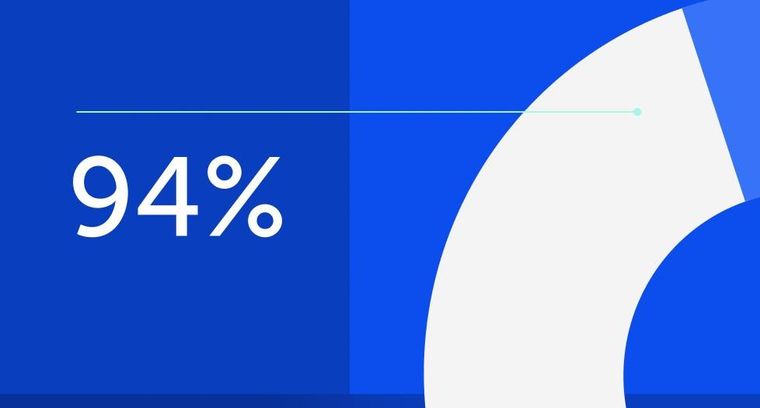
94% of researchers rate our articles as excellent or good
Learn more about the work of our research integrity team to safeguard the quality of each article we publish.
Find out more
ORIGINAL RESEARCH article
Front. Chem., 22 March 2022
Sec. Analytical Chemistry
Volume 10 - 2022 | https://doi.org/10.3389/fchem.2022.873187
This article is part of the Research Topic2D Nanoarchitectures for Sensing/Biosensing ApplicationsView all 12 articles
The rational design and construction of high-performance flexible electrochemical sensors based on hierarchical nanostructure functionalized microelectrode systems are of vital importance for sensitive in situ and real-time detection of biomolecules released from living cells. Herein, we report a novel and facile strategy to synthesize a new kind of high-performance microelectrode functionalized by dual nanozyme composed of rime-like Cu2(OH)3NO3 wrapped ZnO nanorods assembly [Cu2(OH)3NO3@ZnO], and explore its practical application in electrochemical detection of hydrogen peroxide (H2O2) released from living cells. Benefiting from the merits of the unique hierarchical nanohybrid structure and high catalytic activities, the resultant Cu2(OH)3NO3@ZnO-modified AGF microelectrode shows remarkable electrochemical sensing performance towards H2O2 with a low detection limit of 1 μM and a high sensitivity of 272 μA cm−2 mM−1, as well as good anti-interference capability, long-term stability, and reproducibility. These properties enabled the proposed microelectrode-based electrochemical platform to be applied for in situ amperometric tracking of H2O2 released from different types of human colon cells, thus demonstrating its great prospect as a sensitive cancer cell detection probe for the early diagnosis and management of various cancer diseases.
Since cancer is the second leading cause of death globally, there has always been an increasing research interest in developing efficient techniques for early cancer diagnosis and treatment to circumvent the challenges being faced in clinical practices (Labib et al., 2016; Lin et al., 2016; Asif et al., 2018b). Hydrogen peroxide (H2O2), one of the most common reactive oxygen species, performs as a critical component within biological systems (Lippert et al., 2011; Aziz et al., 2019a). The level of H2O2 in human body can be regarded as a versatile biomarker for early diagnosis of various diseases such as cancer (Xiao et al., 2016). For effective and accurate monitoring of the versatile biomarkers associated with cancer, electrochemical biosensors have comprehensive advantages of fast response, low detection limit, high sensitivity, good reproducibility, easy operation, and the ability to be miniaturized for online, and in vivo analysis (Sun et al., 2015; Sun et al., 2016; Zhao et al., 2021). Moreover, the development of wearable and implantable electrochemical sensing systems for continuous monitoring of the H2O2concentration in the human body has shown greater prospects in clinical diagnosis (Wang L. et al., 2018; Asif et al., 2022). Therefore, it is of great significance to construct structural rigidity, high mechanical strength functionalized microelectrodes, which can be integrated into wearable flexible electrochemical sensors to achieve fast, real-time, and high-sensitivity detection (Aziz et al., 2021b).
