- Neisseria Research Group, Molecular Microbiology, Academic School of Clinical and Experimental Sciences, University of Southampton Faculty of Medicine, Southampton, United Kingdom
Neisseria pathogens express a Macrophage Infectivity Potentiator Protein (MIP), which belongs to the FK506 binding protein (FKBP) family of proteins that exhibit peptidyl-prolyl cis/trans isomerase (PPIase) activity. Neisseria MIP proteins are potential candidates for inclusion into vaccines for gonorrhoea caused by N. gonorrhoeae infection, and meningitis/sepsis caused by M. meningitidis infection. Neisseria MIP proteins are also potential targets for directed drug treatments, although this remains relatively unexplored. In this mini-review, we provide an update into the vaccine potential of Neisseria MIP and the few published drug targeting studies, and explore further the diversity of this protein amongst both pathogenic and commensal Neisseria spp.
Introduction
The genus Neisseria contains the pathogens Neisseria gonorrhoeae (Ng, gonococcus) and Neisseria meningitidis (Nm, meningocococus) that can infect humans, and several commensal species and species that can infect other mammals (Humbert and Christodoulides, 2019). The gonococcus is an obligate human pathogen, not part of the normal human urogenital tract microbiota, and causes the sexually transmitted disease (STD) gonorrhoea (Quillin and Seifert, 2018). There are ~87 million cases of gonorrhoea reported annually worldwide, with the highest burden of infection borne by people in the least developed and low-to-middle income countries (Rowley et al., 2019). This figure is certainly an underestimate due to unreported asymptomatic infections. Gonococci colonize primarily the mucosal epithelium of the male urethra, causing urethritis and a painful discharge, and the mucosal epithelium of the female endo/ectocervix, causing a mucopurulent cervicitis. Asymptomatic infections are common in both genders (Quillin and Seifert, 2018), but more serious for women, and in approximately 10-25% of untreated women, gonococci can ascend into the upper reproductive tract and induce a clinical syndrome of Pelvic Inflammatory Disease (PID). Patients with PID can suffer long-term and/or permanent sequelae, e.g. chronic pelvic pain, Fallopian tube damage, endometritis, ectopic pregnancy and infertility (Quillin and Seifert, 2018; Stevens and Criss, 2018). Atypical infections can occur at other anatomical sites, because of gonococci disseminating from the urogenital tract, or develop as primary infections due to the direct interaction of the pathogen (Humbert and Christodoulides, 2019). Co-infection with other STD pathogens e.g. Human Immunodeficiency Virus (HIV), Treponema pallidum, and Chlamydia, is not uncommon. Notably, gonococcal infection is known to promote HIV transmission and infection (Guvenc et al., 2020).
The meningococcus is a commensal organism present within the human nasopharyngeal microbiota that can behave as an opportunistic pathogen to cause Systemic Meningococcal Disease (SMD), manifested as meningitis and/or sepsis (Brandtzaeg and Van Deuren, 2012). Meningococcal infection has caused historically significant mortality and morbidity (Schoen et al., 2008), but the development and introduction of capsule polysaccharide (CPS)-conjugate vaccines and the protein-based Bexsero and Trumenba vaccines has dramatically reduced cases of infection worldwide (Christodoulides and Heckels, 2017; Rivero-Calle et al., 2019). SMD is wholly preventable by vaccination, but there are no vaccines to prevent gonorrhoea (Wetzler et al., 2016). Moreover, the successful control of gonorrhoea with antibiotics has been compromised, due to the ability of the gonococcus to rapidly develop resistance to every class of antibiotic introduced (Unemo et al., 2019). There is an urgent need for new antimicrobials for gonorrhoea and for candidate vaccines for human trials.
In this mini-review, our aim is to update our previous comprehensive review (Humbert et al., 2015), with published research on the vaccine and therapeutic target potential of the Neisseria Macrophage Infectivity Potentiator (MIP) protein, which belong to the FK506 binding protein (FKBP) family of proteins that exhibit peptidyl-prolyl cis/trans isomerase (PPIase) activity.
Biology and Structure of Neisseria MIP
Gonococci and meningococci both produce FKBP-type MIP PPIases that play roles in the pathogen-host interaction and offer potential targets for vaccines and therapies. Expression of a surface-exposed Ng-MIP lipoprotein (NG1225 = NEIS1487 in the http://pubmlst.org/Neisseria/database) of molecular mass (Mr) of 30 kDa appeared to be important for gonococcal persistence within mouse and human macrophages in vitro and for protection from the bactericidal activity of immune effector cells (Leuzzi et al., 2005). Ng-MIP protein was not involved in gonococcal adherence, since no differences were observed between parent and a Ng-MIP mutant in adhering to macrophages and subsequent invasion. However, the Ng-MIP mutant did show reduced survival following infection, suggesting that the protein played a role in the intracellular survival of the pathogen (Leuzzi et al., 2005). Ng-MIP appears to be expressed during infection in vivo and is immunogenic, with sera from patients with urethritis or disseminated gonococcal infections able to recognize purified Ng-MIP (Leuzzi et al., 2005; Starnino et al., 2010). Ng-MIP was identified as a target in gonococci for protein-linked O-glycosylation (Vik et al., 2009). Nm-MIP (NMB1567 = NEIS1487) is a MIP-like PPIase (Mr ~29 kDa) that was identified by proteomics in high abundance in the meningococcal outer membrane (OM) and found to share homology with the Legionella pneumophila (Lp)-MIP (Williams et al., 2007). Expression of Nm-MIP protein was important for bacterial survival in the blood, with expression of the nm-mip gene upregulated in an ex vivo human whole-blood model infected with wild type N. meningitidis strain MC58 (Echenique-Rivera et al., 2011).
However, it does appear that many PPIases are encoded within the Neisseria chromosome, and the reader can refer to a previous review (Humbert et al., 2015) for further information on these predominantly cytosolic proteins, including a Neisseria FKBP-like PPIase encoded by gene nmb0027 (Sampson and Gotschlich, 1992; McAllister and Stephens, 1993).
