- 1School of Chemistry, University of Southampton, Southampton, United Kingdom
- 2Magritek GmbH, Aachen, Germany
- 3UK National Crystallography Service, School of Chemistry, University of Southampton, Southampton, United Kingdom
Fluorine spin pairs that are constrained in spatial proximity show large scalar spin-spin couplings, despite the atoms being separated by several bonds. This is due to a non-bonded atomic interaction related to partial overlapping of fluorine p-orbitals. In this paper we exploit this phenomenon to create long-lived singlet spin order on the fluorine spin pair. This form of order, which, in this example molecule, is more than an order of magnitude longer than longitudinal order, has the potential to be useful in magnetic resonance imaging and molecular tracing experiments, because of the lack of endogenous fluorine in the human body and the high sensitivity achievable in 19F NMR.
1 Introduction
Molecular systems containing two fluorine atoms that are separated by several chemical bonds but close in space, exhibit significant indirect scalar spin-spin coupling constants. In a first observation in 1956, Saika and Gutowsky were surprised by the large 5
Systems of two scalar-coupled spin-1/2 nuclei, can be prepared to support singlet spin order, a particular type of nuclear spin order that has been shown to decay much slower than longitudinal and transverse magnetization, the latter two forms of spin order being used in all magnetic resonance experiments. Such a slower decay has been exploited in many applications including storage of hyperpolarization (Vasos et al., 2009; Pileio et al., 2013; Kiryutin et al., 2019), investigation of weak ligand-protein binding (Salvi et al., 2012), measurements of slow chemical exchange (Sarkar et al., 2007), small diffusion coefficients (Cavadini et al., 2005), diffusion and tortuosity in porous media (Dumez et al., 2014; Pileio et al., 2015; Pileio and Ostrowska, 2017; Tourell et al., 2018; Melchiorre et al., 2023). Most of these applications were so far based on singlet order created either in 1H spin-1/2 pairs (due to the molecule of interest or to maximize sensitivity) or in 13C- or 15N-doubly enriched molecules, carefully designed to maximize singlet order lifetimes. Recently, examples of singlet spin order in 31P (Korenchan et al., 2022) and 103Rh spin pairs (Harbor-Collins et al., 2024) were also reported.
In this paper, we report long-lived singlet spin order in molecules containing pairs of 19F nuclei coupled via non-bonded scalar interactions of “through-space” type. Accessing and exploiting singlet spin order in molecules containing pairs of 19F nuclei is interesting because 19F has a large gyromagnetic ratio (high NMR sensitivity), an essentially 100% natural abundance (no need for isotopic labelling), and because there is no endogenous fluorine in living organisms. This means there is no background signal in bio-medical applications, which contrasts with the intrinsic limitation in singlet NMR of molecules containing 1H pairs. However, a large chemical shift range of several hundreds of ppm’s and a typically small scalar coupling1 of molecules containing 19F spin pairs (Dolbier, 2016) has not played in favour of singlet order applications because this form of spin order is an eigenoperator of the Hamiltonian superoperator only in condition of nearly-magnetic-equivalence, i.e., when the chemical shift frequency difference between the two coupled nuclei is small compared to their spin mutual scalar coupling frequency. Conversely, some of the systems displaying “through-space” coupling, such as substituted 1,8-difluoronaphthalenes and 4,5-difluorophenanthrenes have the potential to meet the conditions for near-magnetic-equivalence, capitalising on their large “through-space” coupling, which is of the order of 170–190 Hz. In addition, and as exploited below, one can benefit from modern desktop NMR spectrometers and use their relatively low static magnetic field (typically less than 2 T) to reduce the chemical shift frequency difference between the two 19F spins to meet the conditions for near-magnetic-equivalence in a larger pool of 19F spin pairs, while maintaining good sensitivity and high spectral resolution characteristic of these machines.