The development of advanced electrochemical sensors necessitates the design and construction of freestanding electrode system with good mechanical strength and high flexibility (Aziz et al., 2021a), which can be rolled up for use as well as good sensing performance in cancer cell detection. In this regard, graphene fiber (GF), a new type of carbon fiber assembled from graphene nanosheets, possesses one-dimensional microstructure with good flexibility, mechanical strength, high electronic conductivity, and excellent electrochemical stability (Xin et al., 2015; Huang et al., 2018; Xin et al., 2019). The prepared GF integrates the high electrical conductivity of graphene nanosheets and the unique mechanical properties of its macroscopic fiber structure, which has broad application prospects in flexible energy storage/conversion devices, wearable devices, artificial intelligence, etc. (Zhang et al., 2016; Huang et al., 2018). In particular, the intrinsic advantages GF microelectrode, such as adjustable active sites, high signal-to-noise ratio, and good biological compatibility, as well as immobilization of enzyme, enable its application in electrochemical sensing fields (Ding et al., 2016; Chen et al., 2019; Zeng et al., 2019). However, among different kinds of electrochemical sensors, GF microelectrode-based electrochemical biosensor is still in its infancy, so rational design of nanohybrid GF with active components is important for improving the comprehensive performance of electrochemical sensors for the detection of biomolecules. Nanozyme is a class of nanomaterials with enzyme-like characteristics (Wang H. et al., 2018; Wu et al., 2019). With the recent advance of nanotechnology with biology, a variety of nanozymes, such as carbon-based nanomaterials (Ji et al., 2021; Li et al., 2021), transition metal dichalcogenides/peroxides/oxides nanosheets (Asif et al., 2018a; Asif et al., 2019; Huang et al., 2019), noble metal nanoparticles (NPs) (Shen et al., 2015; Xu et al., 2018), and their hybrids (Zhang et al., 2019), have been discovered to possess unique enzyme-mimic catalytic activities and used in the biomedicine or bioanalysis by virtue of their reasonable stability, low cost, mass production, and long-term storage properties that are superior to nature enzymes. In the past few years, tremendous research efforts have been devoted to developing nanozyme-modified GF microelectrodes, which have been used in electrochemical detection of versatile biomarkers, such as adrenaline (Zeng et al., 2019), glucose (Chen et al., 2019), dopamine (Cai et al., 2016; Aziz et al., 2019b), and H2O2 (Peng et al., 2018; Zhao et al., 2020; Guo et al., 2021). Although considerable progress has been made in this field, it is still a challenge for the development of high-performance functionalized GF-based microelectrodes with optimized microstructural configurations by judiciously selecting electroactive species and rationally designing the composition and structure of nanozyme for continuous detection of biological samples.
In this work, we have developed an activated graphene fiber (AGF) modified with MOF-mediated rime-like hierarchical nanozyme as flexible and biocompatible microelectrode for in situ electrochemical detection of H2O2 in human colon cells. As far as we know, this has never been reported previously. As shown in Figure 1, the freestanding and flexible AGF is synthesized by wet-spinning using graphene oxide as the structural units and ionic liquid (IL) as coagulation bath to introduce heteroatoms (Cheng et al., 2015). The introduction of heteroatoms into the graphene materials not only possesses superior charge mobility to deliver high electrochemical activity, but also provides abundant active sites for the accessibility of substrate molecules and anchoring extrinsic functional species. To improve its catalytic activity, a metal-organic framework (MOF)-mediated rime-like hierarchical nanozyme [i.e., Cu2(OH)3NO3@ZnO] is assembled on heteroatom-doped GF (Cui et al., 2016). Specifically, ZnO nanorods are grown on AGF via a typical solvothermal method. ZIF-8 is then in situ grown on ZnO nanorods by the impregnation method to obtain ZIF-8@ZnO/AGF. The Cu2(OH)2(NO3) is bonded to ZnO/AGF by chemical etching of ZIF-8, named, Cu2(OH)3NO3@ZnO/AGF. Benefitting from the synergistic contributions of dual nanozymatic activity of rime-like hierarchical Cu2(OH)3NO3@ZnO as well as unique structural and electrical properties, the resultant rime-like hierarchical Cu2(OH)3NO3@ZnO-decorated heteroatom-doped GF [Cu2(OH)3NO3@ZnO/AGF] microelectrode demonstrated significantly improved sensing performances to H2O2 detection, with a low detection limit (LOD) of 1 µM (S/N = 3) and a sensitivity of 272 μA cm−2 mM−1. These characteristics combined with its favorable anti-interference ability, high reducibility, and long-term stability, as well as good biocompatibility, enable a Cu2(OH)3NO3@ZnO/AGF microelectrode-based electrochemical biosensor to be used for real-time tracking of H2O2 released from different live human colon cells, which can act as a sensitive probe to distinguish colon cancer cells and normal colon epithelial cells. Thus, our strategy for the development of high-performance electrochemical biosensor coupled with recent advance in GF microelectrode and nanozyme opens up a new avenue for facile and efficient detection of possible disease-related clinical specimens, which is of great significance for the early diagnosis of various diseases in clinic practice.
