lipofectamine 2000 (Invitrogen). PAM cells were seeded on 6/12-well plates and cultured until the cells reached approximately 70%–80% confluence, and then transfected with the indicated plasmids or siRNA. For each transfection, 3 ng of the HA-NOD1 expression plasmid or 40 pM of siRNA was used. Cell extract was collected at 6, 9, 12, and 24 h post infection (hpi).
siRNA-Mediated Interference of NOD1
The siRNA targeting porcine NOD1 or negative control siRNA was synthesized by GenePharma (China). Knockdown of endogenous NOD1 in PAM cells was carried out by transfection of NOD1 siRNA. Transient transfection of siRNA was performed by using lipofectamine 2000 (Vazyme, China) according to the manufacturer’s instructions. The siRNA sequences used were as follows: si2815-NOD1, sense 5’-CAGACGUUGAAACACUUAUTT-3’, antisense 5’-AUAAGUGUUUCAACGUCUGTT-3’; Negative control, sense 5’-UUCUUCGAACGUGUCACGUTT-3’, antisense 5’-ACGUGACACGUUCGG AGAATT-3’.
Western Blot Assay
Cell extracts were prepared by adding 200 μL 2 × lysis buffer A (LBA) (65 mM Tris–HCl [pH 6.8], 4% sodium dodecyl sulfate, 3% DL-dithiothreitol, and 40% glycerol) and sonicated. The extracts were lysed by boiling in sodium dodecylsulphate (SDS) sample buffer (2% SDS, 60 mM Tris-HCl [pH 6.8], 10% glycerol, 0.001% bromophenolblue, and 0.33% β-mercaptoethanol), and then loaded on acrylamide sodium dodecyl sulphate polyacrylamide (SDS-PAGE) gel. The separated proteins were transferred onto PVDF membranes (Millipore, Billerica, MA). The resultant membranes were blocked with 5% (w/v) dried skim milk in tris-buffered saline containing 0.05% (v/v) Tween 20 (TBST) and incubated with primary antibodies. The antibodies used in western blot assay were anti-NOD1 monoclonal antibody (Santa Cruz, USA), and anti-mhp384 polyclonal antibody (Liu et al., 2019b). β-actin was detected with an anti-beta-actin monoclonal antibody (MAb) (Beyotime, China) to serve as the loading control. Secondary antibodies, horseradish peroxidase-conjugated anti-mouse and anti-rabbit IgG antibody, were used at a dilution of 1:4000, and the protein bands were visualized through enhanced chemiluminescence (ECL) reagents (Thermo, USA) following the manufacturer’s instructions. The signal was acquired by scanning the membranes, while the number of pixels per surface area was quantified by using ImageJ software.
Flow Cytometry Analysis
The role of mhp390 in M. hyopneumoniae adherence was evaluated through flow cytometry. PAM cells were seeded in 24-well plates, and then rMhp390 was incubated with PAM cells at 37°C for 30 min. Each well was washed for 5 times to remove the unbound protein. The bound rMhp390 was labeled green through anti His-tag mouse mAb and goat anti-mouse IgG-FITC. M. hyopneumoniae adhesin P97R1 and BSA served as the positive and negative control, respectively. The fluorescence intensity of PAM cells was evaluated with flow cytometry (BD C6 plus flow cytometer).
For the rMhp390 inhibition assay, M. hyopneumoniae was pre-blocked with two-fold serial dilution of rMhp390, which corresponded to 1.25–10.0 μg/ml of protein. BSA (10 μg/mL) and 1 mL of RPMI 1640 alone were used as the negative and blank controls, respectively. For the rMhp390 antiserum inhibition assay, M. hyopneumoniae was incubated with anti-rMhp390 serum diluted from 1:50 to 1:1000 before infection. The mixed negative serum (unimmunized rabbit serum, 1:50 dilution) was used as the negative control. The cells were evaluated with flow cytometry (BD C6 plus flow cytometer) and the adhesion ability of M. hyopneumoniae was evaluated based on the fluorescence intensity of PAM cells. These experiments were performed independently for three times.
Confocal Microscopy Assay
PAM cells were propagated in RPMI-1640 on microscope coverslips in 6-well plates (Corning, Inc., USA). M. hyopneumoniae was cultured in a 5% CO2 atmosphere at 37°C for 42 h and fluorescently labeled by incubation with 5 μM CFDA-SE at 37°C for 30 min (avoid light). The bacteria were washed three times to remove excess CFDA-SE. PAM cells (106) were treated with fluorescently labeled M. hyopneumoniae (108 CCU) or PBS at 37°C. The cells were washed with PBS for three times and fixed with 4% neutralized paraformaldehyde for 15 min at room temperature. Then, the cells were blocked with 1% (w/v) BSA in PBS (pH 7.4) overnight at 4°C. Endogenous NOD1 was detected by incubation with mouse anti-NOD1 mAb (Santa Cruz, USA) at 37°C for 1 h, and then overlain with Cy3-conjugated affinipure goat anti-mouse IgG (H+L) diluted at 1:100 (Proteintech, China) at 37°C for 1 h. 4,6-Diamidino-2-phenylindole (DAPI) (Beyotime, China) was used to label the cell nuclei. The cells were washed with PBS between each step. Immunofluorescence was detected with a Zeiss LSM900 laser scanning confocal microscope (Zeiss LSM 900 with Airyscan 2, Germany).
Biolayer Interferometry Assay
The binding kinetics of rMhp390 and rNOD1 was monitored with a biolayer interferometry on ForteBio Octet Red96 instrument (ForteBio, USA). rMhp390 was labeled with biotin through LinKine™ biotin labeling kit (Abbkine, China) and immobilized on the streptavidin (SA) biosensors. After equilibrium in PBST (PBS containing 0.02% (v/v) Tween20) for 300 s, association between rMhp390 and rNOD1 was performed for 300 s. Dissociation was carried out in PBST buffer for 600 s. The kinetic dissociation rate constant (KD) was obtained using a 1:1 binding model. The association and dissociation constants were obtained using the DataAnalysisHT software (ForteBio, USA) version 12.0.
Statistical Analysis
Statistical analysis was performed with the two-way analysis of variance test using the GraphPad PRISM software (version 8.4.3 for Windows; www.graphpad.com/) to evaluate the potential differences among different groups. Data were presented as mean ± standard deviation (SD). P-value < 0.05 was considered as significant; P-value < 0.01 was considered as highly significant; P-value < 0.001 was considered as extremely significant; and ns represents no difference.
Results
M. hyopneumoniae Infection Steadily Up-Regulates the Expression of NOD1
PAM cells are highly permissive for M. hyopneumoniae infection (Bai et al., 2013; Liu et al., 2017). To investigate the expression kinetics of NOD1 after challenge with M. hyopneumoniae, PAM cells were infected with M. hyopneumoniae (100 μL, 108 CCU). The infected cells were collected at 0, 6, 12, 24, 36 hpi, and then subjected to western blotting and qRT-PCR using the primers listed in Table S1. As shown in Figure 1A, M. hyopneumoniae infection steadily increased the expression of NOD1 protein to nearly 1.69 folds at 6 hpi. A similar increase in the mRNA expression of NOD1 was observed after M. hyopneumoniae infection, with a maximum level of 4.19 folds at 6 hpi (Figure 1B).
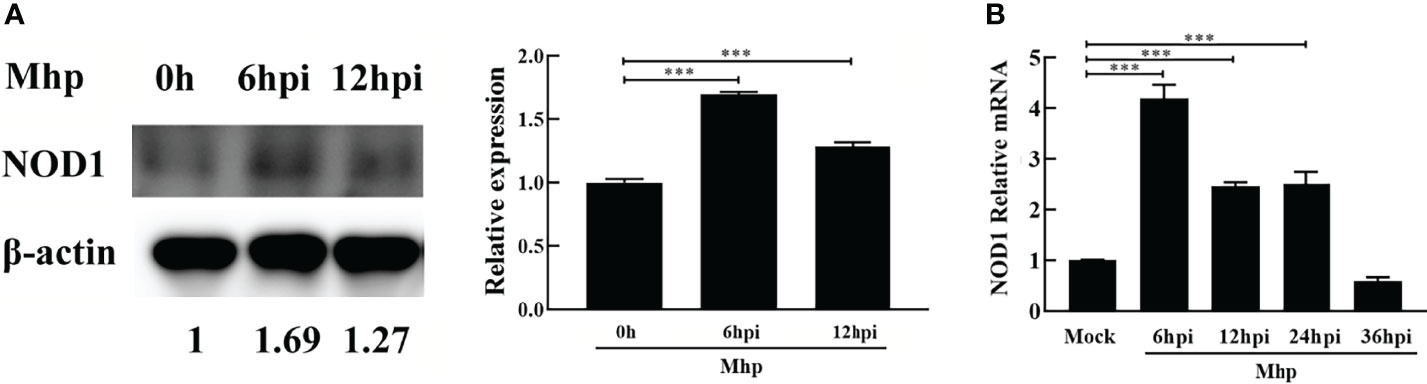
Figure 1 M. hyopneumoniae infection triggers NOD1 transcription and steadily up-regulates NOD1 protein abundance. (A) M. hyopneumoniae infection up-regulates NOD1 protein abundance. (B) M. hyopneumoniae infection triggers NOD1 transcription. ***P < 0.001 indicate statistically significant differences among different groups.