2 Materials and methods
2.1 Molecular design, chemical synthesis and characterisation
In seeking a molecular scaffold to support a 19F spin pair of nearly-magnetic-equivalence, we speculated, based on visual inspection, that molecule I might be a suitable candidate. Moreover, the synthetic route described by Murai et al. (2022). appeared amenable for modification such that hydrogen atoms providing deleterious singlet relaxation mechanisms may be substituted with alternative groups possessing more desirable magnetic properties for our purposes. Accordingly, we prepared molecule I, and derivative molecules II and III, bearing deuterium and methoxy-
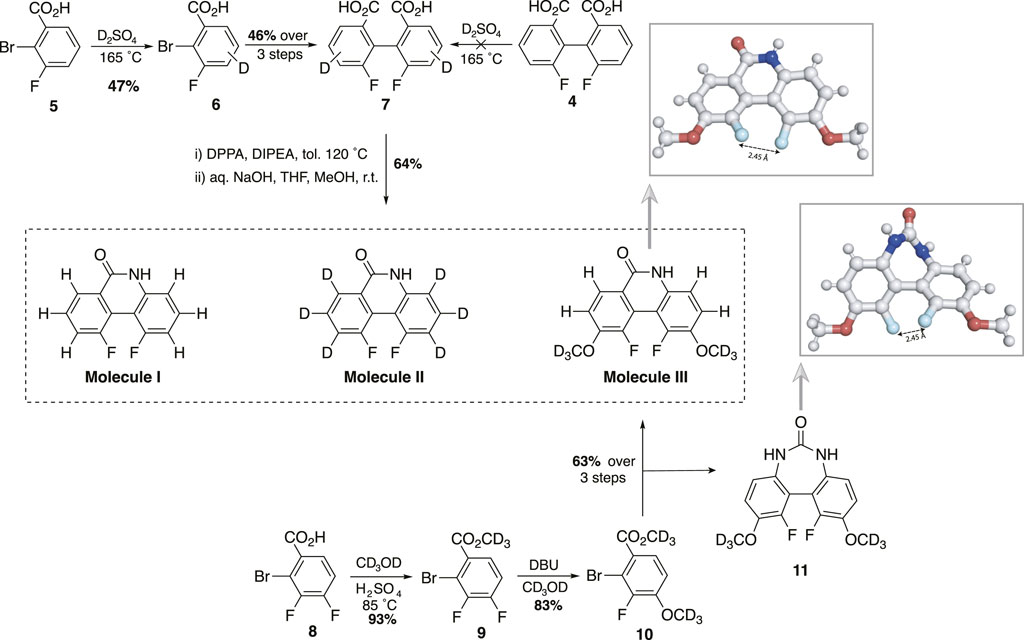
Scheme 1. Synthesis of molecules I, II, III (DBU, 1,8-diazabicyclo (5.4.0)undec-7-ene; DPPA, diphenylphosphoryl azide; DIPEA, N,N-diisopropylethylamine; THF, tetrahydrofuran; Me,
2.2 NMR samples
Each of the three difluoro-substituted molecules I, II and III were dissolved in DMSO-
2.3 NMR experiments
NMR experiments where performed using two different NMR spectrometers: a 9.4 T Bruker magnet coupled to an Avance II console and equipped with a selective fluorine probehead for observation of 19F with 1H decoupling and z-gradients, and a 1.02 T Magritek SpinSolve 40 Carbon desktop spectrometer operating at 43.4 MHz for proton. Measurements of
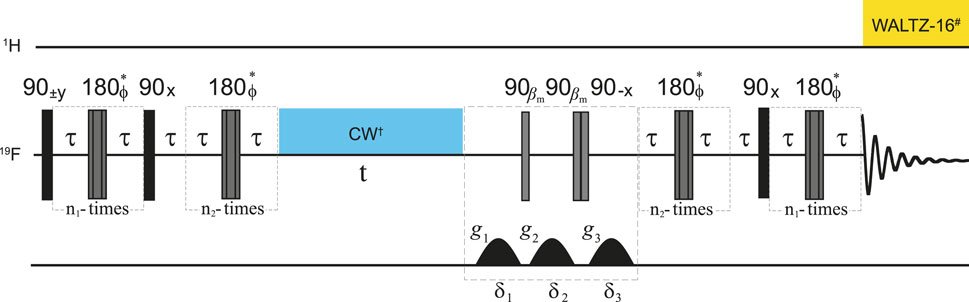
Figure 1. Pulse sequence used to measure the lifetime of singlet order in 19
The parameters occurring in the pulse sequence in Figure 1 are reported in Table 2.