FIGURE 1. Fabrication procedure of a rime-like Cu2(OH)3NO3@ZnO/AGF microelectrode for live cancer cell detection.
Sulfuric acid (H2SO4, 98%), hydrochloric acid (HCl, 37%), potassium nitrate (KNO3), potassium permanganate (KMnO4), hydrogen peroxide (H2O2, 30%), ethanol, zinc nitrate hexahydrate (Zn(NO3)2·6H2O), zinc acetate dihydrate (Zn(CH3COO)2)·2H2O, hexamethylenetetramine (C6H12N4), and copper nitrate trihydrate (Cu(NO3)2·3H2O) were purchased from the Sinopharm Chemical Reagent Co., Ltd. 1-Butyl-3-methylimidazolium tetrafluoroborate ([BMIM][BF4]), hydroiodic acid (HI, 48%), and worm-like expanded graphite powder (50 mesh number) were purchased from Shanghai Aladdin Biochemical Technology Co. Ltd. Guanine (G), adenine (A), cytosine (C), uracil (U), ascorbic acid (AA), D(+)-glucose (Glu), uric acid (UA), and dopamine (DA) were purchased from the Macklin Co. Ltd. N-formylmethionyl-leucyl-phenyl-alanine (fMLP, ≥99.5%) was obtained from Sigma-Aldrich (United States). All chemicals and reagents are of analytical grade and used without any further purification.
SEM images were acquired on Nova NanoSEM 450 (10 kV). TEM images were examined by Tecnai G2-F20 (200 kV). X-ray photoelectron spectroscopy (XPS) measurements were carried out on a Perkin-Elmer model PHI 5600 XPS system; all peaks were standardized to C 1s line at 284.6 eV correction. Powder x-ray diffraction (PXRD) was performed on a Rigaku D/max-IIIA diffractometer (Cu Kα, λ = 1.54056 Å) at room temperature. Electrochemical experiments were conducted on a CHI 760E electrochemical workstation (Shanghai CH Instruments Co., China).
Graphene oxide (GO) was first synthesized from worm-like expanded graphite powder based on a modified Hummer’s method (Xu et al., 2016). The as-obtained GO suspension was centrifuged twice at 14,000 rpm for 30 min to obtain concentrated GO suspension with a mass concentration of 20 mg ml−1. For wet spinning of GO fiber, the concentrated GO suspension was transferred into a 5-ml syringe connected by polyetheretherketone tube with a glass capillary (inner diameter of 500 μm) at the other end (Supplementary Figure S1). It was pumped into [BMIM][BF4] IL coagulation bath solution at a rate of 450 μl min−1. Subsequently, GO fibers were immersed into 30 ml of HI solution and kept at 80°C for 12 h to be reduced. Finally, they were washed successively with water and methanol to remove the HI and the iodine and dried at 60°C for 12 h.
Reduced GF was placed in 30 ml of 30% H2O2 solutions at 70°C for 30 min and then washed with ultrapure water to obtain AGF. For the synthesis of ZnO nanorod-modified graphene fibers (ZnO/AGF), Zn(NO3)2 6H2O (59.50 mg) and hexamethylenetetramine (28.04 mg) were dissolved in 40 ml of deionized water and stirred for 10 min. AGF was placed in a PTFE autoclave containing the above solution for 12 h at 90°C. Then, the obtained fibers were placed into a high-temperature tube furnace, and heated to 400°C at a heating rate of 5°C min−1 for 40 min to obtain ZnO/AGF. The as-synthesized ZnO/AGF was incubated into 2-methylimidazole solution (1.5 M, 30 ml) for 24 h for the in situ growth of ZIF-8 (ZIF-8@ZnO/AGF). Then, the ZIF-8@ZnO/AGF was soaked in Cu(NO3)2 solution (0.5 M) for 5–10 min to obtain Cu2(OH)3NO3@ZnO/AGF.