M. hyopneumoniae Employs Host NOD1 to Activate the Innate Immune Signaling Pathway
siRNA-mediated interference was performed to determine whether the silencing of NOD1 affects the M. hyopneumoniae-induced innate immune signal pathway. As shown in Figure 2A, the knockdown efficiency of the NOD1-specific si2815 was over 50% at the protein level, while the mRNA knockdown efficiency of si2815 was about 30% at 24 h post transfection (hpt) (Figure 2B). No obvious interference of NOD1 was found by using si41 or si2684. Thus, NOD1 si2815 was used for further experiments in this study.
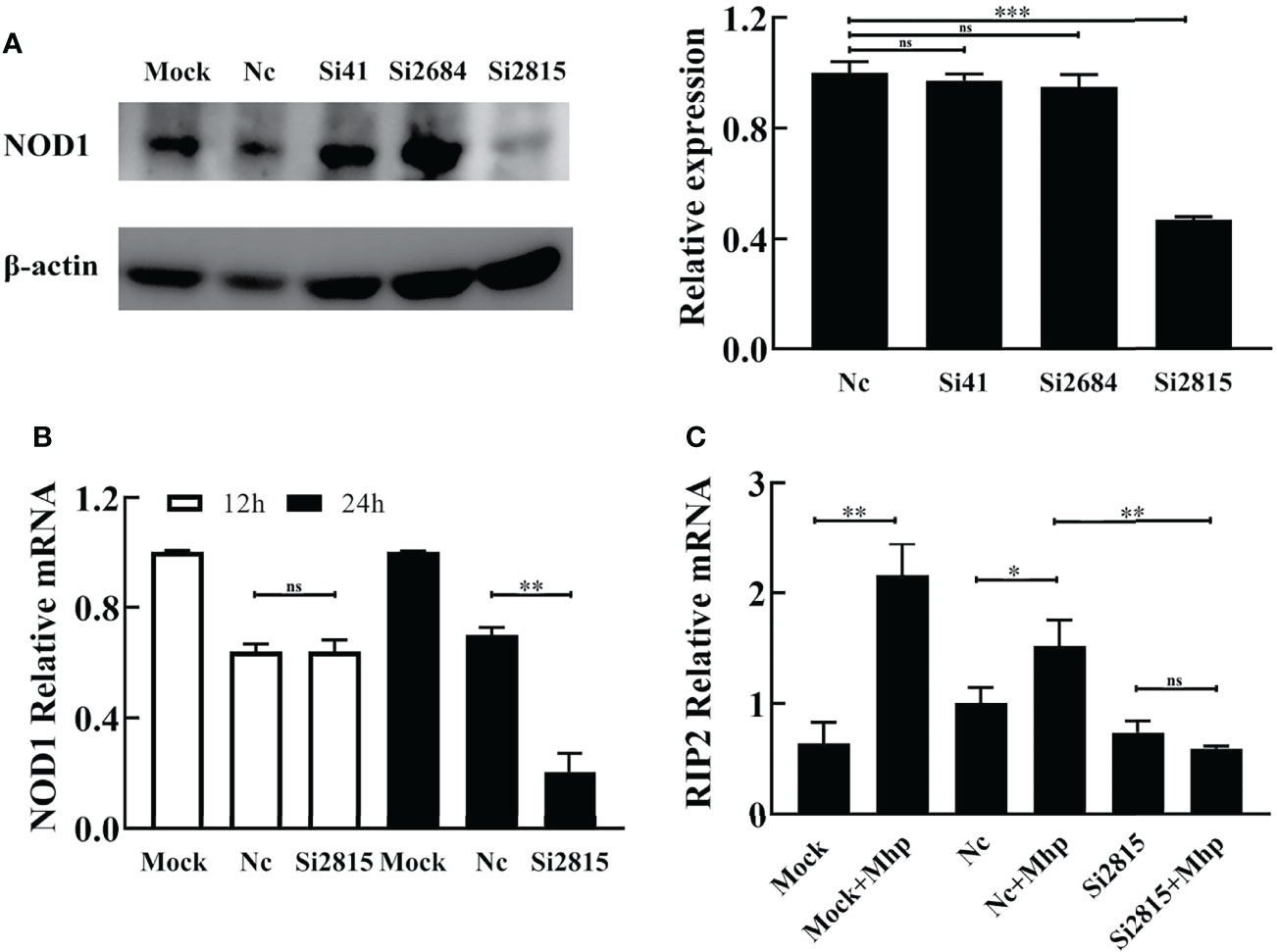
Figure 2 Knockdown of NOD1 inhibits the activation of RIP2 signaling pathway by M. hyopneumoniae. (A) Silencing efficiency of siRNA targeting NOD1. Alveolar macrophages were transfected with the indicated siRNA. The expression of NOD1 was evaluated by western blot at 24 h post transfection. Anti-β-actin was included as a control for sample loading. The relative levels of NOD1 in comparison to those in NC siRNA treated cells are shown as folds in the right panel. (B) The mRNA transcription of NOD1 was measured through real time RT-PCR and compared with that of GAPDH at 12 h/24 h post infection (hpi). (C) Alveolar macrophages were transfected with NOD1 siRNA or NC siRNA for 24 h, and then infected with M. hyopneumoniae (100 μL, 108 CCU). Cells were collected at 6 hpi, and subjected to real-time RT-PCR to determine the expression of RIP2 mRNA. *P < 0.05, **P < 0.01, and ***P < 0.001 indicate statistically significant differences among different groups, and ns represents no difference.
It is well known that the cytosolic receptor NOD1 can sense bacterial peptidoglycan and activate the NF-κB signaling pathway through its adaptor protein, the receptor-interacting protein 2 (RIP2), which will lead to the production of multiple inflammatory cytokines (Jing et al., 2014). Therefore, we investigated whether interference of NOD1 blocks the activation of RIP2 expression in PAM cells upon M. hyopneumoniae infection. As shown in Figure 2C, the mRNA of RIP2 was dramatically decreased in NOD1 siRNA-treated cells compared with that in the NC siRNA cells at 12 hpi.
To study the involvement of the NOD1-mediated innate immune signaling pathway in M. hyopneumoniae infection, we firstly determined the mRNA levels of pro-inflammatory cytokines in PAMs after M. hyopneumoniae infection by qRT-PCR using primers listed in Table S1. As shown in Figures 3A, B, the mRNA expression of both TRIF and MYD88 was significantly up-regulated with a maximum increase at 12 hpi. In addition, M. hyopneumoniae infection remarkably enhanced the mRNA expression of TNF-α (Figure 3C).