Based on the structure of Lp-MIP protein (Riboldi-Tunnicliffe et al., 2001; Ceymann et al., 2008), Neisseria MIP proteins exist as a homo-dimer in the bacterial OM, following cleavage of a N-terminal signal sequence peptide during transport across the cytoplasmic membrane. Each monomer of the homo-dimer can be structurally divided into i) a N-terminal α-helix that participates in a dimerization domain and is probably lipidated to allow anchoring of MIP into the OM (Bielecka et al., 2015); ii) a long connecting α-helix in the centre, ending with iii) a globular C-terminal domain containing the FKBP-type PPIase function (Figure 1A). BLAST sequence analyses demonstrated that the human (h)FKBP2 PPIase protein and Nm/Ng-MIP share ~ 48% amino acid similarity within the region located between amino acids 166 and 252 (Bielecka et al., 2015) (Figure 1B). Thus, Neisseria MIP is a partial molecular mimic of human FKBP protein, which may provide the pathogens with a survival advantage, possibly related to their survival within host cells and for immune avoidance.
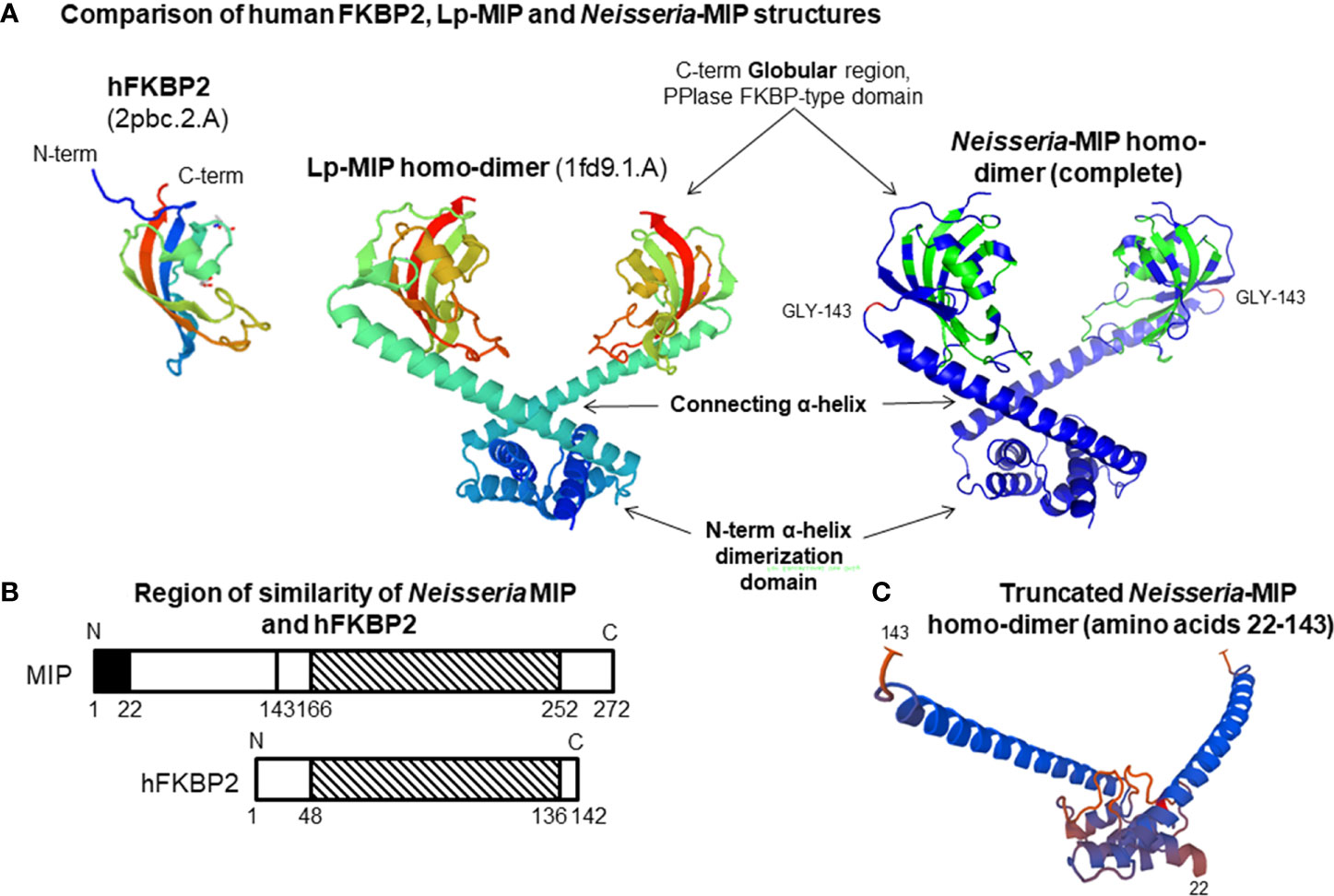
Figure 1 (A) Comparison of human FKBP2, Legionella pneumophila (Lp)-MIP and Neisseria-MIP structures. The Neisseria-MIP model is based on the crystal structure of Lp-MIP and consists of 3 domains as marked. Two monomers assemble to form a homo-dimer that associated with the bacterial OM. The structure of the human FKBP2 PPIase globular protein is shown for comparison. (B) Cartoon showing the overlapping region of similarity between Neisseria MIP and human FKBP2 protein. Similarity is within the striped C- terminal region. (C) Structure of the truncated MIP homo-dimer. The sequence of amino acids 22-143 located within the N-terminal region after removal of the globular regions that contain FKBP activity. Adapted from our figures published by Humbert et al. (2015) and Bielecka et al. (2015).