Human colon cells SW-48, NCM-460, and HCT-116 were obtained from the American Type Culture Collection (ATCC, Manassas, VA, United States). Cells were seeded in a 6-well plate for 24 h, and grown in Dulbecco’s modified eagle medium containing 10% fetal bovine serum supplemented with 100 units ml−1 penicillin and 100 mg ml−1 streptomycin in an incubator at 37°C with 5% CO2. The incubation solution was removed after growing to 90% confluence, then washed with PBS for three times to collect cells. The number of cells was counted by a hemocytometer. For real-time measurements of H2O2 in live cells, a three-electrode system was located on the plate containing 3 ml of PBS with the cell density of 5 × 106 cells ml−1, and the amperometric responses of different live cells were recorded.
Nikon Ti-U microscope was used to record the color changes of fluorescent dichlorofluorescein. The microscope was equipped with a CSU-X1 spinning-disk confocal unit (Yokogawa) and an EM-charge-coupled device camera (iXon+; Andor). The chronoamperometry (i-t) measurement was performed at an applied potential of −0.8 V vs. Ag/AgCl. After the baseline was stable, 10 μl of fMLP (1 mg ml−1) was injected into wells (1 ml) of different types of colon cell, and then the responding currents were recorded to monitor the concentration of H2O2.
For the preparation of hierarchical nanohybrid microelectrode, the flexible GF has been used as the freestanding electrode substrate. As shown in Figures 2A,B, it can be observed that GF consists of stacked graphene nanosheets neatly aligned along the axis to form a layered structure with a uniform diameter of ∼50 μm and wrinkled structure (Figure 2B). The wrinkled structure endows the GF with a typical porous microstructure, which provides a larger specific surface area. For the subsequent growth of nanohybrids, the pristine GF is oxidized by chemical methods to introduce the desired functional groups on its surface to enhance its hydrophilicity. The ZnO is synthesized by a hydrothermal method with a rod-like morphology (Figure 2C), endowing ZnO/AGF with a larger surface area than bare AGF, which is conducive to easier loading of other active materials in subsequent derivatization steps. After the coordination of 2-methylimidazole with zinc ions, the ZnO nanorods are in situ converted to ZIF-8 with rhombic dodecahedron morphology. The average particle size of ZIF-8 is about 3 μm with smooth and uniform surface (Figure 2D). Then, ZIF-8 is used as a mediator to facilitate the growth of Cu2(OH)3NO3 nanozyme on ZnO nanorods. As shown in Figures 2E–H, ZIF-8 with rhombic dodecahedron is etched into irregular particles, while the inner part of the particles still has a rod-shaped ZnO skeleton. The morphology of Cu2(OH)3NO3@ZnO/AGF resembles rime in natural landscape (Supplementary Figure S2). The nanostructure of Cu2(OH)3NO3@ZnO/AGF has also been investigated by transmission electron microscopy (TEM). Figure 2I reveals that Cu2(OH)3NO3@ZnO/AGF possesses ultrathin nanosheet morphology. The ultrathin nanosheet morphology further endows the microelectrode with more exposed active sites and high electrocatalytic activity towards H2O2. High-resolution TEM (HRTEM) image shows that the lattice fringe with a crystal spacing of 0.248 and 0.153 nm matches well with the crystal plane ZnO (101) and Cu2(OH)3NO3 (040), respectively (Figure 2J). The corresponding selected area electron diffraction (SAED) pattern presents some clear bright spots, further confirming the composition of Cu2(OH)3NO3@ZnO/AGF (Figure 2K). The HAADF-STEM and the corresponding energy-dispersive spectroscopy (EDS) mappings demonstrate the distribution of C, N, O, Cu, and Zn in Cu2(OH)3NO3@ZnO/AGF (Figures 2L–O), further confirming the successful synthesis of Cu2(OH)3NO3@ZnO/AGF.
FIGURE 2. SEM images of (A) cross-sectional view and (B) front view of the AGF; SEM images of (C) ZnO/AGF and (D) ZIF-8@ZnO/AGF; SEM images of (E–H) Cu2(OH)3NO3@ZnO/AGF at different magnifications; TEM image of (I) Cu2(OH)3NO3@ZnO/AGF; (J) HRTEM image and corresponding (K) SAED pattern of Cu2(OH)3NO3@ZnO/AGF; (L–O) EDS mappings of C, Cu, N, O, and Zn elements in Cu2(OH)3NO3@ZnO/AGF.