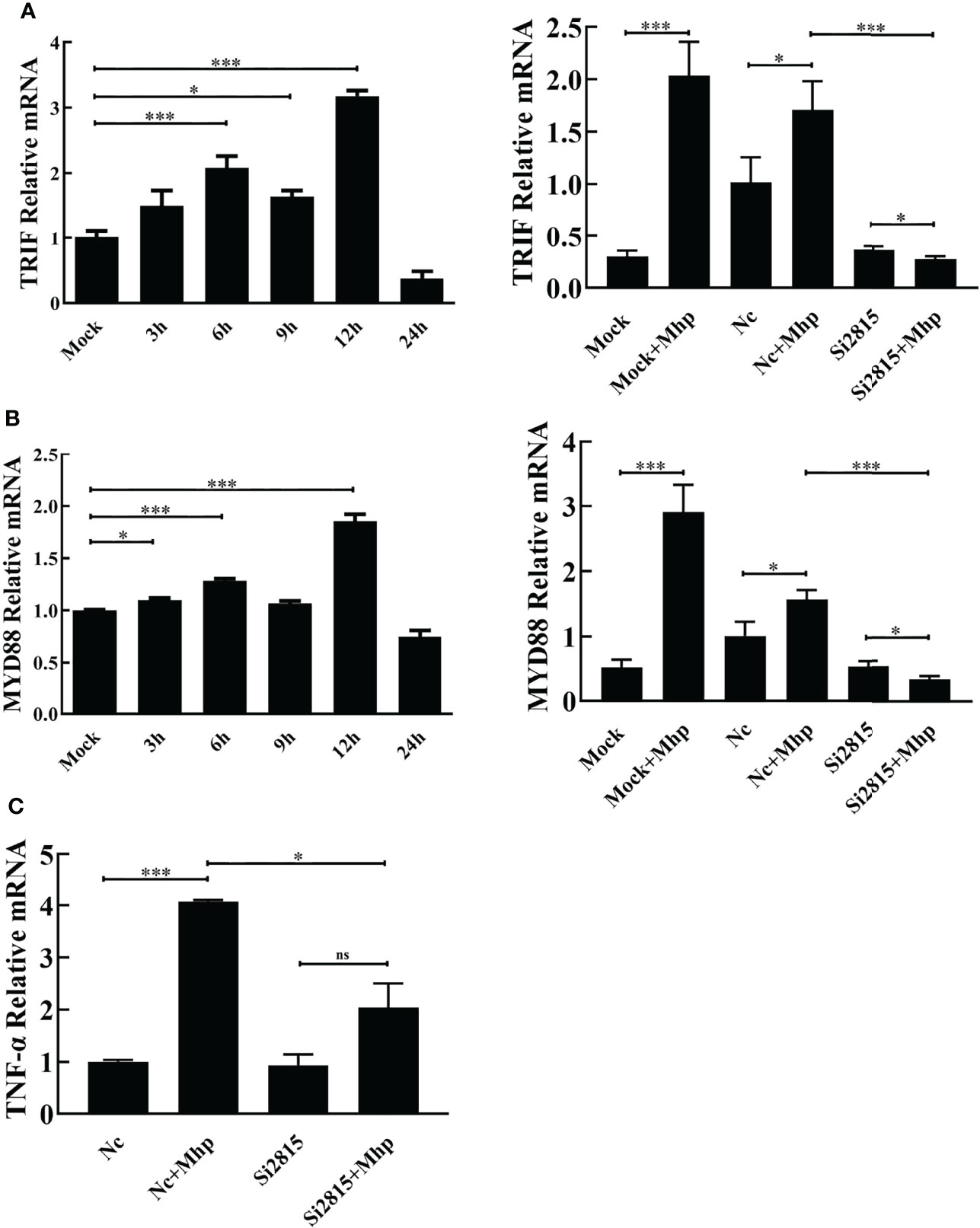
Figure 3 M. hyopneumoniae infection activates NOD1-mediated TRIF and MYD88 signaling pathways. M. hyopneumoniae infection induces TRIF (A) and MYD88 (B) transcription in a time-dependent manner. Alveolar macrophages were infected with M. hyopneumoniae (100 μL, 108 CCU) or mock-infected. Cells were collected separately at the indicated time points, and subjected to qRT-PCR to analyze the relative mRNA transcription. Alveolar macrophages were transfected with NOD1 siRNA or NC siRNA for 24 h, and the cells were then mock infected or infected with M. hyopneumoniae (100 μL, 108 CCU). The cells were collected at 12 hpi. The MYD88 and TRIF mRNA expression levels were determined by qRT-PCR assay. (C) Knockdown of NOD1 blocked M. hyopneumoniae-induced mRNA expression of TNF-α. Transfection and infection experiments performed as described for panels (A, B) The cells were collected at 12 hpi and subjected to qRT-PCR to determine the mRNA expression. *P < 0.05, and ***P < 0.001 indicate statistically significant differences among different groups, and ns represents no difference.
Then, PAM cells were transfected with si2815 or the negative control (NC). At 24 hpt, the transfected cells were stimulated by mock infection or M. hyopneumoniae infection. Furthermore, the mRNA expression of cytokines was determined in M. hyopneumoniae-infected cells. As expected, the mRNA of TRIF, MYD88 and TNF-α significantly decreased in NOD1 si2815 cells compared with that in the NC siRNA cells after M. hyopneumoniae challenge (Figure 3). Taken together, these results suggested that NOD1 silencing blocks the activation of pro-inflammatory cytokines triggered by M. hyopneumoniae.
NOD1 Inhibits the Proliferation of M. hyopneumoniae During Infection
To assess the role of NOD1 in M. hyopneumoniae proliferation, we evaluated the yield of M. hyopneumoniae in PAM cells transfected with hemagglutinin (HA)-NOD1-expressing plasmid (Figure 4A). At 24 hpt, the transfected cells were infected with equal amounts of M. hyopneumoniae. The abundance of mhp384 protein from M. hyopneumoniae, which can indirectly indicate the amount of M. hyopneumoniae, was then determined at 24 hpi. The results demonstrated that overexpression of NOD1 significantly suppressed the proliferation of M. hyopneumoniae compared with the HA infected group (Figure 4B). In contrast, the abundance of mhp384 was slightly increased in NOD1 knockdown cells compared with that in the NC siRNA cells after M. hyopneumoniae challenge (Figure 4C).
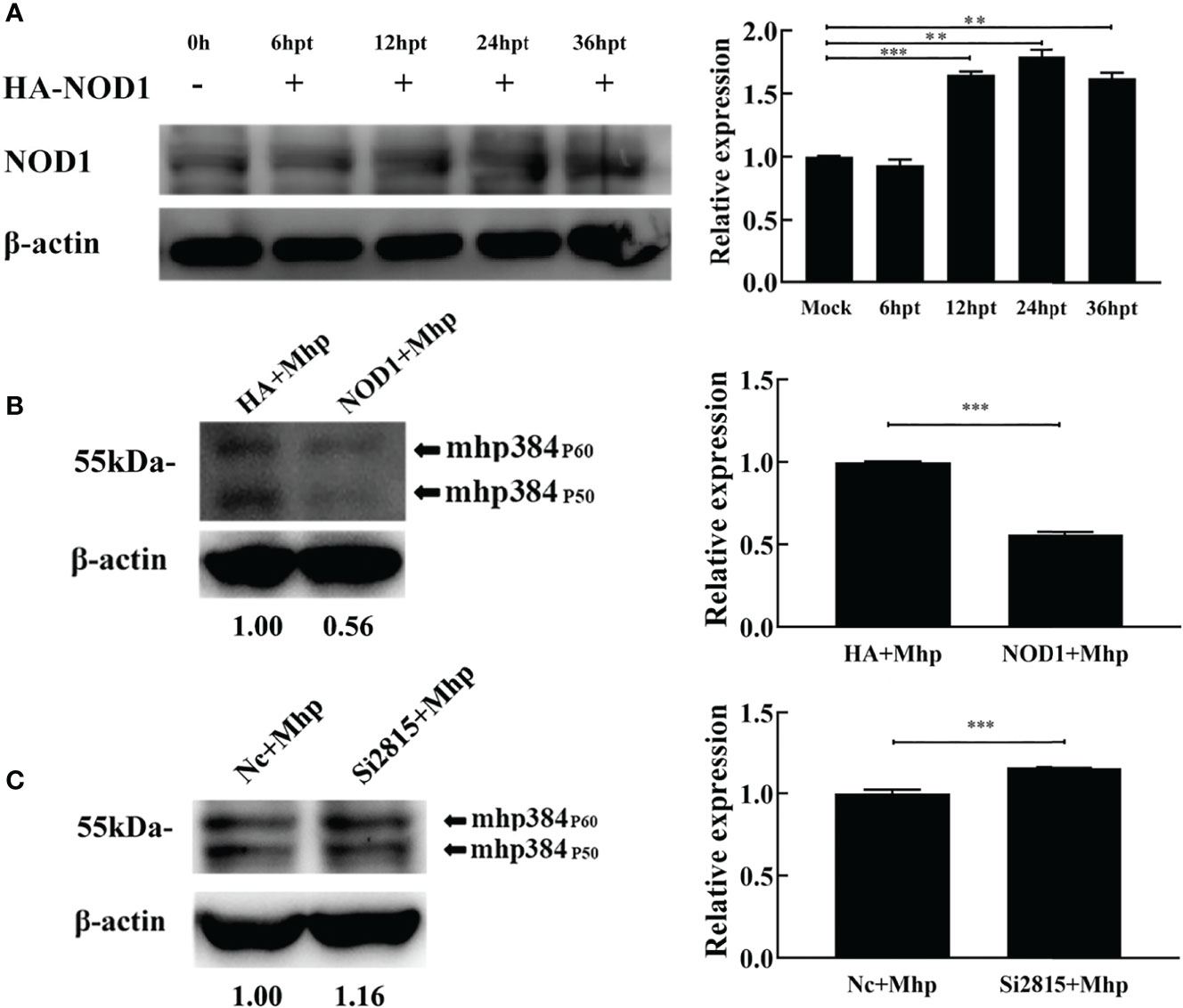
Figure 4 NOD1 inhibits M. hyopneumoniae replication during infection. (A) Alveolar macrophages were transfected with pCMV-HA-NOD1 plasmid or empty pCMV-HA vector. At 0, 6, 12, 24, or 36 hpt, the cells were lysed and subjected to western blotting analysis. Relative expression levels of NOD1 are presented as folds in the right panel. (B) Cells were transfected with pCMV-HA-NOD1 or pCMV-HA for 24 h, and then infected with M. hyopneumoniae (100 μL, 108 CCU). (C) Cells were transfected with Nc siRNA or si2815 for 24 h, and then infected with M. hyopneumoniae (100 μL, 108 CCU). Cells were collected at 24 hpi, and subjected to western blotting to determine the expression of M. hyopneumoniae mhp384. **P < 0.01, and ***P < 0.001 indicate statistically significant differences among different groups.