Table 2. The parameters used to run the pulse sequence in Figure 1.
3 Results and discussion
3.1 Sample A
The 19F-NMR spectrum of Sample A, taken at 9.4 T and 25°C, is reported in Figure 2A and shows a complex multiplet. The spectrum is shown using a frequency scale in Hz and has been centred at 0 Hz for simplicity (the chemical shift at the centre of the multiplet is −101.7 ppm). Based on visual inspection of the linewidth, it is clear that the fluorine nuclei are strongly coupled to each other and weakly coupled to possibly all other protons of the aromatic rings. This is easily confirmed by the 19F-{1
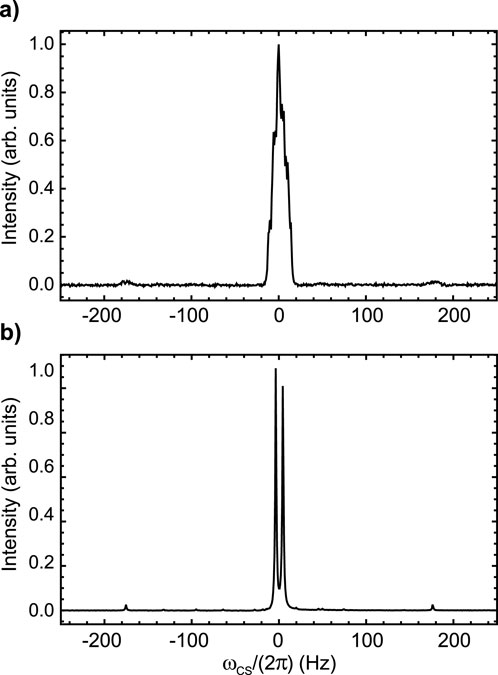
Figure 2. 19F NMR spectra of Sample A taken at 9.4 T and 25°C without (A) and with (B) proton decoupling. The intensity is in arbitrary units and the peak has been centred at 0 Hz for simplicity (the chemical shift at the centre of the multiplet is −101.7 ppm).
It is therefore clear that the 19F spin pair is not isolated from the other spins in the molecule and all coupled spins should be considered in the discussion. The presence of scalar coupling to neighbouring protons may give rise to three types of problem: i) The spin Hamiltonian contains cross terms that connect the 19F singlet state to the triplet states. In fact, 19F singlet spin order may be not a good eigenoperator of the Hamiltonian superoperator; ii) The scalar couplings between 19F and 1H nuclei can give rise to relaxation via a scalar coupling of the second kind mechanism (S2K), which will shorten the lifetime of singlet order proportionally to the magnitude of the spin couplings involved and the size of the proton
Fortunately, the large value of
With this in mind, we measured the relaxation decay constant of 19F longitudinal spin order (

Table 3. The19F
Given that the non-bonding nature of the 19F-19F scalar coupling relies on partial orbital overlap, we decided to explore whether the magnitude of the
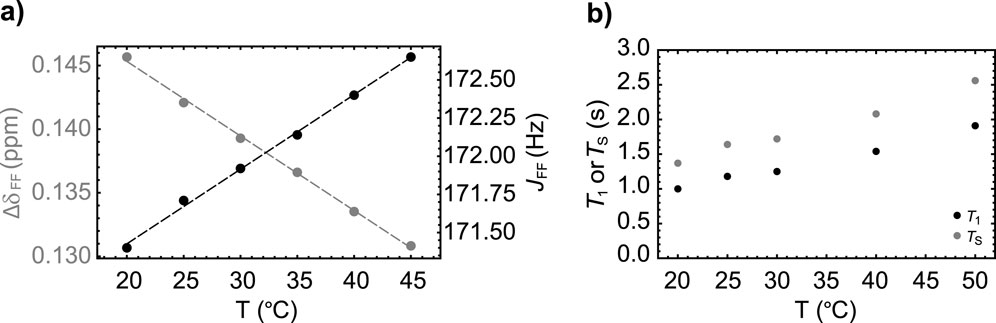
Figure 3. (A) The temperature dependence of the chemical shift difference between the two fluorine nuclei (gray circles) and their mutual scalar coupling constant (black circles) in Sample A. Dashed lines are best fit to the experimental points. (B) The variation of
3.2 Sample B