The composition of hierarchical nanohybrids has been investigated by powder x-ray diffraction (PXRD) analysis. Figure 3A shows that the PXRD patterns of AGF exhibit a broad peak at 26.4°, corresponding to the crystallographic planes of C (002). After decorating with ZnO, four distinct characteristic diffraction peaks are observed at 31.7°, 34.4°, 36.2°, and 47.4°, corresponding to the (100), (002), (101), and (102) planes of ZnO (PDF#36-1451) (Anila et al., 2021). A series of peaks with weak intensity appeared at 5°–20° in the ZIF-8@ZnO/AGF pattern, which can be attributed to the diffraction peaks of ZIF-8. The PXRD pattern of Cu2(OH)3NO3@ZnO/AGF exhibits new peaks at 12.8°, 25.7°, 33.6°, 35.3°, 36.4°, 39.8°, 43.5°, and 49.2°, corresponding to the crystallographic planes of (001), (002), (−201), (−121), (121), (−202), (122), and (−131) of Cu2(OH)3NO3 (PDF#15-0014) (Supplementary Figure S3), which are well consistent with the HRTEM characterization (Munyemana et al., 2020). Thermogravimetric analysis (TGA) under N2 atmosphere provides stability information of Cu2(OH)3NO3@ZnO/AGF, which shows a two-step weight loss (Supplementary Figure S4). TG curve shows a sharp weight loss beyond 225°C associated with the decomposition of Cu2(OH)3NO3, followed by a long plateau until 700°C. The mass loss at 700°C corresponds to the reduction of ZnO by carbon at high temperature to generate carbon dioxide or carbon monoxide. These results demonstrate the successful synthesis of hierarchical nanohybrids on AGF.
FIGURE 3. (A) PXRD patterns of AGF, ZnO/AGF, ZIF-8@ZnO/AGF, and Cu2(OH)3NO3@ZnO/AGF. High-resolution XPS spectra of (B) C 1s, (C) N 1s, (D) O 1s, (E) Cu 2p, and (F) Zn 2p regions in Cu2(OH)3NO3@ZnO/AGF.
The surface chemical states of different elements in Cu2(OH)3NO3@ZnO/AGF have further been verified by x-ray photoelectron spectroscopy (XPS) analysis. The survey spectrum exhibits five predominant peaks at 285, 400, 533, 930, and 1,030 eV, assigned to C 1s, N 1s, O 1s, Cu 2p, and Zn 2p, respectively (Supplementary Figure S5). The surface atomic ratio of C:N:O:Cu:Zn is 29.87:8.42:44.46:10.59:6.65. The corresponding fitting curves of these elements are depicted in Figures 3B–F. The C 1s spectra can be deconvoluted into four components corresponding to different functional groups. The peaks at 284.8, 285.8, 286.4, and 288.6 eV are designated as C-C, C-N, C-O, and C=O, respectively. The high-resolution spectrum of N 1s shows a strong intensity of peak at 408.0 eV and a smaller intensity of peak in the range of 399–402 eV. The strong intensity of peak is assigned to NO3− and the smaller intensity of peak region is deconvoluted into four peaks at 399.2, 400.0, 401.1, and 401.7 eV, which are further assigned to pyridinic N, pyrrolic N, graphitic N, and N-oxide/Cu, respectively (Zhuang et al., 2014). The O 1s spectrum of Cu2(OH)3NO3@ZnO/AGF can be deconvoluted into three spin-orbit doublets, the fitting peaks at a binding energy of 533.3, 534.1, and 535.0 eV corresponding well with C=O, C-N-O, and C-O respectively. The Cu 2p core level XPS spectrum exhibits the Cu2+ 2p3/2 peak at 936.6 eV and the Cu2+ 2p1/2 peaks at 956.4 eV, with an energy gap of 19.8 eV, indicating that Cu exhibits only one oxidation valence of +2. The Zn 2p core level XPS spectrum exhibits the Zn 2p3/2 peak at 1,023 eV and the Zn 2p1/2 peaks at 1,047 eV, with an energy gap of 24 eV. All XPS results indicated that the successful synthesis of hierarchical dual nanozyme Cu2(OH)3NO3@ZnO on AGF.