M. hyopneumoniae Adheres to Alveolar Macrophages and is Co-Localized With NOD1
The adhesion of M. hyopneumoniae to alveolar macrophages was investigated through fluorescence microscopy. Briefly, the host NOD1 was stained red by mouse anti-NOD1 mAb and goat anti-mouse IgG-Cy3, while the M. hyopneumoniae cells were labeled with CFDA-SE (green). As shown in Figure 5, M. hyopneumoniae infection up-regulated the expression of NOD1 compared with the mock infection control. M. hyopneumoniae cells were adhered and ingested into the alveolar macrophages and localized to regions enriched in NOD1, as indicated by the merged yellow signal. These observations suggested an intimate association between M. hyopneumoniae and host NOD1.
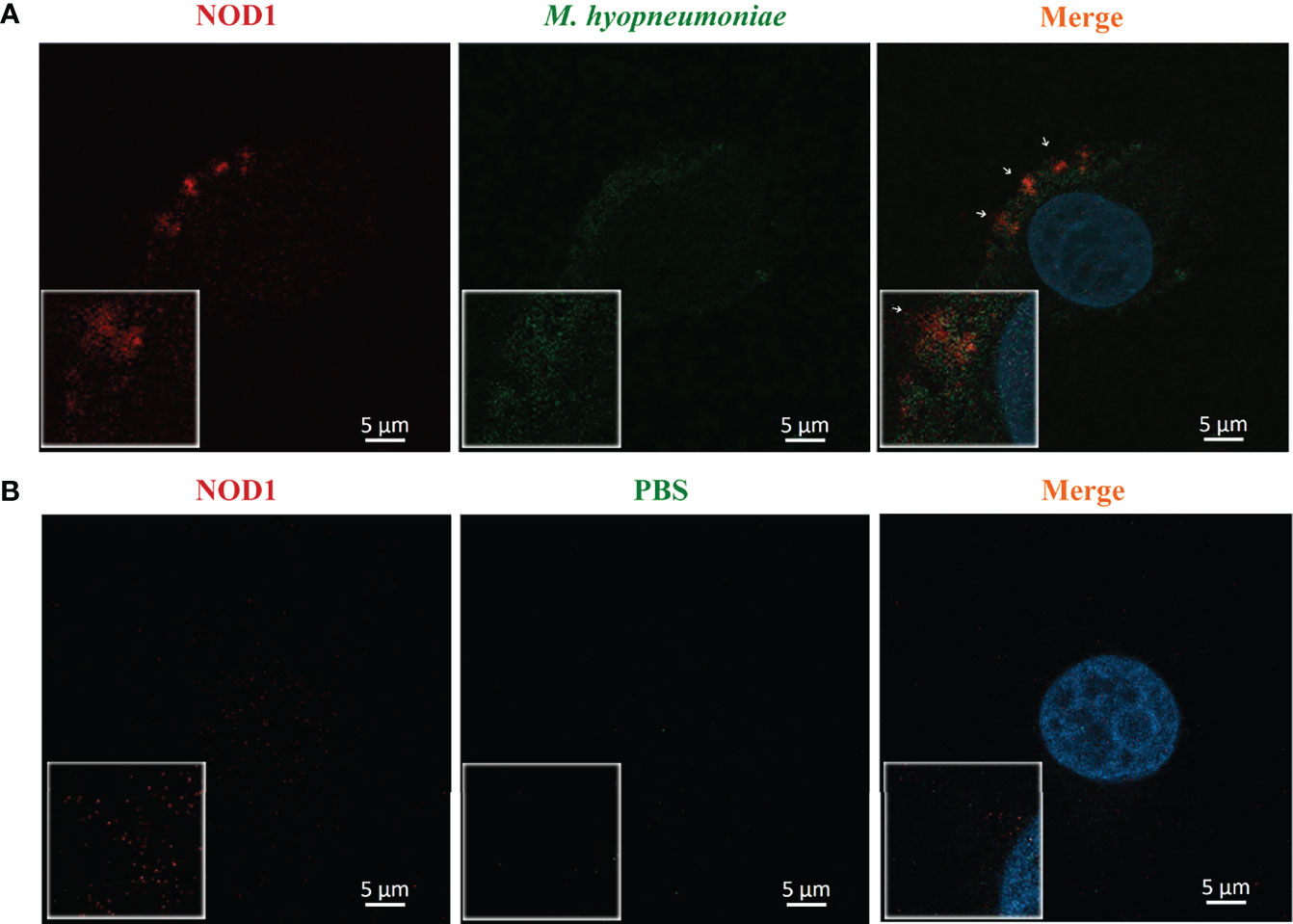
Figure 5 Confocal laser scanning micrograph for the adhesion of M. hyopneumoniae cells to alveolar macrophages. Alveolar macrophages were treated with M. hyopneumoniae (A) and PBS (B), respectively. M. hyopneumoniae cells were labeled with CFDA-SE (green); cell nuclei were stained with DAPI (blue); and NOD1 was labeled red by using mouse anti-NOD1 mAb and goat anti-mouse IgG-Cy3. M. hyopneumoniae cells were adhered to the edges of alveolar macrophages, presenting a merged yellow signal, where M. hyopneumoniae was co-localized with the cellular NOD1 (white arrows). Scale bars = 5 µm.
mhp390 (P68) Mediates Cell Adhesion
The binding ability of mhp390 to alveolar macrophages was determined by using flow cytometry. Briefly, PAM cells were seeded in 24-well plates, and then infected with rMhp390. The unbound protein was removed, and the bound rMhp390 was labeled green through anti His-tag mouse mAb and goat anti-mouse IgG-FITC. M. hyopneumoniae adhesin P97R1 and BSA were used as positive and negative control, respectively. The results demonstrated that mhp390 has a strong adhesive capacity to alveolar macrophages (Figure 6A). In the positive control group, P97R1 showed a 3.65-fold adherence activity relative that of the mock group.
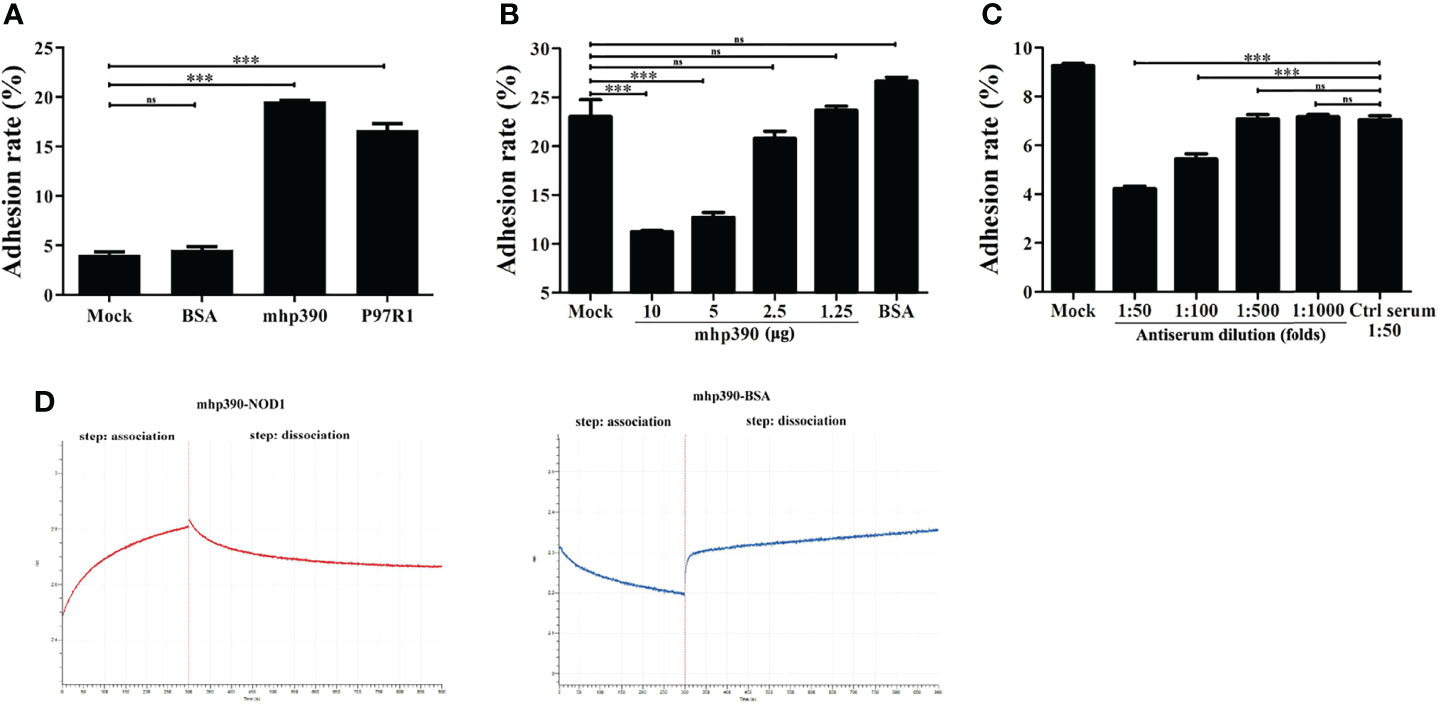
Figure 6 mhp390 mediates M. hyopneumoniae adhesion to alveolar macrophages and interacts with NOD1. (A) Adhesion of rMhp390 to alveolar macrophages detected by flow cytometry. (B) The adhesion of M. hyopneumoniae to alveolar macrophages was inhibited by rMhp390. The cells were incubated with different concentrations of rMhp390 before infection. BSA (10 μg/mL) and 1 mL of RPMI 1640 alone were used as the negative and blank controls, respectively. (C) The adhesion of M. hyopneumoniae to alveolar macrophages was inhibited by anti-rMhp390 serum. M. hyopneumoniae was incubated with anti-rMhp390 serum diluted from 1:50 to 1:1000 before infection. The mixed negative serum (unimmunized rabbit serum, 1:50 dilution) was used as the negative control. ***P < 0.001 indicate statistically significant differences among different groups, and ns represents no difference. (D) Kinetic analysis of the affinity between mhp390 and NOD1 through bio-layer interferometry.