Update on Vaccine Potential of Neisseria MIP Proteins
Neisseria MIP proteins have several important attributes for inclusion into vaccines: the mip gene is present in all gonococci and meningococci examined thus far and the proteins are highly conserved, expressed by all strains (studied thus far), anchored in the OM and surface-exposed, and capable of inducing functional bactericidal antibodies (Leuzzi et al., 2005; Hung et al., 2011).
Ng-MIP and Nm-MIP are highly conserved proteins: in 2015, MIP allelic information and sequence diversity was examined in 5860 pathogenic Neisseria isolates in the http://pubmlst.org/Neisseria/database that had complete genome sequence information (Jolley and Maiden, 2010). For gonococci, the number of genomes that could be analyzed increased from 1217 in 2015 to 12805 in 2022, with the number of Ng-MIP allelic loci increasing from 25 to 66 and the number of non-redundant Ng-MIP amino acid sequences increasing from 16 to 32 (Table 1 and Supplementary Dataset 1). The Clustal alignment of the non-redundant allele amino acid sequences is shown in Supplementary Dataset 2 and the dendrogram in Supplementary Figure 1. The ng-mip gene was present in 100% of the genomes that could be analyzed using the PubMLST plug-in program. The dominant alleles expressed by gonococci were still alleles 10 and 35, with the number of isolates expressing allele 10 protein increasing from 49 to 64%, and the number of isolates expressing allele 35 decreasing from 35 to 22%. Despite these minor changes, expression of alleles 10 and 35 together still covered >84% of all gonococcal isolates examined, and >90% coverage would be obtained by including isolates expressing allele 140 protein (Supplementary Table 1).
For meningococci, the number of genomes that could be analyzed increased considerably from 4643 in 2015 to 26941 in 2022, with the number of Nm-MIP allelic loci increasing from 91 to 365 (with identifiable isolates) and the number of non-redundant Nm-MIP amino acid sequences increasing from 44 to 149 (Table 1 and Supplementary Dataset 3). The Clustal alignment of the non-redundant allele amino acid sequences is shown in Supplementary Dataset 4 and the dendrogram in Supplementary Figure 2. The nm-mip gene was present in all but one of the 26941 genomes that could be analyzed using the PubMLST plug-in program. The dominant alleles expressed by meningococci were still alleles 2, 1 and 7, with little significant change in the percentages of isolates expressing these alleles over the intervening years (Table 1). Taken together, expression of alleles 2, 1 and 7 proteins still covered >80% of all meningococcal isolates examined, and >90% coverage could be obtained by including the isolates expressing allele 22 and 13 proteins (Supplementary Table 1).
The vaccine potential of a recombinant (r)Nm-MIP protein was first shown by Hung et al. (Hung et al., 2011), who reported that antibodies raised to Type I rNm-MIP bound to the surface OM of meningococci and were bactericidal via a complement-dependent mechanism. Moreover, antibodies to Type 1 rNm-MIP had cross-strain bactericidal activity and could kill heterologous strains expressing Type II and III Nm-MIP proteins. Liposomal incorporation of rNm-MIP was preferred for inducing cross-strain bactericidal activity, whereas adsorption to Al(OH)3 was contra-indicated, suggesting that conformational refolding in the liposomal membrane is essential for inducing functional antibodies. Whether cross-protection extends to isolates expressing other allelic proteins has yet to be determined. Costoya and colleagues confirmed that liposomal presentation of a rMIP protein enhanced the bactericidal response (Costoya et al., 2017). Nm-MIP was also identified in a study of the meningococcal surface-ome and proteome (Tsolakos et al., 2014). MIP-specific antisera were shown to i) bind to whole meningococci in ELISA, ii) facilitate opsonophagocytosis and iii) deposit complement factors on the surface of meningococcal isolates of different serosubtypes.
Rational design of Neisseria MIPs for vaccines needs to consider i) the structure of the protein and ii) the potential amino acid sequence similarity with human PPIases. Clustal alignment of the amino acids of the whole protein encoded by Ng-MIP alleles 10, 35, 140 and Nm-MIP alleles 2, 1, 7, 22, 13, which provide >90% coverage of respective gonococcal and meningococcal isolates in the PubMLST Neisseria database, showed that there was overall ~96% sequence similarity (Supplementary Datasets 2, 4). There was ~93% sequence similarity in the N-terminal amino acid region 22 – 143 amongst all these proteins (Supplementary Datasets 2, 4). In order to bypass the homologous region of molecular mimicry of Neisseria MIP with hFKBP2 protein (amino acids 166 - 252), truncated proteins were generated by removing the globular domain that contains PPIase activity and sequence similarity. Significantly, a truncated rNm-MIP construct spanning amino acids 22 - 143 (Figure 1C) induced antibodies that killed strains expressing different Type Nm-MIP proteins and different CPS serogroups (Bielecka et al., 2015). Recently, we showed that antibodies raised to truncated rNm-MIP killed gonococci (Humbert and Christodoulides, 2018). These bactericidal antibodies do not cross-react with native pure hFKBP2 protein, whereas significant cross-reactivity was observed with antisera raised to full sequence rMIP proteins.
There is evidence that vaccination with meningococcal OM vesicle (OMV) vaccines can reduce the case incidence of gonorrhea. Anecdotal data from Cuba and Norway and the controlled retrospective case-control study in New Zealand suggested a decline in gonorrhea in the period immediately after use of meningococcal OMV vaccines (Petousis-Harris and Radcliff, 2019). The most robust data come from New Zealand, where a reduction in gonorrhea cases was observed after introduction of the MeNZB OMV vaccine. Vaccine effectiveness against gonorrhea in the population was estimated at 31% (95% Confidence Intervals 21–39) (Petousis-Harris et al., 2017). MenZB is a component of Bexsero, and a recent study in mice showed that immunization with Bexsero accelerated vaginal clearance of colonizing gonococci and that antibodies could recognize several gonococcal proteins (Leduc et al., 2020). One can speculate that antibodies to non-hFKBP2 regions of Nm-MIP in OMV contribute to protection against gonorrhoea. The MenZB strain is NZ98/254 (id 34542 in PubMLST/Neisseria database) and this strain expresses Allele 2 Type 1 Nm-MIP, the dominant allelic protein, which we know shares ~99% homology with Ng-MIP.