In order to remove dipolar interactions with the neighbouring proton, we synthesised molecule II (prepared as Sample B, see Materials and Methods), which is an analogue of molecule I with the aromatic ring hydrogens substituted with deuteriums so to scale down the size of the 19F dipolar couplings by a factor of about six (the ratio between hydrogen and deuterium gyromagnetic ratios). The 19F-NMR spectrum of Sample B, taken at 9.4 T and 25°C, is reported in Figure 4, where a set of asterisks mark the four peaks of the expected AB pattern for the two fluorine peaks in the sample (couplings to neighbouring deuterium nuclei fall within the linewidth). Other than these four marked peaks, the spectrum shows a series of other minor peaks that are due to the trace isotopologues containing mixtures of hydrogen and deuterium atoms (see Section 2.1 and supplementary information).
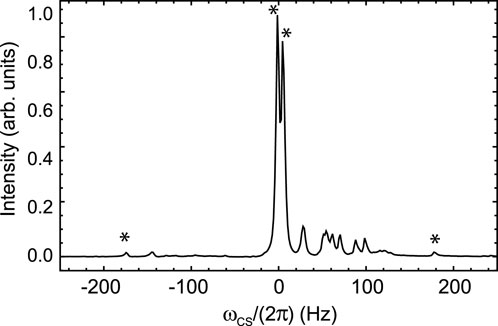
Figure 4. 19F NMR spectra of Sample B taken at 9.4 T and 25°C. The intensity is in arbitrary units and the peak has been centred at 0 Hz for simplicity (the chemical shit at the centre of the AB pattern is −101.8 ppm). The expected AB pattern arising from the 19F spin pair is marked with asterisks. All unmarked peaks arise from isotopomers containing protons in place of deuterium.
A measurement of the longitudinal order decay constant at 9.4 T and 25°C on this sample resulted in a rather short value of
As done for Sample A, we report the results of a series of experiments to study the temperature dependence of the spin system parameters (Figure 5A) and the relaxation decay constants
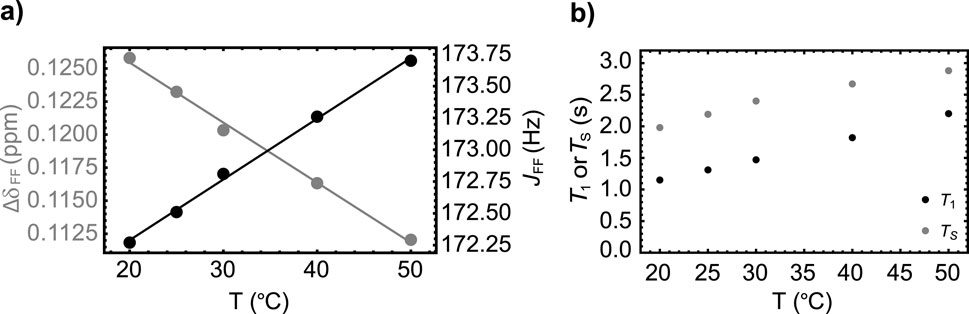
Figure 5. (A) The variation with temperature of the chemical shift difference between the two fluorine nuclei (gray circles) and their mutual scalar coupling constant (black circles) in Sample B. Dashed lines are best fit to the experimental points. (B) The variation of
3.3 Sample C
With the aim of removing any immediate nuclear spin coupled to the fluorine atoms, we synthesised a third molecule (molecule III in Scheme 1, prepared as Sample C as described in Materials and Methods) where we aimed to replace the closest spin to the two fluorine nuclei with -
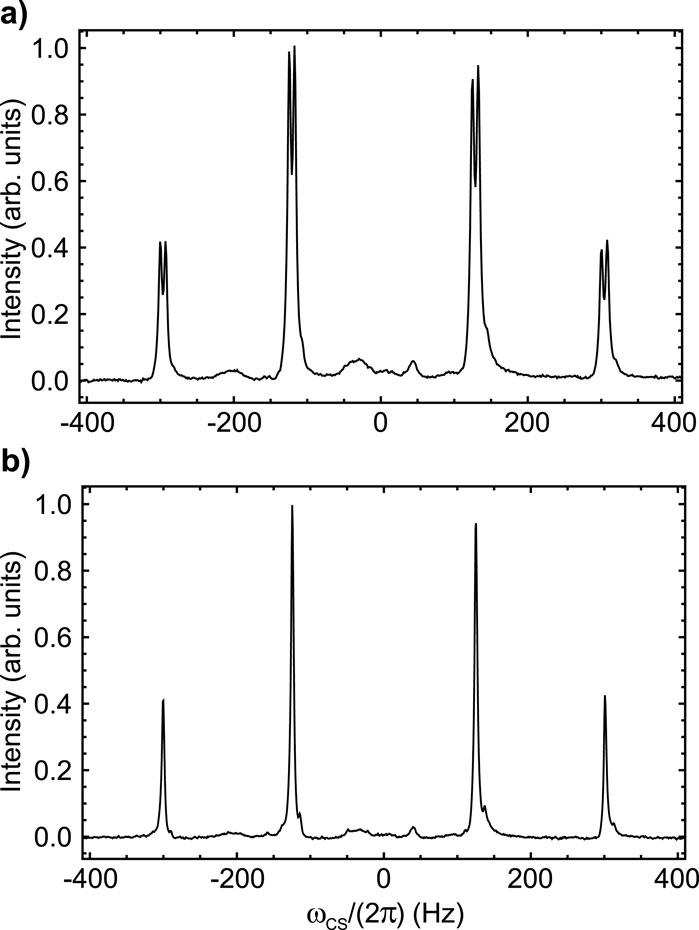