We further investigated the role of mhp390 in M. hyopneumoniae adhesion through flow cytometry. The adhesion of M. hyopneumoniae to PAM cells was first tested. Briefly, M. hyopneumoniae was cultured in a 5% CO2 atmosphere at 37°C for 48 h and fluorescently labeled by incubation with CFDA-SE. Then, the bacteria were washed to remove excess CFDA-SE. PAM cells (106) were infected with fluorescently labeled M. hyopneumoniae at 37°C for 30 min at a multiplicity of infection (MOI) of 1:100. The cells were evaluated with flow cytometry and the adhesion ability of M. hyopneumoniae was evaluated based on the fluorescence intensity of PAM cells.
Subsequently, the inhibitory effect of both rMhp390 and antiserum on the adhesion of M. hyopneumoniae to PAM cells was assayed. To check the binding inhibition effect of rMhp390, M. hyopneumoniae was pre-blocked with two-fold serial dilution of rMhp390. The adhesion rate between the M. hyopneumoniae and alveolar macrophages was determined. With increasing rMhp390 concentration, more significant and dose-dependent inhibition of the binding between M. hyopneumoniae and alveolar macrophage was observed (Figure 6B). In contrast, no significant inhibition effect was found in the BSA and mock groups. As shown in Figure 6C, the binding of M. hyopneumoniae to PAM cells was effectively inhibited by antiserum directed against rMhp390 in a dose-dependent manner, whereas the negative serum from mock-immunized rabbit did not affect M. hyopneumoniae binding.
mhp390 (P68) Interacts With NOD1
To gain more insights into the mechanism for the activation of NOD1 signaling by M. hyopneumoniae, we immobilized rMhp390 on biosensors and analyzed its interaction with rNOD1 through biolayer interferometry (BLI, Octet. RED 96e). As shown in the association and dissociation kinetic chart (Figure 6D), rNOD1 was rapidly bound to but slowly dissociated from SA-biosensors coated with rMhp390. rMhp390 bound rNOD1 with a high affinity (KD = 8.14 μM). In the negative control group, no interaction was observed, with no value of KD.
To determine whether NOD1 plays a role in autophagy induction upon M. hyopneumoniae challenge, PAM cells were transfected with NOD1 siRNA or NC negative control siRNA before M. hyopneumoniae infection. Cell lysates were incubated with anti-LC3 antibody and analyzed through Western blotting. The LC3II/I ratio was determined by using ImageJ. As shown in Figure S1, M. hyopneumoniae activated the autophagy of PAM cells, whereas NOD1 silencing did not impede the autophagy induction. The results suggested that M. hyopneumoniae may induce autophagy through some other pathways instead of the NOD1 signaling pathway.
Discussion
It has been widely recognized that NLRs sense PAMPs and play a crucial role in host immune response against viral and bacterial infection (Kumar et al., 2013; Oviedo-Boyso et al., 2014). In this study, we for the first time investigated the role of NOD1 in M. hyopneumoniae infection and provided evidence that M. hyopneumoniae activates the NOD1-RIP2 pathway to induce pro-inflammatory cytokine production.
Interstitial pneumonia is one of the most obvious clinical symptoms of M. hyopneumoniae infection, indicating that inflammatory response plays a critical role in the pathogenesis of M. hyopneumoniae (Lee et al., 2021). During the colonization of M. hyopneumoniae in the lower respiratory tract, a strong inflammatory response would be stimulated by inducing macrophages to release high levels of cytokines such as TNF-α, IL-1, IL-6, and IL-8 (Damte et al., 2011; Hwang et al., 2011). Large amounts of pro-inflammatory cytokines and high cytotoxic and phagocytic activities of macrophages are responsible for the development of lung tissue injury (Garcia-Morante et al., 2016). These innate immune responses can only be activated when the innate immune system can recognize the invading Mycoplasma through particular receptor-ligand interaction. Previous studies have documented that a heterodimer derived from TLR2 and TLR6 can recognize bacterial lipoproteins and plays an important role in sensing Mycoplasma (Takeda et al., 2002; Muneta et al., 2003; Takeuchi and Akira, 2007). It has been reported that the TLR1/TLR6 heterodimer is involved in sensing the dipalmitoylated lipoproteins from M. pneumonia (Shimizu et al., 2005). M. pneumoniae can induce inflammatory responses through TLR4 and mediate the toxin-dependent activation of inflammasome through several NLRs such as NLRP3 (Shimizu, 2016), resulting in the release of IL-18 and IL-1β (Khare et al., 2012; Segovia et al., 2018). TLR2 and TLR6 are also important in the recognition of M. hyopneumoniae, and blocking of these receptors would reduce the production of TNF-α in porcine alveolar macrophages (Okusawa et al., 2004).
Interestingly, the above inflammatory responses and the release of cytokines induced by M. hyopneumoniae are all dependent on TLR receptors. The results of the present study provide new insights into the mechanism for M. hyopneumoniae to activate inflammatory response through the NOD1 receptor (Figure 7). Besides, it is worth noting that M. hyopneumoniae should utilize some of its own proteins to interact with the NOD1 receptor to activate the subsequent signal cascade. These interesting issues have been studied by our laboratory. The results of NOD1 expression kinetics revealed that M. hyopneumoniae infection could increase the abundance of NOD1 in the host at both protein and mRNA levels. By confocal laser scanning microscopy, we found that M. hyopneumoniae cells were adhered to the edges of alveolar macrophages and co-localized with cellular NOD1. The role of NOD1 in the triggering of inflammatory responses by M. hyopneumoniae was also assessed. NOD1 silencing blocked the activation of pro-inflammatory cytokines triggered by M. hyopneumoniae, such as TRIF, MYD88, and TNF-α. The importance of the NOD-RIP2 pathway in accelerating secondary bacterial infection has been reported for several viruses (Vissers et al., 2012). It has been documented that murine norovirus-1 activates NOD1 and NOD2 signaling, which can accelerate the secondary E. coli infection (Kim et al., 2011). Besides, porcine reproductive and respiratory syndrome virus (PRRSV) infection can augment the NOD2-RIP2 signaling pathway to induce pro-inflammatory response. M. hyopneumoniae frequently co-infects the host with PRRSV, Pasteurella multocida, Actinobacilus pleuropneumoniae, and Haemophilus parasuis, contributing to the respiratory outbreak (Weissenbacher-Lang et al., 2016; Saade et al., 2020). Since NOD receptors can sense foreign pathogens and are responsible for triggering host inflammatory response, the pro-inflammatory cytokines mass produced via these pathways might result in more severe inflammation and expedite the development of lung lesions.