Another vaccine design approach was published by Gholami et al., who modelled in silico a chimeric antigen of Adhesin Complex Protein-MIP-PilQ (Gholami et al., 2015). Biophysical and biochemical properties and predictions of B cell, T cell and antigenic epitopes were made in silico, but disappointingly no in vivo immunological studies were reported. Moreover, the incorporation of full MIP protein into their design would be contra-indicated as shown above.
If the http://pubmlst.org/Neisseria/database is interrogated with analysis of gonococcal and meningococcal case reports for the recent year with the most complete available allele data, it is possible to estimate potential coverage of circulating isolates using MIP protein-based vaccines. For gonococci, the latest complete data are for 2019, in which there were 524 isolates of which 400 had Ng-MIP alleles identified. Consistent with the overall data shown in Table 1, the majority alleles for this most recent year were 10, 35 and 140 (Supplementary Table 2), which together accounted for 97% of all isolates. For meningococci, the latest complete data are for 2021, in which there were 198 isolates of which 166 had Nm-MIP alleles identified. Again, consistent with the overall data (Table 1), the majority alleles were 1 and 2, which together accounted for 80% of the isolates, with the inclusion of isolates expressing alleles 22 and 13 bringing this up to 90% coverage. Thus, vaccines containing 3 rNg-MIP proteins, or 3-5 rNm-MIP proteins, would be sufficient for almost total gonococcal and meningococcal isolate coverage, respectively. The observed cross-protection induced by any single recombinant Neisseria MIP protein (Hung et al., 2011; Bielecka et al., 2015; Humbert and Christodoulides, 2018) will reduce the number of antigens that are needed.
Presence of MIP in Commensal Neisseria
The non-pathogenic Neisseria species comprise part of the commensal bacterial microbiota of the human and animal oropharynx, but these ‘apparently harmless’ commensals can produce infection in a wide variety of anatomical sites including the heart, nervous system (to cause meningitis), bloodstream (to cause septicaemia), respiratory tract, bone marrow, skin and genital tract. The distribution and location of these commensal Neisseria in and on the human body have been reviewed extensively elsewhere (Weyand, 2017; Humbert and Christodoulides, 2019). Commensal Neisseria in the upper respiratory tract (URT) can compete with meningococci for niche occupation and provide some protection against colonization. Neisseria spp have been identified in the URTs of many mammals, including dogs, cats, cattle and non-human primates. N. mucosa has been cultured even from the woodlouse, and from environmental water and sediment (Liu et al., 2015). Although animal to human transmission of Neisseria spp has been reported, e.g. through bite wounds and then causing infection, Neisseria spp are not zoonotic organisms in the strict sense of the term. Interestingly, the pathogenic Neisseria have been found in other mammals, with an intriguing recent report of gonococci isolated from the vaginal microbiome of giant pandas (Zhang et al., 2020).
With the increase in available genomes in the PubMLST/Neisseria database, it is possible to examine whether human commensal species and species that colonize other mammals also express MIP, and the diversity of their allelic expression. Of the 39 species additional to N. gonorrhoeae and N. meningitidis, and the two categories identified as Neisseria sp and Non-Neisseria sp, in the PubMLST/Neisseria database, MIP alleles were present in 13 species (basseii, benedictiae, bergeri, blantyrii, cinerea, lactamica, maigaei, mucosa, oralis, polysaccharea, subflava, uirgultaei, viridiae) and within the Neisseria sp category (Supplementary Table 3). A consideration for any Neisseria vaccine antigen is whether they are also potentially expressed by commensal Neisseria spp, since generated immune responses towards vaccine antigens may also target commensal species. This is particularly relevant to meningococci, as the 13 species isolates expressing MIP allele proteins have been isolated from throat swabs and are thus present in the oral cavity and nasopharynx. None of these 13 species isolates expressed MIP protein encoded by the dominant meningococcal Allele 1, 2, 7, 22 or 13. However, we did identify some minor allele proteins shared by these species with meningococci (Supplementary Table 3), notably allele 27 with bergeri, allele 113 with cinerea, alleles 9, 15, 58 and 324 with lactamica and alleles 88 and 229 with polysaccharea. No allele proteins were shared between gonococci and any of these other Neisseria spp (Supplementary Table 3). The one Neisseria sp category isolate IE21Nm045, id 95638, expressed Allele 10-encoded MIP protein and was isolated from the eye and was suggested to be a gonococcus by rMLST analysis.
Although commensal Neisseria spp do not share the major allele-encoded MIP proteins of the pathogens, the amino acid sequence similarities between the dominant gonococcal and meningococcal allele proteins and other allele proteins may still be high. To check this, we did a Clustal alignment of MIP proteins encoded by alleles 1, 2, 7, 22, 13 (dominant meningococcal) and 10, 34, 140 (dominant gonococcal) with MIP allele proteins from each of the other 13 species that are expressed by most isolates belonging to each of the species and excluding the ones mentioned in the previous paragraph (Supplementary Dataset 5). Examination of the vaccine-relevant N-terminal amino acid sequence 22-143 showed that there is significant similarity between the commensal and pathogen MIP protein sequences. Thus, within this sequence, Ng/Nm-MIP share ~ 93% sequence similarity with benedictiae and cinerea MIP, and 95-97% similarity with basserii, bergeri, blantyrii, lactamica, maigaei, polysaccharea, viridae and uirgultaei MIP. By contrast, Ng/Nm-MIP share only 69% similarity with oralis MIP, and 71% and 73% similarity with subflava and mucosa MIP respectively (Supplementary Dataset 5).