The electrochemical properties of the stepwise fabrication process of nanohybrid microelectrodes, such as current responses (Ipa) and peak-to-peak separation (ΔEp), are studied by CV in 0.1 M KCl solution containing 5.0 mM [Fe(CN)6]3-/4-. The voltammetric curves for ZIF-8@ZnO/AGF and Cu2(OH)3NO3@ZnO/AGF exhibit a pair of well-defined quasi-reversible peaks (Figure 4A), which signify the oxidation/reduction process by outer-sphere electrode reaction of the redox couple. However, ZnO/AGF does not show a pair of well-defined quasi-reversible peaks, which is due to the insulator characteristics of ZnO. The current response is sequentially improved by modification with ZIF-8@ZnO and Cu2(OH)3NO3@ZnO nanohybrids, respectively. The oxidation and reduction peak currents of Cu2(OH)3NO3@ZnO/AGF are approximately 24 times higher than that of ZnO/AGF. However, the peak separation is increased after modification, which should be attributed to the non-porous structure of Cu2(OH)3NO3. Furthermore, CVs of the stepwise fabrication process of nanohybrid microelectrodes in contact with 0.1 M KCl solution containing 5.0 mM [Fe(CN)6]3–/4– at different scan rates from 0.01 to 0.09 V s−1 are recorded. Figures 4B,C and Supplementary Figure S6 show that the current response increases with the increase in scan rate. As seen in Figures 4B,C inset and Supplementary Figure S6 inset, plots of anodic (Ipa) peak currents against square root of scan rate show the great linearity, suggesting that the reaction mechanism is diffusion-controlled. Based on the Randles-Sevick equation, the electroactive surface area of the modified electrodes is calculated to be 0.0091, 0.0351, and 0.2995 cm2 for ZnO/AGF, ZIF-8@ZnO/AGF, and Cu2(OH)3NO3@ZnO/AGF, respectively. Compared to ZnO/AGF, the peak current densities of the modified electrodes are drastically increased in the order of ZnO/GF < ZIF-8@ZnO/GF < Cu2(OH)3NO3@ZnO/AGF, indicating that decorating Cu2(OH)3NO3@ZnO on the surface of AGF-based microelectrodes remarkably improves the electroactive surface area and increases the rate of electron transfer between active sites and the redox species in solution.
FIGURE 4. (A) CV curves of different microelectrodes in 0.1 M KCl solution containing 5.0 mM [Fe(CN)6]3−/4−. Scan rate: 0.09 V s−1. CV measurements of (B) ZIF-8@ZnO/AGF and (C) Cu2(OH)3NO3@ZnO/AGF are performed with [Fe(CN)6]3−/4− as the redox probe at different scan rates from 0.01 to 0.09 V s−1. (D) Nyquist plots of ZnO/AGF, ZIF-8@ZnO/AGF, and Cu2(OH)3NO3@ZnO/AGF in 5 mM [Fe(CN)6]3−/4− solution. Frequency range: 0.01–106 Hz.
To investigate the charge transport mechanisms of various fabricated microelectrodes, electrochemical impedance spectroscopy (EIS) measurements are conducted using [Fe(CN)6]3–/4– as redox probes, where the Nyquist plots can be fitted by an equivalent circuit (Supplementary Figure S7). Typically, the semicircular part of the Nyquist plot in the EIS coincides with the electron transfer-dependent process, where the diameter represents the interfacial charge transfer resistance (Rct), and the low frequency of the linear part is attributed to the diffusion-dependent process (Ciucci 2019). As shown in Figure 4D, there are larger Rct values of 504.4 Ω for ZnO/AGF. The Rct decreases to 141.4 Ω after ZIF-8 in situ grown on ZnO/AGF, which should be attributed to the porosity of ZIF-8 facilitating charge transport to GF by ions in solution. When the Cu2(OH)3NO3 is bonded to ZnO/AGF by chemical etching of ZIF-8, the Rct value is further decreased to 52.8 Ω, which is due to the positive charge of [Cu2(OH)3]+ (the electrostatic attraction between positively charged`` [Cu2(OH)3]+ and negatively charged [Fe(CN)6]3–/4– facilitates the charge transfer of [Fe(CN)6]3–/4– on the electrode surface). All the above EIS results indicate that the electrochemical sensing platforms for H2O2 are fabricated successfully as expected.