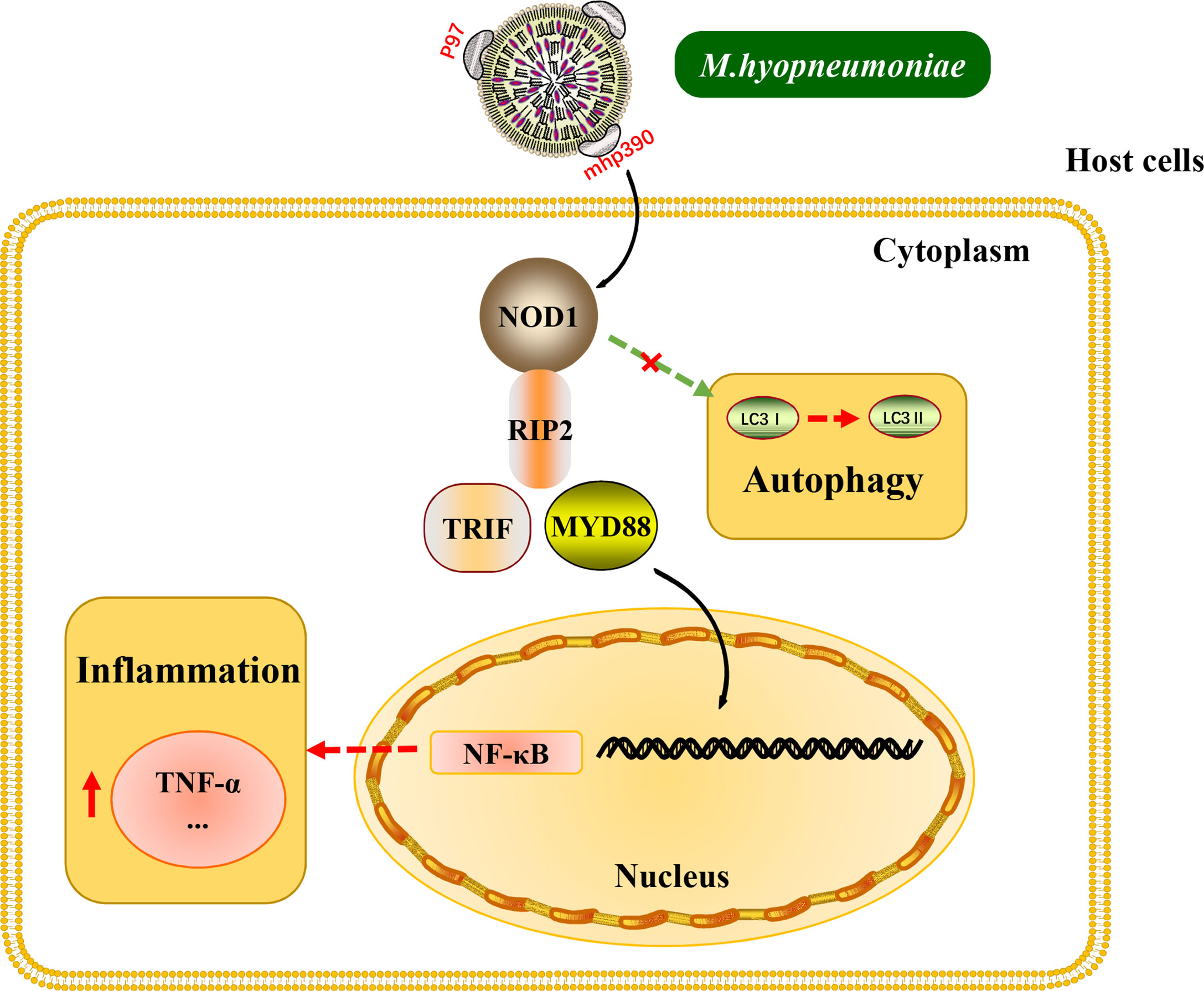
Figure 7 Schematic representation of the NOD1-RIP2 signaling pathway in M. hyopneumoniae invasion of alveolar macrophages.
NOD receptors have been reported to play a variety role in the replication of different viruses. For instance, NOD1 activation is known to up-regulate antiviral response and suppress human cytomegalovirus replication (Fan et al., 2016). Moreover, NOD2 can inhibit the replication of respiratory syncytial virus, influenza virus and foot-and-mouth disease virus, but facilitates that of coxsackievirus B3 (Vissers et al., 2012; Le Bel and Gosselin, 2015; Liu et al., 2019a). Interestingly, we found that NOD1 had antibacterial activity upon M. hyopneumoniae infection. Interference of NOD1 blocked the activation of proinflammatory cytokines, whereas NOD1 overexpression significantly suppressed M. hyopneumoniae proliferation. We speculate that this antibacterial activity may be ascribed to the activation of inflammatory responses by NOD1 signaling.
M. hyopneumoniae mhp390 is a multifunctional protein (Liu et al., 2019b). Our previous study has shown that it is a new cilia adhesin with a strong binding ability for porcine respiratory cilia. Also, mhp390 could induce significant apoptosis of host immune cells. In addition, mhp390 stimulation was found to result in high levels of pro-inflammatory cytokines (Liu et al., 2019b). In this study, M. hyopneumoniae was observed to bind to alveolar macrophages and be co-localized with NOD1. The flow cytometry data confirmed that mhp390 mediates the adhesion of M. hyopneumoniae cells to alveolar macrophages. Furthermore, a strong interaction was found between host NOD1 and mhp390. Taken together, these findings suggest that mhp390 plays a crucial role in the interaction between M. hyopneumoniae and host cells. However, the specific domain responsible for this interaction remains unknown and needs further investigation.
In conclusion, our results demonstrate that M. hyopneumoniae activates the NOD1-RIP2 pathway and is co-localized with host NOD1 during infection. M. hyopneumoniae also employs NOD1 to induce pro-inflammatory response by influencing the TRIF and MYD88 pathway, thereby resulting in enhanced antibacterial activity of the host. Moreover, we for the first time described the interaction between M. hyopneumoniae mhp390 and NOD1 receptor, and the results indicated that mhp390 and NOD1 are possibly involved in the recognition of M. hyopneumoniae. These findings enrich our understanding of the crosstalk between pattern recognition receptors and M. hyopneumoniae and the cellular antibacterial immunity against M. hyopneumoniae.
Data Availability Statement
The original contributions presented in the study are included in the article/Supplementary Material. Further inquiries can be directed to the corresponding authors.
Author Contributions
WL, PS and YT conceived and designed the experiments. PJ, KY, FY, CL and ZL performed the experiments. WL, TG, DZ and QS analyzed the data. WL wrote the paper. All authors contributed to the article and approved the submitted version.
Funding
This work was supported by The National Natural Sciences Foundation of China (31502069), Hubei Province Innovation Center of Agricultural Sciences and Technology (2019-620-000-001-17), Natural Science Foundation of Anhui (N0.1708085MC83), Natural Science Foundation of Tianjin (19JCQNJC13700), Research Project of Tianjin Education Commission (2018KJ185).
Conflict of Interest
The authors declare that the research was conducted in the absence of any commercial or financial relationships that could be construed as a potential conflict of interest.
Publisher’s Note
All claims expressed in this article are solely those of the authors and do not necessarily represent those of their affiliated organizations, or those of the publisher, the editors and the reviewers. Any product that may be evaluated in this article, or claim that may be made by its manufacturer, is not guaranteed or endorsed by the publisher.
Acknowledgments
We would like to thank Prof. Guoqing Shao for kindly providing the M. hyopneumoniae strain 168.
Supplementary Material
The Supplementary Material for this article can be found online at: https://www.frontiersin.org/articles/10.3389/fcimb.2022.927840/full#supplementary-material
Supplementary Figure 1 | NOD1 may not participate in the induction of autophagy by M. hyopneumoniae. (A) Alveolar macrophages were transfected with NOD1 siRNA or NC siRNA for 24 h, and the cells were then mock infected or infected with M. hyopneumoniae (100 μL, 109 CCU). The cells were collected at 9 hpi and subjected to Western blotting by using anti-LC3 polyclonal antibody. (B) The LC3II/I ratio is shown as folds in the right panel.
Supplementary Table 1 | Oligonucleotide primer sequences used in this study for gene amplification and qRT-PCR.