Figure 6. 19F NMR spectra of Sample C taken at 9.4 T and 25°C without (A) and with (B) proton decoupling. The intensity is in arbitrary units and the peak has been centred at 0 Hz for simplicity (the chemical shit at the centre of the multiplet is −124.6 ppm).
At this point, we found it very interesting to prepare 19F singlet spin order in the low magnetic field of a desktop spectrometer operating at 1.02 T, where the chemical shift frequency difference is only 42.1 Hz and the two fluorine nuclei would remain strongly-coupled. For this, we coded the pulse sequence in Figure 1 into a Spinsolve and measured both
4 Conclusion
We have demonstrated that the strong scalar coupling between 19F spin pairs separated by many bonds, but constrained in spatial proximity, can be deployed to prepare long-lived singlet spin order. In proof of principle experiments conducted on three newly-synthesized molecules containing spatially-constrained 19F pairs that display very large scalar couplings of “through-space” nature, we have shown a 13-fold extension in spin memory. The work has evidenced a significant contribution of chemical shift anisotropy to singlet order relaxation and hinted at the possibility of other complementary mechanisms such as scalar coupling of the first kind or the presence of molecular aggregation. A full understanding of these relaxation contributions requires supplementary experiments and numerical simulations that are the subject of future work. For example, it would be interesting to run field-cycling experiments to measure
Data availability statement
CCDC 2390768-2390769 contains the supplementary crystallographic data for this paper. The data can be obtained free of charge from The Cambridge Crystallographic Data Centre via www.ccdc.cam.ac.uk/structures.
Author contributions
GP: Conceptualization, Formal Analysis, Investigation, Methodology, Resources, Supervision, Validation, Writing–original draft, Writing–review and editing. DY: Formal Analysis, Investigation, Writing–review and editing. CE: Methodology, Software, Writing–review and editing. GT: Data curation, Formal Analysis, Investigation, Writing–review and editing. ST: Conceptualization, Formal Analysis, Investigation, Methodology, Resources, Supervision, Writing–review and editing.