Considering the unique hierarchical structure of Cu2(OH)3NO3@ZnO/AGF, the electrocatalytic performance of different microelectrodes towards H2O2 has been investigated by CV measurements in 0.1 M PBS (pH 7.4) solution containing 5 mM H2O2. As shown in Figure 5A, the CV curve of Cu2(OH)3NO3@ZnO/AGF shows a clear reduction peak at −0.8 V, and the peak current density is much higher than that of ZnO/AGF and ZIF-8@ZnO/AGF. With the addition of H2O2, the reduction current increases linearly, indicating that Cu2(OH)3NO3@ZnO/AGF possesses high electrocatalytic activity towards H2O2 (Figure 5B). However, CV curves of ZIF-8@ZnO/AGF and ZnO/AGF show relatively low activity towards H2O2 (Supplementary Figure S8). This result can be explained in the following two aspects: (I) From a structure point of view, after in situ conversion of ZnO nanorods to ZIF-8, ZIF-8@ZnO/AGF possesses a larger surface area and adsorption capacity due to the unique porous structure of the metal-organic framework, benefiting the catalytic activity of ZIF-8@ZnO/AGF towards H2O2. In addition, a redox couple of Cu2+/Cu+ is introduced by the metal salt impregnation method to etch ZIF-8 into rime-like Cu2(OH)3NO3, which greatly improves the catalytic activity of Cu2(OH)3NO3@ZnO/AGF. (II) From an electrochemical perspective, the charge transfer from Cu2(OH)3NO3@ZnO to AGF microelectrode leads to a slight change in the electronic structure of Cu2(OH)3NO3, which further improves their electrocatalytic performance. In terms of the catalytic mechanisms, the exposure of Cu2+/Cu+ sites can act as a facilitating center to promote H2O2 activation. For the reaction process on Cu2(OH)3NO3, Cu2 (OH)3NO3 is partially reduced in situ into Cu2(OH)NO3 during electrochemical treatment (Yi et al., 2020). Then, the generated Cu2(OH)NO3 are oxidized again to Cu2(OH)3NO3 in the presence of H2O2. The mechanism process of Cu2(OH)3NO3@ZnO/AGF to catalyze the decomposition of H2O2 can be presented as follows (Ling et al., 2020):
FIGURE 5. (A) CV curves of ZnO/AGF, ZIF-8@ZnO/AGF, and Cu2(OH)3NO3@ZnO/AGF in 0.1 M PBS solution (pH 7.4) containing 5 mM H2O2. (B) CV curves of Cu2(OH)3NO3@ZnO/AGF in 0.1 M PBS solution (pH 7.4) containing different concentrations of H2O2. Scan rate: 50 mV s−1. (C) Amperometric current responses of Cu2(OH)3NO3@ZnO/AGF upon stepwise addition of different concentrations gradients of H2O2 in stirred 0.1 M PBS solution (pH 7.4). Inset: Amperometric current responses of Cu2(OH)3NO3@ZnO/AGF at low concentrations. Applied potential: −0.8 V. (D) Linear relationship between the corresponding current and H2O2 concentration.
Furthermore, the amperometric measurements are applied to evaluate the sensitivity of Cu2(OH)3NO3@ZnO/AGF upon stepwise addition of different concentrations of H2O2 in 0.1 M PBS solution with stirring (pH 7.4), where an optimal applied potential of −0.8 V is selected for testing. As shown in Figure 5C, a fast response signal can be observed with the addition from 1 μM to 2 mM H2O2, reaching 95% of the steady-state current within 3 s. The significant drop of the reduction current can be attributed to the rapid diffusion and activation of H2O2 on the active sites of the rime-like Cu2(OH)3NO3 with high specific surface, which could facilitate the fast electron transfer kinetics. Figure 5D shows that the proposed electrochemical sensor displays a wide linear range of the reduction current versus H2O2 concentration in the range of 1 μM–17.4 mM with a sensitivity value of 272 μA cm−2 mM−1. The calculated LOD is as low as 1 µM (S/N = 3). These results are better than or comparable with those of most H2O2 sensors reported in the recently published literature (Supplementary Table S1).
Anti-interference is another essential parameter of H2O2 sensors. In this work, the injection of 1.0 mM interfering species, i.e., K+, Na+, H2S, UA, AA, Glu, DA, GSH, DA, U, A, C, and G almost does not cause distinctive amperometric current density changes compared with 0.2 mM H2O2 under the negative applied potential of −0.8 V, demonstrating the preferential selectivity of Cu2(OH)3NO3@ZnO/AGF towards the detection of H2O2 (Figure 6A). The reproducibility of the proposed flexible electrode is also evaluated by successive monitoring of 0.2 mM H2O2 with six modified electrodes prepared under the same procedure. The relative standard derivation value of their amperometric current responses is measured to be only 5% (Figure 6B inset). In addition, the current response maintains 90% of its initial current value after 4 weeks by periodically recording the current response to 0.2 mM H2O2, indicative of its good long-term stability (Figure 6B).