References
Bai, F., Ni, B., Liu, M., Feng, Z., Xiong, Q., Xiao, S., et al. (2013). Mycoplasma Hyopneumoniae-Derived Lipid-Associated Membrane Proteins Induce Apoptosis in Porcine Alveolar Macrophage via Increasing Nitric Oxide Production, Oxidative Stress, and Caspase-3 Activation. Vet. Immunol. Immunopathol. 155 (3), 155–161. doi: 10.1016/j.vetimm.2013.07.004
Boal-Carvalho, I., Mazel-Sanchez, B., Silva, F., Garnier, L., Yildiz, S., Bonifacio, J. P., et al. (2020). Influenza A Viruses Limit NLRP3-NEK7-Complex Formation and Pyroptosis in Human Macrophages. EMBO Rep. 21 (12), e50421. doi: 10.15252/embr.202050421
Chae, C. (2016). Porcine Respiratory Disease Complex: Interaction of Vaccination and Porcine Circovirus Type 2, Porcine Reproductive and Respiratory Syndrome Virus, and Mycoplasma Hyopneumoniae. Vet. J. 212, 1–6. doi: 10.1016/j.tvjl.2015.10.030
Choudhury, S. M., Ma, X., Abdullah, S. W., Zheng, H. (2021). Activation and Inhibition of the NLRP3 Inflammasome by RNA Viruses. J. Inflamm. Res. 14, 1145–1163. doi: 10.2147/JIR.S295706
da Costa, L. S., Outlioua, A., Anginot, A., Akarid, K., Arnoult, D. (2019). RNA Viruses Promote Activation of the NLRP3 Inflammasome Through Cytopathogenic Effect-Induced Potassium Efflux. Cell Death Dis. 10 (5), 346. doi: 10.1038/s41419-019-1579-0
Damte, D., Lee, S. J., Hwang, M. H., Gebru, E., Choi, M. J., Lee, J. S., et al. (2011). Inflammatory Responses to Mycoplasma Hyopneumoniae in Murine Alveolar Macrophage Cell Lines. N. Z. Vet. J. 59 (4), 185–190. doi: 10.1080/00480169.2011.579553
Eddicks, M., Eddicks, L., Stadler, J., Hermanns, W., Ritzmann, M. (2021). The Porcine Respiratory Disease Complex (PRDC) - A Clinical Review. Tierarztl. Prax. Ausg. G Grosstiere Nutztiere 49 (2), 120–132. doi: 10.1055/a-1403-1976
Fan, Y. H., Roy, S., Mukhopadhyay, R., Kapoor, A., Duggal, P., Wojcik, G. L., et al. (2016). Role of Nucleotide-Binding Oligomerization Domain 1 (NOD1) and its Variants in Human Cytomegalovirus Control In Vitro and In Vivo. Proc. Natl. Acad. Sci. U. S. A. 113 (48), E7818–E7827. doi: 10.1073/pnas.1611711113
Garcia-Morante, B., Segales, J., Lopez-Soria, S., de Rozas, A. P., Maiti, H., Coll, T., et al. (2016). Induction of Mycoplasmal Pneumonia in Experimentally Infected Pigs by Means of Different Inoculation Routes. Vet. Res. 47 (1), 54. doi: 10.1186/s13567-016-0340-2
Girardin, S. E., Tournebize, R., Mavris, M., Page, A. L., Li, X., Stark, G. R., et al. (2001). CARD4/Nod1 Mediates NF-kappaB and JNK Activation by Invasive Shigella Flexneri. EMBO Rep. 2 (8), 736–742. doi: 10.1093/embo-reports/kve155
Hwang, M. H., Damte, D., Lee, J. S., Gebru, E., Chang, Z. Q., Cheng, H., et al. (2011). Mycoplasma Hyopneumoniae Induces Pro-Inflammatory Cytokine and Nitric Oxide Production Through NFkappaB and MAPK Pathways in RAW264.7 Cells. Vet. Res. Commun. 35 (1), 21–34. doi: 10.1007/s11259-010-9447-5
Jing, H., Fang, L., Wang, D., Ding, Z., Luo, R., Chen, H., et al. (2014). Porcine Reproductive and Respiratory Syndrome Virus Infection Activates NOD2-RIP2 Signal Pathway in MARC-145 Cells. Virology 458-459, 162–171. doi: 10.1016/j.virol.2014.04.031
Khare, S., Dorfleutner, A., Bryan, N. B., Yun, C., Radian, A. D., de Almeida, L., et al. (2012). An NLRP7-Containing Inflammasome Mediates Recognition of Microbial Lipopeptides in Human Macrophages. Immunity 36 (3), 464–476. doi: 10.1016/j.immuni.2012.02.001
Kim, Y. G., Park, J. H., Reimer, T., Baker, D. P., Kawai, T., Kumar, H., et al. (2011). Viral Infection Augments Nod1/2 Signaling to Potentiate Lethality Associated With Secondary Bacterial Infections. Cell Host Microbe 9 (6), 496–507. doi: 10.1016/j.chom.2011.05.006
Kumar, S., Ingle, H., Prasad, D. V., Kumar, H. (2013). Recognition of Bacterial Infection by Innate Immune Sensors. Crit. Rev. Microbiol. 39 (3), 229–246. doi: 10.3109/1040841X.2012.706249
Le Bel, M., Gosselin, J. (2015). Leukotriene B4 Enhances NOD2-Dependent Innate Response Against Influenza Virus Infection. PloS One 10 (10), e0139856. doi: 10.1371/journal.pone.0139856
Lee, J. Y., Park, K. H., Oh, T., Yang, S., Suh, J., Ham, H. J., et al. (2021). Experimental Reproduction of Porcine Respiratory Disease Complex in Pigs Inoculated Porcine Reproductive and Respiratory Syndrome Virus and Mycoplasma Hyopneumoniae and Followed by Inoculation With Porcine Circovirus Type 2. J. Vet. Med. Sci. 83 (3), 427–430. doi: 10.1292/jvms.20-0577
Li, X., Zhang, Y. K., Yin, B., Liang, J. B., Jiang, F., Wu, W. X. (2019). Toll-Like Receptor 2 (TLR2) and TLR4 Mediate the IgA Immune Response Induced by Mycoplasma Hyopneumoniae. Infect. Immun. 88 (1), e00697–19. doi: 10.1128/IAI.00697-19
Liang, S., Wang, M. S., Zhang, B., Feng, Y., Tang, J., Xie, M., et al (2021). NOD1Is Associated With the Susceptibility of Pekin Duck Flock to Duck Hepatitis A Virus Genotype 3. Front. Immunol. 12. doi: 10.3389/fimmu.2021.766740
Liu, M., Du, G., Liu, B., Hu, Y., Liu, J., Jia, Y., et al. (2017). Cholesterol Exacerbates Mycoplasma Hyopneumoniae-Induced Apoptosis via Stimulating Proliferation and Adhesion to Porcine Alveolar Macrophages. Vet. Microbiol. 211, 112–118. doi: 10.1016/j.vetmic.2017.10.007
Liu, W., Feng, Z., Fang, L., Zhou, Z., Li, Q., Li, S., et al. (2011). Complete Genome Sequence of Mycoplasma Hyopneumoniae Strain 168. J. Bacteriol. 193 (4), 1016–1017. doi: 10.1128/JB.01305-10
Liu, W., Zhou, D., Yuan, F., Liu, Z., Duan, Z., Yang, K., et al. (2019b). Surface Proteins Mhp390 (P68) Contributes to Cilium Adherence and Mediates Inflammation and Apoptosis in Mycoplasma Hyopneumoniae. Microb. Pathog. 126, 92–100. doi: 10.1016/j.micpath.2018.10.035
Liu, H., Zhu, Z., Xue, Q., Yang, F., Cao, W., Zhang, K., et al. (2019a). Foot-And-Mouth Disease Virus Antagonizes NOD2-Mediated Antiviral Effects by Inhibiting NOD2 Protein Expression. J. Virol. 93, e00124–19. doi: 10.1128/JVI.00124-19
Lupfer, C., Kanneganti, T. D. (2013). The Expanding Role of NLRs in Antiviral Immunity. Immunol. Rev. 255 (1), 13–24. doi: 10.1111/imr.12089
Lupfer, C., Thomas, P. G., Anand, P. K., Vogel, P., Milasta, S., Martinez, J., et al. (2013). Receptor Interacting Protein Kinase 2-Mediated Mitophagy Regulates Inflammasome Activation During Virus Infection. Nat. Immunol. 14 (5), 480–488. doi: 10.1038/ni.2563
McKernan, D. P. (2020). Pattern Recognition Receptors as Potential Drug Targets in Inflammatory Disorders. Adv. Protein Chem. Struct. Biol. 119, 65–109. doi: 10.1016/bs.apcsb.2019.09.001
Meyns, T., Maes, D., Calus, D., Ribbens, S., Dewulf, J., Chiers, K., et al. (2007). Interactions of Highly and Low Virulent Mycoplasma Hyopneumoniae Isolates With the Respiratory Tract of Pigs. Vet. Microbiol. 120 (1-2), 87–95. doi: 10.1016/j.vetmic.2006.10.010