Funding
The author(s) declare that financial support was received for the research, authorship, and/or publication of this article. Financial support was provided by EPSRC UK grant numbers EP/V047663/1, EP/X525807/1 and EP/W021129/1.
Acknowledgments
The authors would like to thank Dr. N. J. Wells for providing access to the 400 MHz NMR spectrometer equipped with a fluorine probe.
Conflict of interest
Author CE was employed by Magritek GmbH.
The remaining authors declare that the research was conducted in the absence of any commercial or financial relationships that could be construed as a potential conflict of interest.
The author(s) declared that they were an editorial board member of Frontiers, at the time of submission. This had no impact on the peer review process and the final decision.
Generative AI statement
The author(s) declare that no Generative AI was used in the creation of this manuscript.
Publisher’s note
All claims expressed in this article are solely those of the authors and do not necessarily represent those of their affiliated organizations, or those of the publisher, the editors and the reviewers. Any product that may be evaluated in this article, or claim that may be made by its manufacturer, is not guaranteed or endorsed by the publisher.
Supplementary material
The Supplementary Material for this article can be found online at: https://www.frontiersin.org/articles/10.3389/fchem.2024.1511720/full#supplementary-material
Footnotes
1The 19F-19F scalar coupling can be quite large in vicinal 19F pairs but then the 19F nuclei are often chemically equivalent and therefore singlet order cannot be accessed.
2CCDC #2390769 (molecule III), #2390768 (11).
References
Ahuja, P., Sarkar, R., Vasos, P. R., and Bodenhausen, G. (2007). Molecular properties determined from the relaxation of long-lived spin states. J. Chem. Phys. 127, 134112. doi:10.1063/1.2778429
Bakhmutov, V. I., Galakhov, M. V., and Fedin, E. I. (1985). Through-space 19F,19F spin—spin coupling constants in some fluoroallyl cations. Magnetic Reson. Chem. 23, 971–972. doi:10.1002/mrc.1260231117
Cavadini, S., Dittmer, J., Antonijevic, S., and Bodenhausen, G. (2005). Slow diffusion by singlet state nmr spectroscopy. J. Am. Chem. Soc. 127, 15744–15748. doi:10.1021/ja052897b
Dolbier, W. R. J. (2016). Guide to fluorine NMR for organic chemists. John Wiley Sons, Ltd. doi:10.1002/9781118831106
Dumez, J. N., Hill-Cousins, J. T., Brown, R. C., and Pileio, G. (2014). Long-lived localization in magnetic resonance imaging. J. Magnetic Reson. 246, 27–30. doi:10.1016/j.jmr.2014.06.008
Elliott, S. J., Bengs, C., Brown, L. J., Hill-Cousins, J. T., O’Leary, D. J., Pileio, G., et al. (2019). Nuclear singlet relaxation by scalar relaxation of the second kind in the slow-fluctuation regime. J. Chem. Phys. 150, 064315. doi:10.1063/1.5074199
Ernst, L., and Ibrom, K. (1995). A new quantitative description of the distance dependence of through-space 19F,19F spin-spin coupling. Angewandte Chemie Int. Ed. Engl. 34, 1881–1882. doi:10.1002/anie.199518811
Ernst, L., Ibrom, K., Marat, K., Mitchell, R. H., Bodwell, G. J., and Bushnell, G. W. (1994). syn-ar,ar’-difluorometacyclophanes: strong 19F,19F spin-spin interactions transmitted through space. Chem. Berichte 127, 1119–1124. doi:10.1002/cber.19941270623
Gerig, J. T. (2001). Fluorine nmr. Available at: https://api.semanticscholar.org/CorpusID:20942460.