The structure of the MIP proteins from different Neisseria spp can be modelled on the crystal structure of Lp-MIP. As expected, there is remarkable conservation of structure between the MIP proteins (Supplementary Figure 3). Thus, there is the potential for antibodies generated to MIP, in particular Nm-MIP, to cross-react with MIP proteins expressed by another Neisseria spp. Commensal species occupying niches in the URT that bind anti-MIP antibodies could act inadvertently as decoys by adsorbing some of the available antibody pool and reducing immune clearance of colonizing pathogens. This would provide the pathogen with an advantage for colonization, although it is likely that other bacteria in the microbiota will still compete for these available niches.
Neisseria MIP Proteins as Drug Targets
PPIase enzymes catalyze the slow cis-trans isomerization of proline imidic peptide bonds in oligopeptides and proteins and accelerate protein folding. PPIase activity has been shown for many bacterial MIP proteins, including Lp-MIP, Ng-MIP, Chlamydia trachomatis-MIP, Coxiella burnetii-MIP, Burkholderia pseudomallei (Bp)-MIP, E.coli-MIP, Shewanella sp. SIB1-MIP, and Vibrio anguillarum-MIP (Fischer et al., 1992; Lundemose et al., 1993; Mo et al., 1995; Suzuki et al., 2004; Leuzzi et al., 2005; Norville et al., 2011; Jana et al., 2012; Kim et al., 2014), as well as a MIP from the parasite Trypanosoma cruzi (Moro et al., 1995). PPIase activity of bacterial MIP proteins is commonly measured using the protease-coupled assay (Fischer et al., 1984; Fischer et al., 1992). Briefly, succinamide-Ala-Phe-Pro-Phe-p-nitroalanine peptide substrate is incubated with purified MIP (in the presence or absence of various inhibitor concentrations) for 4 min at 10°C in 35 mM HEPES/NaOH buffer (pH 7.8). The reaction is started by adding the isomer specific protease chymotrypsin, and the release of 4-nitroaniline is monitored by measuring absorbance at λ 390nm over 15 min.
MIP proteins offer targets for inhibitory drugs. PPIase activity can be inhibited by the fungal immunosuppressant macrocyclic compounds of rapamycin (sirolimus) and FK506 (tacrolimus) (Schreiber, 1991). Ng-MIP PPIase activity can be inhibited by rapamycin (Leuzzi et al., 2005). A recent study showed that pipecolic acid derivatives PipN3 and PipN4, originally shown to be inhibitors of Lp-MIP (Juli et al., 2011), inhibited the PPIase activity of Ng-MIP and affected the survival of gonococci in the presence of neutrophils (Reimer et al., 2016). PipN3 and PipN4 also inhibited the invasion and intracellular survival of meningococci in Detroit 562 epithelial cells. Subsequently, Seufert et al. established a library of pipecolic acid derivatives and used hot-spot analyses and exploratory docking experiments against Lp-Mip and Bp-Mip to suggest possible structure modifications to improve inhibitory activity (Seufert et al., 2016). Coupled with considerations of drug toxicity and stability, the authors synthesized and evaluated compounds in PPIase inhibition assays and identified compound S-5x as the most active inhibitor with the best safety profile. In general, such structure-activity analysis approaches, whereby the chemistry of potential inhibitors is modified to improve activity, can be applied to developing bacterial MIP PPIase inhibitors. Studies with purified MIP proteins in vitro would then select those drugs that can progress to in vivo activity and toxicity studies in animal infection models.
A proteomics study of the gonococcal response to sub-inhibitory concentrations of extended-spectrum cephalosporins such as ceftriaxone, found increased expression of Ng-MIP, and it was proposed that this up-regulated expression might be an adaptation or a tolerance mechanism of the bacteria in response to antibiotic stress (Nabu et al., 2017).
Conclusions and Future Work
In summary, species of the genus Neisseria express a FKBP-type MIP protein in their OM. The key characteristics of MIP are that 1) anti-MIP antibodies that bind to the surfaces of pathogenic gonococci and meningococci are bactericidal, and 2) it may offer an OM-located drug target to help treat infections caused by antibiotic-resistant gonococci. Future work on MIP should focus on these two aspects. MIP deserves to be explored perhaps as a component of the first generation of modern antigen-specific gonococcal vaccines that are currently being developed (Wetzler et al., 2016). Perhaps revisiting the strategy to over-express truncated MIP in OMV should also be considered (Humbert and Christodoulides, 2018). What is unknown also is the peptide epitope sequence(s) that is/are responsible for inducing bactericidal antibodies and whether these sequences vary much between the different MIP proteins. Identifying these epitopes may offer the opportunity to use structural vaccinology approaches to develop refined subunit vaccines. Furthermore, even though studies suggest that MIP is a potential drug target, development of drugs to inhibit its virulence properties has not progressed. And finally, whether gonococci develop resistance to anti-MIP drugs is not known and should be addressed for any promising new therapeutics.
Author Contributions
MC wrote the article and updated the literature from a previous in-depth review published elsewhere.
Conflict of Interest
The author declares that the article was written without any commercial or financial relationships that could be construed as potential conflicts of interest.
Publisher’s Note
All claims expressed in this article are solely those of the authors and do not necessarily represent those of their affiliated organizations, or those of the publisher, the editors and the reviewers. Any product that may be evaluated in this article, or claim that may be made by its manufacturer, is not guaranteed or endorsed by the publisher.
Supplementary Material
The Supplementary Material for this article can be found online at: https://www.frontiersin.org/articles/10.3389/fcimb.2022.861489/full#supplementary-material
Supplementary Figure 1 | Dendrogram for the 32 non-redundant Ng-MIP alleles.
Supplementary Figure 2 | Dendrogram for the 149 non-redundant Nm-MIP alleles.
Supplementary Figure 3 | Comparison of the structures of MIP from pathogenic and commensal Neisseria spp. Each structure used built on the template 1fd9.1. of Legionella pneumophila using SWISS-MODEL.