Minion, F. C., Lefkowitz, E. J., Madsen, M. L., Cleary, B. J., Swartzell, S. M., Mahairas, G. G. (2004). The Genome Sequence of Mycoplasma Hyopneumoniae Strain 232, the Agent of Swine Mycoplasmosis. J. Bacteriol. 186 (21), 7123–7133. doi: 10.1128/JB.186.21.7123-7133.2004
Moreira, L. O., Zamboni, D. S. (2012). NOD1 and NOD2 Signaling in Infection and Inflammation. Front. Immunol. 3. doi: 10.3389/fimmu.2012.00328
Muneta, Y., Uenishi, H., Kikuma, R., Yoshihara, K., Shimoji, Y., Yamamoto, R., et al. (2003). Porcine TLR2 and TLR6: Identification and Their Involvement in Mycoplasma Hyopneumoniae Infection. J. Interferon Cytokine Res. 23 (10), 583–590. doi: 10.1089/107999003322485080
Murillo, L. S., Morre, S. A., Pena, A. S. (2003). Toll-Like Receptors and NOD/CARD Proteins: Pattern Recognition Receptors Are Key Elements in the Regulation of Immune Response. Drugs Today (Barc.) 39 (6), 415–438. doi: 10.1358/dot.2003.39.6.799448
Nueangphuet, P., Suwanruengsri, M., Fuke, N., Uemura, R., Hirai, T., Yamaguchi, R. (2021). Neutrophil and M2-Polarized Macrophage Infiltration, Expression of IL-8 and Apoptosis in Mycoplasma Hyopneumoniae Pneumonia in Swine. J. Comp. Pathol. 189, 31–44. doi: 10.1016/j.jcpa.2021.09.004
Okusawa, T., Fujita, M., Nakamura, J., Into, T., Yasuda, M., Yoshimura, A., et al. (2004). Relationship Between Structures and Biological Activities of Mycoplasmal Diacylated Lipopeptides and Their Recognition by Toll-Like Receptors 2 and 6. Infect. Immun. 72 (3), 1657–1665. doi: 10.1128/IAI.72.3.1657-1665.2004
Opitz, B., Puschel, A., Beermann, W., Hocke, A. C., Forster, S., Schmeck, B., et al. (2006). Listeria Monocytogenes Activated P38 MAPK and Induced IL-8 Secretion in a Nucleotide-Binding Oligomerization Domain 1-Dependent Manner in Endothelial Cells. J. Immunol. 176 (1), 484–490. doi: 10.4049/jimmunol.176.1.484
Opitz, B., Puschel, A., Schmeck, B., Hocke, A. C., Rosseau, S., Hammerschmidt, S., et al. (2004). Nucleotide-Binding Oligomerization Domain Proteins Are Innate Immune Receptors for Internalized Streptococcus Pneumoniae. J. Biol. Chem. 279 (35), 36426–36432. doi: 10.1074/jbc.M403861200
Oviedo-Boyso, J., Bravo-Patino, A., Baizabal-Aguirre, V. M. (2014). Collaborative Action of Toll-Like and NOD-Like Receptors as Modulators of the Inflammatory Response to Pathogenic Bacteria. Mediators Inflamm. 2014, 432785. doi: 10.1155/2014/432785
Philpott, D. J., Girardin, S. E. (2010). Nod-Like Receptors: Sentinels at Host Membranes. Curr. Opin. Immunol. 22 (4), 428–434. doi: 10.1016/j.coi.2010.04.010
Saade, G., Deblanc, C., Bougon, J., Marois-Crehan, C., Fablet, C., Auray, G., et al. (2020). Coinfections and Their Molecular Consequences in the Porcine Respiratory Tract. Vet. Res. 51 (1), 80. doi: 10.1186/s13567-020-00807-8
Segovia, J. A., Chang, T. H., Winter, V. T., Coalson, J. J., Cagle, M. P., Pandranki, L., et al. (2018). NLRP3 Is a Critical Regulator of Inflammation and Innate Immune Cell Response During Mycoplasma Pneumoniae Infection. Infect. Immun. 86 (1), e00548–17. doi: 10.1128/IAI.00548-17
Shimizu, T. (2016). Inflammation-Inducing Factors of Mycoplasma Pneumoniae. Front. Microbiol. 7. doi: 10.3389/fmicb.2016.00414
Shimizu, T., Kida, Y., Kuwano, K. (2005). A Dipalmitoylated Lipoprotein From Mycoplasma Pneumoniae Activates NF-Kappa B Through TLR1, TLR2, and TLR6. J. Immunol. 175 (7), 4641–4646. doi: 10.4049/jimmunol.175.7.4641
Takeda, K., Takeuchi, O., Akira, S. (2002). Recognition of Lipopeptides by Toll-Like Receptors. J. Endotoxin Res. 8 (6), 459–463. doi: 10.1179/096805102125001073
Takeuchi, O., Akira, S. (2007). Signaling Pathways Activated by Microorganisms. Curr. Opin. Cell Biol. 19 (2), 185–191. doi: 10.1016/j.ceb.2007.02.006
Takeuchi, O., Akira, S. (2010). Pattern Recognition Receptors and Inflammation. Cell 140 (6), 805–820. doi: 10.1016/j.cell.2010.01.022
Tourlomousis, P., Wright, J. A., Bittante, A. S., Hopkins, L. J., Webster, S. J., Bryant, O. J., et al. (2020). Modifying Bacterial Flagellin to Evade Nod-Like Receptor CARD 4 Recognition Enhances Protective Immunity Against Salmonella. Nat. Microbiol. 5 (12), 1588–1597. doi: 10.1038/s41564-020-00801-y
Travassos, L. H., Carneiro, L. A., Girardin, S. E., Boneca, I. G., Lemos, R., Bozza, M. T., et al. (2005). Nod1 Participates in the Innate Immune Response to Pseudomonas Aeruginosa. J. Biol. Chem. 280 (44), 36714–36718. doi: 10.1074/jbc.M501649200
Vegna, S., Gregoire, D., Moreau, M., Lassus, P., Durantel, D., Assenat, E., et al. (2016). NOD1 Participates in the Innate Immune Response Triggered by Hepatitis C Virus Polymerase. J. Virol. 90 (13), 6022–6035. doi: 10.1128/JVI.03230-15
Vissers, M., Remijn, T., Oosting, M., de Jong, D. J., Diavatopoulos, D. A., Hermans, P. W., et al. (2012). Respiratory Syncytial Virus Infection Augments NOD2 Signaling in an IFN-Beta-Dependent Manner in Human Primary Cells. Eur. J. Immunol. 42 (10), 2727–2735. doi: 10.1002/eji.201242396
Walsh, D., McCarthy, J., O'Driscoll, C., Melgar, S. (2013). Pattern Recognition Receptors–Molecular Orchestrators of Inflammation in Inflammatory Bowel Disease. Cytokine Growth Factor Rev. 24 (2), 91–104. doi: 10.1016/j.cytogfr.2012.09.003
Weissenbacher-Lang, C., Kureljusic, B., Nedorost, N., Matula, B., Schiessl, W., Stixenberger, D., et al. (2016). Retrospective Analysis of Bacterial and Viral Co-Infections in Pneumocystis Spp. Positive Lung Samples of Austrian Pigs With Pneumonia. PloS One 11 (7), e0158479. doi: 10.1371/journal.pone.0158479
Keywords: Mycoplasma hyopneumoniae, mhp390, NOD1, inflammation, interaction.
Citation: Liu W, Jiang P, Yang K, Song Q, Yuan F, Liu Z, Gao T, Zhou D, Guo R, Li C, Sun P and Tian Y (2022) Mycoplasma hyopneumoniae Infection Activates the NOD1 Signaling Pathway to Modulate Inflammation. Front. Cell. Infect. Microbiol. 12:927840. doi: 10.3389/fcimb.2022.927840
Received: 25 April 2022; Accepted: 20 June 2022;
Published: 08 July 2022.
Edited by:
Hui Jin, Huazhong Agricultural University, ChinaReviewed by:
Zhenyu Cheng, Dalhousie University, CanadaZhixin Feng, Jiangsu Academy of Agricultural Sciences (JAAS), China
Copyright © 2022 Liu, Jiang, Yang, Song, Yuan, Liu, Gao, Zhou, Guo, Li, Sun and Tian. This is an open-access article distributed under the terms of the Creative Commons Attribution License (CC BY). The use, distribution or reproduction in other forums is permitted, provided the original author(s) and the copyright owner(s) are credited and that the original publication in this journal is cited, in accordance with accepted academic practice. No use, distribution or reproduction is permitted which does not comply with these terms.
*Correspondence: Yongxiang Tian, dHl4YW5iaXRAMTYzLmNvbQ==; Pei Sun, c3VucGVpMTk3OUAxMjYuY29t
†These authors have contributed equally to this work