Hahn, E. L. (1949). An accurate nuclear magnetic resonance method for measuring spin-lattice relaxation times. Phys. Rev. 76, 145–146. doi:10.1103/PhysRev.76.145
Harbor-Collins, H., Sabba, M., Bengs, C., Moustafa, G., Leutzsch, M., and Levitt, M. H. (2024). NMR spectroscopy of a 18O-labeled rhodium paddlewheel complex: isotope shifts, 103Rh–103Rh spin–spin coupling, and 103Rh singlet NMR. J. Chem. Phys. 160, 014305. doi:10.1063/5.0182233
Kiryutin, A. S., Rodin, B. A., Yurkovskaya, A. V., Ivanov, K. L., Kurzbach, D., Jannin, S., et al. (2019). Transport of hyperpolarized samples in dissolution-dnp experiments. Phys. Chem. Chem. Phys. 21, 13696–13705. doi:10.1039/C9CP02600B
Korenchan, D., Lu, J., Sabba, M., Dagys, L., Brown, L., Levitt, M., et al. (2022). Limits of the quantum cognition hypothesis: 31P singlet order lifetimes of pyrophosphate from experiment and simulation. ChemRxiv. doi:10.26434/chemrxiv-2022-7cvbc-v2
Mallory, F. B., Mallory, C. W., Butler, K. E., Lewis, M. B., Xia, A. Q., Luzik, E. D., et al. (2000). Nuclear spin-spin coupling via non-bonded interactions. 8.1 the distance dependence of through-space fluorine-fluorine coupling. J. Am. Chem. Soc. 122, 4108–4116. doi:10.1021/ja993032z
Mallory, F. B., Mallory, C. W., and Fedarko, M. C. (1974). Substituent effects on through-space fluorine-19-fluorine-19 coupling in the 1,8-difluoronaphthalene system. J. Am. Chem. Soc. 96, 3536–3542. doi:10.1021/ja00818a029
Mallory, F. B., Mallory, C. W., Grant, D. M., and Harris, R. K. (1996). Encyclopedia of nuclear magnetic resonance. J. Wiley and Sons 3, 1491–1501.
Mallory, F. B., Mallory, C. W., and Ricker, W. M. (1985). Nuclear spin-spin coupling via nonbonded interactions. 4. fluorine-fluorine and hydrogen-fluorine coupling in substituted benzo[c]phenanthrenes. J. Org. Chem. 50, 457–461. doi:10.1021/jo00204a006
Melchiorre, G., Giustiniano, F., Rathore, S., and Pileio, G. (2023). Singlet-assisted diffusion-nmr (sad-nmr): extending the scope of diffusion tensor imaging via singlet nmr. Front. Chem. 11, 1224336. doi:10.3389/fchem.2023.1224336
Murai, T., Xing, Y., Kurokawa, M., Kuribayashi, T., Nikaido, M., Elboray, E. E., et al. (2022). One-pot preparation of (nh)-phenanthridinones and amide-functionalized [7]helicene-like molecules from biaryl dicarboxylic acids. J. Org. Chem. 87, 5510–5521. doi:10.1021/acs.joc.1c02769
Petrakis, L., and Sederholm, C. H. (1961). NMR fluorine-fluorine coupling constants in saturated organic compounds. J. Chem. Phys. 35, 1243–1248. doi:10.1063/1.1732030
Pileio, G. (2011). Singlet state relaxation via intermolecular dipolar coupling. J. Chem. Phys. 134, 214505. doi:10.1063/1.3596379
Pileio, G. (2017). Singlet nmr methodology in two-spin-1/2 systems. Prog. Nucl. Magnetic Reson. Spectrosc. 98-99, 1–19. doi:10.1016/j.pnmrs.2016.11.002
Pileio, G., Bowen, S., Laustsen, C., Tayler, M. C. D., Hill-Cousins, J. T., Brown, L. J., et al. (2013). Recycling and imaging of nuclear singlet hyperpolarization. J. Am. Chem. Soc. 135, 5084–5088. doi:10.1021/ja312333v
Pileio, G., Dumez, J. N., Pop, I. A., Hill-Cousins, J. T., and Brown, R. C. (2015). Real-space imaging of macroscopic diffusion and slow flow by singlet tagging mri. J. Magnetic Reson. 252, 130–134. doi:10.1016/j.jmr.2015.01.016