Abbreviations
MIP, macrophage infectivity potentiator; FKBP, FK506 binding protein (FKBP) family of proteins; PPIase, peptidyl-prolyl cis/trans isomerase; PID, pelvic inflammatory disease; HIV, human immunodeficiency virus; SMD, systemic (invasive) meningococcal disease; Nm, Neisseria meningitidis; Ng, Neisseria gonorrhoeae; MLST, multi-locus sequence typing; Supplementary Material; BIGSbd, Bacterial Isolate Genome Sequence database; OM, outer membrane; OMV, outer membrane vesicle; Al(OH)3, aluminium hydroxide; Pip, pipecolic acid; Lp-MIP, Legionella pneumophilia MIP.URT, upper respiratory tract; CPS, capsule polysaccharide.
References
Bielecka, M. K., Devos, N., Gilbert, M., Hung, M. C., Weynants, V., Heckels, J. E., et al. (2015). Recombinant Protein Truncation Strategy for Inducing Bactericidal Antibodies to the Macrophage Infectivity Potentiator Protein of Neisseria Meningitidis and Circumventing Potential Cross-Reactivity With Human FK506-Binding Proteins. Infect. Immun. 83, 730–742. doi: 10.1128/IAI.01815-14
Brandtzaeg, P., Van Deuren, M. (2012). “Classification and Pathogenesis of Meningococcal Infections,” in Neisseria Meningitidis:Advanced Methods and Protocols. Ed. Christodoulides, M. (Humana Press), New York, New York 21–35.
Ceymann, A., Horstmann, M., Ehses, P., Schweimer, K., Paschke, A. K., Steinert, M., et al. (2008). Solution Structure of the Legionella Pneumophila Mip-Rapamycin Complex. BMC.Struct.Biol 8, 17. doi: 10.1186/1472-6807-8-17
Christodoulides, M., Heckels, J. (2017). Novel Approaches to Neisseria Meningitidis Vaccine Design. Pathog. Dis. 73, ftx033. doi: 10.1093/femspd/ftx033
Costoya, L., Marzoa, J., Ferreiros, C., Criado, M. T. (2017). Liposomes or Traditional Adjuvants: Induction of Bactericidal Activity by the Macrophage Infectivity Potentiator Protein (Mip) of Neisseria Meningitidis. APMIS 125, 725–731. doi: 10.1111/apm.12709
Echenique-Rivera, H., Muzzi, A., Del Tordello, E., Seib, K. L., Francois, P., Rappuoli, R., et al. (2011). Transcriptome Analysis of Neisseria Meningitidis in Human Whole Blood and Mutagenesis Studies Identify Virulence Factors Involved in Blood Survival. PloS Pathog. 7, e1002027. doi: 10.1371/journal.ppat.1002027
Fischer, G., Bang, H., Ludwig, B., Mann, K., Hacker, J. (1992). MIP Protein of Legionella Pneumophila Exhibits Peptidyl-Prolyl-Cis Trans Isomerase (PPlase) Activity. Mol. Microbiol. 6, 1375–1383. doi: 10.1111/j.1365-2958.1992.tb00858.x
Fischer, G., Bang, H., Mech, C. (1984). [Determination of Enzymatic Catalysis for the Cis-Trans-Isomerization of Peptide Binding in Proline-Containing Peptides]. BioMed. Biochim. Acta 43, 1101–1111.
Gholami, M., Salimi Chirani, A., Moshiri, M., Sedighi, M., Pournajaf, A., Tohidfar, M., et al. (2015). In Silico Analysis and Modeling of ACP-MIP-PilQ Chimeric Antigen From Neisseria Meningitidis Serogroup B. Rep. Biochem. Mol. Biol. 4, 50–59.
Guvenc, F., Kaul, R., Gray-Owen, S. D. (2020). Intimate Relations: Molecular and Immunologic Interactions Between Neisseria Gonorrhoeae and HIV-1. Front. Microbiol. 11, 1299. doi: 10.3389/fmicb.2020.01299
Humbert, M. V., Almonacid Mendoza, H. L., Jackson, A. C., Hung, M. C., Bielecka, M. K., Heckels, J. E., et al. (2015). Vaccine Potential of Bacterial Macrophage Infectivity Potentiator (MIP)-Like Peptidyl Prolyl Cis/Trans Isomerase (PPIase) Proteins. Exp. Rev. Vacc 14, 1633–1649. doi: 10.1586/14760584.2015.1095638
Humbert, M. V., Christodoulides, M. (2018). Immunization With Recombinant Truncated Neisseria Meningitidis-Macrophage Infectivity Potentiator (rT-Nm-MIP) Protein Induces Murine Antibodies That are Cross-Reactive and Bactericidal for Neisseria Gonorrhoeae. Vaccine 36 (27), 3926–3936 doi: 10.1016/j.vaccine.2018.05.069
Humbert, M. V., Christodoulides, M. (2019). Atypical, Yet Not Infrequent, Infections With Neisseria Species. Pathogens 9 (1), 10. doi: 10.3390/pathogens9010010
Hung, M. C., Salim, O., Williams, J. N., Heckels, J. E., Christodoulides, M. (2011). The Neisseria Meningitidis Macrophage Infectivity Potentiator Protein Induces Cross-Strain Serum Bactericidal Activity and is a Potential Serogroup B Vaccine Candidate. Infect. Immun. 79, 3784–3791. doi: 10.1128/IAI.05019-11
Jana, B., Bandhu, A., Mondal, R., Biswas, A., Sau, K., Sau, S. (2012). Domain Structure and Denaturation of a Dimeric Mip-Like Peptidyl-Prolyl Cis–Trans Isomerase From Escherichia Coli. Biochemistry 51, 1223–1237. doi: 10.1021/bi2015037
Jolley, K. A., Maiden, M. C. (2010). BIGSdb: Scalable Analysis of Bacterial Genome Variation at the Population Level. BMC Bioinf. 11, 595. doi: 10.1186/1471-2105-11-595