Pileio, G., Hill-Cousins, J. T., Mitchell, S., Kuprov, I., Brown, L. J., Brown, R. C. D., et al. (2012). Long-lived nuclear singlet order in near-equivalent 13c spin pairs. J. Am. Chem. Soc. 134, 17494–17497. doi:10.1021/ja3089873
Pileio, G., and Levitt, M. H. (2007). J-stabilization of singlet states in the solution nmr of multiple-spin systems. J. Magnetic Reson. 187, 141–145. doi:10.1016/j.jmr.2007.03.019
Pileio, G., and Levitt, M. H. (2009). Theory of long-lived nuclear spin states in solution nuclear magnetic resonance. II. Singlet spin locking. J. Chem. Phys. 130, 214501. doi:10.1063/1.3139064
Pileio, G., and Ostrowska, S. (2017). Accessing the long-time limit in diffusion nmr: the case of singlet assisted diffusive diffraction q-space. J. Magnetic Reson. 285, 1–7. doi:10.1016/j.jmr.2017.10.003
Saika, A., and Gutowsky, H. S. (1956). An unusual fluorine magnetic resonance multiplet. J. Am. Chem. Soc. 78, 4818–4819. doi:10.1021/ja01599a081
Salvi, N., Buratto, R., Bornet, A., Ulzega, S., Rentero Rebollo, I., Angelini, A., et al. (2012). Boosting the sensitivity of ligand-protein screening by nmr of long-lived states. J. Am. Chem. Soc. 134, 11076–11079. doi:10.1021/ja303301w
Sarkar, R., Vasos, P. R., and Bodenhausen, G. (2007). Singlet-state exchange nmr spectroscopy for the study of very slow dynamic processes. J. Am. Chem. Soc. 129, 328–334. doi:10.1021/ja0647396
Servis, K. L., and Fang, K. N. (1968). Nuclear magnetic resonance studies of 19F-19 spin-spin coupling. 1-substituted 4,5-difluoro-8-methylphenanthrenes. J. Am. Chem. Soc. 90, 6712–6717. doi:10.1021/ja01026a027
Stevanato, G., Hill-Cousins, J. T., Häkansson, P., Roy, S. S., Brown, L. J., Brown, R. C. D., et al. (2015). A nuclear singlet lifetime of more than one hour in room-temperature solution. Angew. Chem. Int. Ed. 54, 3740–3743. doi:10.1002/anie.201411978
Tourell, M. C., Pop, I. A., Brown, L. J., Brown, R. C. D., and Pileio, G. (2018). Singlet-assisted diffusion-nmr (sad-nmr): redefining the limits when measuring tortuosity in porous media. Phys. Chem. Chem. Phys. 20, 13705–13713. doi:10.1039/C8CP00145F
Keywords: long-lived spin states, nuclear singlet spin order, through-space scalar coupling, NMR, fluorine NMR
Citation: Pileio G, Yamano D, Eccles CD, Tizzard GJ and Thompson S (2025) Singlet spin order in spin pairs coupled via non-bonded interactions. Front. Chem. 12:1511720. doi: 10.3389/fchem.2024.1511720
Received: 15 October 2024; Accepted: 16 December 2024;
Published: 30 January 2025.
Edited by:
Paul Vasos, University of Bucharest, RomaniaReviewed by:
Michael Tayler, The Institute of Photonic Sciences (ICFO), SpainRiddhiman Sarkar, Helmholtz Association of German Research Centres (HZ), Germany
Copyright © 2025 Pileio, Yamano, Eccles, Tizzard and Thompson. This is an open-access article distributed under the terms of the Creative Commons Attribution License (CC BY). The use, distribution or reproduction in other forums is permitted, provided the original author(s) and the copyright owner(s) are credited and that the original publication in this journal is cited, in accordance with accepted academic practice. No use, distribution or reproduction is permitted which does not comply with these terms.
*Correspondence: Giuseppe Pileio, Zy5waWxlaW9Ac290b24uYWMudWs=; Sam Thompson, c3QzYTE1QHNvdG9uLmFjLnVr