Juli, C., Sippel, M., Jager, J., Thiele, A., Weiwad, M., Schweimer, K., et al. (2011). Pipecolic Acid Derivatives as Small-Molecule Inhibitors of the Legionella MIP Protein. J. Med. Chem. 54, 277–283. doi: 10.1021/jm101156y
Kim, S.-H., Lee, J. M., Kang, D. S., Kim, D.-G., Ahn, S.-H., Kong, I.-S. (2014). Expression, Purification and Characterization of Soluble Recombinant Peptidyl-Prolyl Cis/Trans Isomerase From Vibrio Anguillarum. Protein Expression Purification 101, 54–60. doi: 10.1016/j.pep.2014.06.005
Leduc, I., Connolly, K. L., Begum, A., Underwood, K., Darnell, S., Shafer, W. M., et al. (2020). The Serogroup B Meningococcal Outer Membrane Vesicle-Based Vaccine 4cmenb Induces Cross-Species Protection Against Neisseria Gonorrhoeae. PloS Pathog. 16, e1008602. doi: 10.1371/journal.ppat.1008602
Leuzzi, R., Serino, L., Scarselli, M., Savino, S., Fontana, M., Monaci, E., et al. (2005). Ng-MIP, a Surface-Exposed Lipoprotein of Neisseria Gonorrhoeae, has a Peptidyl-Prolyl Cis/Trans Isomerase (PPIase) Activity and is Involved in Persistence in Macrophages. Mol. Microbiol. 58, 669–681. doi: 10.1111/j.1365-2958.2005.04859.x
Liu, G., Tang, C. M., Exley, R. M. (2015). Non-Pathogenic Neisseria: Members of an Abundant, Multi-Habitat, Diverse Genus. Microbiol 161, 1297–1312. doi: 10.1099/mic.0.000086
Lundemose, A. G., Kay, J. E., Pearce, J. H. (1993). Chlamydia Trachomatis MIP-Like Protein has Peptidyl-Prolyl Cis Trans Isomerase Activity That is Inhibited by Fk506 and Rapamycin and is Implicated in Initiation of Chlamydial Infection. Mol. Microbiol. 7, 777–783. doi: 10.1111/j.1365-2958.1993.tb01168.x
Mcallister, C. F., Stephens, D. S. (1993). Analysis in Neisseria Meningitidis and Other Neisseria Species of Genes Homologous to the FKBP Immunophilin Family. Mol.Microbiol. 10, 13–23. doi: 10.1111/j.1365-2958.1993.tb00899.x
Mo, Y. Y., Cianciotto, N. P., Mallavia, L. P. (1995). Molecular Cloning of a Coxiella Burnetii Gene Encoding a Macrophage Infectivity Potentiator (Mip) Analogue. Microbiol 141 ( Pt 11), 2861–2871. doi: 10.1099/13500872-141-11-2861
Moro, A., Ruizcabello, F., Fernandezcano, A., Stock, R. P., Gonzalez, A. (1995). Secretion by Trypanosoma Cruzi of A Peptidyl-Prolyl Cis-Trans Isomerase Involved in Cell Infection. EMBO J. 14, 2483–2490. doi: 10.1002/j.1460-2075.1995.tb07245.x
Nabu, S., Lawung, R., Isarankura-Na-Ayudhya, P., Roytrakul, S., Dolprasit, S., Sengyee, S., et al. (2017). Comparative Proteomics Analysis of Neisseria Gonorrhoeae Strains in Response to Extended-Spectrum Cephalosporins. Excli J. 16, 1207–1229. doi: 10.17179/excli2017-832
Norville, I. H., Harmer, N. J., Harding, S. V., Fischer, G., Keith, K. E., Brown, K. A., et al. (2011). A Burkholderia Pseudomallei Macrophage Infectivity Potentiator-Like Protein has Rapamycin-Inhibitable Peptidylprolyl Isomerase Activity and Pleiotropic Effects on Virulence. Infect. Immun. 79, 4299–4307. doi: 10.1128/IAI.00134-11
Petousis-Harris, H., Paynter, J., Morgan, J., Saxton, P., Mcardle, B., Goodyear-Smith, F., et al. (2017). Effectiveness of a Group B Outer Membrane Vesicle Meningococcal Vaccine Against Gonorrhoea in New Zealand: A Retrospective Case-Control Study. Lancet 390, 1603–1610. doi: 10.1016/S0140-6736(17)31449-6
Petousis-Harris, H., Radcliff, F. J. (2019). Exploitation of Neisseria Meningitidis Group B OMV Vaccines Against N. Gonorrhoeae to Inform the Development and Deployment of Effective Gonorrhea Vaccines. Front. Immunol. 10, 683. doi: 10.3389/fimmu.2019.00683
Quillin, S. J., Seifert, H. S. (2018). Neisseria Gonorrhoeae Host Adaptation and Pathogenesis. Nat. Rev. Microbiol. 16, 226–240. doi: 10.1038/nrmicro.2017.169
Reimer, A., Seufert, F., Weiwad, M., Ebert, J., Bzdyl, N. M., Kahler, C. M., et al. (2016). Inhibitors of Macrophage Infectivity Potentiator-Like PPIases Affect Neisserial and Chlamydial Pathogenicity. Int. J. Antimicrob. Agents 48, 401–408. doi: 10.1016/j.ijantimicag.2016.06.020
Riboldi-Tunnicliffe, A., Konig, B., Jessen, S., Weiss, M. S., Rahfeld, J., Hacker, J., et al. (2001). Crystal Structure of Mip, a Prolylisomerase From Legionella Pneumophila. Nat.Struct.Biol 8, 779–783. doi: 10.1038/nsb0901-779
Rivero-Calle, I., Raguindin, P. F., Gómez-Rial, J., Rodriguez-Tenreiro, C., Martinón-Torres, F. (2019). Meningococcal Group B Vaccine For The Prevention Of Invasive Meningococcal Disease Caused By Neisseria Meningitidis Serogroup B. Infect. Drug Resist. 12, 3169–3188. doi: 10.2147/IDR.S159952
Rowley, J., Vander Hoorn, S., Korenromp, E., Low, N., Unemo, M., Abu-Raddad, L. J., et al. (2019). Chlamydia, Gonorrhoea, Trichomoniasis and Syphilis: Global Prevalence and Incidence Estimates, 2016. Bull. World Health Organ 97, 548–562P. doi: 10.2471/BLT.18.228486
Sampson, B. A., Gotschlich, E. C. (1992). Neisseria Meningitidis Encodes an FK506-Inhibitable Rotamase. Proc. Natl. Acad. Sci. U.S.A. 89, 1164–1168. doi: 10.1073/pnas.89.4.1164
Schoen, C., Blom, J., Claus, H., Schramm-Gluck, A., Brandt, P., Muller, T., et al. (2008). Whole-Genome Comparison of Disease and Carriage Strains Provides Insights Into Virulence Evolution in Neisseria Meningitidis. Proc. Natl. Acad. Sci. U.S.A. 105, 3473–3478. doi: 10.1073/pnas.0800151105
Schreiber, S. L. (1991). Chemistry and Biology of the Immunophilins and Their Immunosuppressive Ligands. Science 251, 283–287. doi: 10.1126/science.1702904
Seufert, F., Kuhn, M., Hein, M., Weiwad, M., Vivoli, M., Norville, I. H., et al. (2016). Development, Synthesis and Structure-Activity-Relationships of Inhibitors of the Macrophage Infectivity Potentiator (Mip) Proteins of Legionella Pneumophila and Burkholderia Pseudomallei. Bioorg Med. Chem. 24, 5134–5147. doi: 10.1016/j.bmc.2016.08.025
Starnino, S., Leuzzi, R., Ghisetti, V., De Francesco, M. A., Cusini, M., Impara, G., et al. (2010). Molecular Analysis of Two Novel Neisseria Gonorrhoeae Virulent Components: The Macrophage Infectivity Potentiator and the Outer Membrane Protein A. New Microbiol. 33, 167–170.
Stevens, J. S., Criss, A. K. (2018). Pathogenesis of Neisseria Gonorrhoeae in the Female Reproductive Tract: Neutrophilic Host Response, Sustained Infection, and Clinical Sequelae. Curr. Opin. Hematol. 25, 13–21. doi: 10.1097/MOH.0000000000000394
Suzuki, Y., Haruki, M., Takano, K., Morikawa, M., Kanaya, S. (2004). Possible Involvement of an FKBP Family Member Protein From a Psychrotrophic Bacterium Shewanella Sp. SIB1 in Cold-Adaptation. Eur. J. Biochem. 271, 1372–1381. doi: 10.1111/j.1432-1033.2004.04049.x
Tsolakos, N., Brookes, C., Taylor, S., Gorringe, A., Tang, C. M., Feavers, I. M., et al. (2014). Identification of Vaccine Antigens Using Integrated Proteomic Analyses of Surface Immunogens From Serogroup B Neisseria Meningitidis. J. Prot 101, 63–76. doi: 10.1016/j.jprot.2014.02.013
Unemo, M., Golparian, D., Eyre, D. W. (2019). Antimicrobial Resistance in Neisseria Gonorrhoeae and Treatment of Gonorrhea. Methods Mol. Biol. 1997, 37–58. doi: 10.1007/978-1-4939-9496-0_3
Vik, A., Aas, F. E., Anonsen, J. H., Bilsborough, S., Schneider, A., Egge-Jacobsen, W., et al. (2009). Broad Spectrum O-Linked Protein Glycosylation in the Human Pathogen Neisseria Gonorrhoeae. Proc. Natl. Acad. Sci. U. S. A. 106, 4447–4452. doi: 10.1073/pnas.0809504106
Wetzler, L. M., Feavers, I. M., Gray-Owen, S. D., Jerse, A. E., Rice, P. A., Deal, C. D. (2016). Summary and Recommendations From the National Institute of Allergy and Infectious Diseases (NIAID) Workshop "Gonorrhea Vaccines: The Way Forward". Clin. Vacc Immunol. 23, 656–663. doi: 10.1128/CVI.00230-16
Weyand, N. J. (2017). Neisseria Models of Infection and Persistence in the Upper Respiratory Tract. Pathog. Dis. 73 (3). doi: 10.1093/femspd/ftx031
Williams, J. N., Skipp, P. J., Humphries, H. E., Christodoulides, M., O'connor, C. D., Heckels, J. E. (2007). Proteomic Analysis of Outer Membranes and Vesicles From Wild-Type Serogroup B Neisseria Meningitidis and a Lipopolysaccharide-Deficient Mutant. Infect. Immun. 75, 1364–1372. doi: 10.1128/IAI.01424-06
Keywords: Neisseria meningitidis, Neisseria gonorrhoeae, Macrophage Infectivity Potentiator, vaccine, targeted treatments, commensal Neisseriae
Citation: Christodoulides M (2022) Update on the Neisseria Macrophage Infectivity Potentiator-Like PPIase Protein. Front. Cell. Infect. Microbiol. 12:861489. doi: 10.3389/fcimb.2022.861489
Received: 24 January 2022; Accepted: 07 February 2022;
Published: 22 March 2022.
Edited by:
Michael Steinert, Technische Universitat Braunschweig, GermanyReviewed by:
Vera Kozjak-Pavlovic, Julius Maximilian University of Würzburg, GermanyCopyright © 2022 Christodoulides. This is an open-access article distributed under the terms of the Creative Commons Attribution License (CC BY). The use, distribution or reproduction in other forums is permitted, provided the original author(s) and the copyright owner(s) are credited and that the original publication in this journal is cited, in accordance with accepted academic practice. No use, distribution or reproduction is permitted which does not comply with these terms.
*Correspondence: Myron Christodoulides, bWM0QHNvdG9uLmFjLnVr