FIGURE 6. (A) Amperometric current response curves of Cu2(OH)3NO3@ZnO/AGF to 0.2 mM H2O2, KCl, NaCl, H2S, UA, AA, Glu, DA, GSH, DA, U, A, C, and G. Applied potential: −0.8 V. (B) Current responses of six different Cu2(OH)3NO3@ZnO/AGF to 0.2 mM H2O2. Inset: Current responses of Cu2(OH)3NO3@ZnO/AGF to 0.2 mM H2O2 for 4 weeks.
Colon cancer is a clinically common human gastrointestinal malignancy with the third highest incidence worldwide and the fifth highest mortality rate among all malignancies in China, and the only way to improve survival rate as well as to escape from these deadly attacks is through early diagnosis (Wang et al., 2021). Therefore, the practical application of the proposed Cu2(OH)3NO3@ZnO/AGF microelectrode has been explored for tracking intracellular H2O2 fluctuations in different human colon cells, i.e., colon cancer cells (SW-48 and HCT-116), and epithelial cell lines from normal colon (NCM-460). Cytotoxicity tests show that Cu2(OH)3NO3@ZnO/AGF microelectrode does not induce cell death (apoptosis and necrosis) in SW-48 and NCM-460 cells (Figure 7A). fMLP is a kind of chemotactic peptide, used as a stimulator to initiate the release of H2O2 from living cells. The amperometric curves will exhibit an obvious reduced current density after injecting an amount of fMLP in the test cell containing different human body cells. Afterwards, the released H2O2 is quantified by electrochemical measurement using Cu2(OH)3NO3@ZnO/AGF as probe. Figure 7B shows the amperometric current responses to the addition of 10 μM fMLP in test solution containing 5.0 × 106 cells. The amperometric current values increase by 0.11, 0.62, and 0.85 μA for NCM-460, SW-48, and HCT-116, respectively. These results indicate that human colon cancer cells produce more extracellular H2O2 than normal cells, which is consistent with the titration measurement observation (Supplementary Table S2). Thus, the Cu2(OH)3NO3@ZnO/AGF-based electrochemical sensor can be applied to effectively differentiate normal and tumor cells for cancer cell detection.
FIGURE 7. (A) Live and dead body fluorescence images of SW-48 and NCM-460 cells, detected by Calcein-AM/PI, with Calcein-AM staining surviving cells green and PI staining dead cells red. Scale bar, 100 μm. (B) Amperometric current responses of Cu2(OH)3NO3@ZnO/AGF to the addition of 10 μM fMLP in test solution containing 5.0 × 106 NCM-460, SW-48, and HCT-116 cells.
In summary, we have developed a new type of high-performance flexible microelectrode based on MOF-mediated hierarchical nanohybrid-modified AGF, and used it in the electrochemical sensing system. Our results show that due to the synergistic effects of dual nanozymatic activity of rime-like hierarchical Cu2(OH)3NO3@ZnO as well as their unique structural and electrical properties, excellent sensitivity, selectivity, long-term stability, reproducibility, and practicality, the Cu2(OH)3NO3@ZnO/AGF microelectrode shows remarkable electrochemical sensing performance towards H2O2. The resultant electrochemical sensing platforms can be used for real-time tracking of H2O2 released from different kinds of live colon cells, which provides an effective strategy to distinguish cancer cells from the normal one for clinical diagnosis. An extension to more complex systems can be foreseen, upon conducting process/device engineering with this system. We envision that this work will open up a new pathway for the design of an assisting technology for cancer diagnosis and treatments in the future to hold great potential in the development of advanced implantable and wearable smartsensor for clinical practices.
The original contributions presented in the study are included in the article/Supplementary Material, further inquiries can be directed to the corresponding author.
WH: Conceptualization, Methodology, Investigation, and Writing—original draft. YX: Methodology, Validation, Investigation, Data curation, and Formal analysis. YS: Conceptualization, Writing—review and editing, Supervision, Project administration, and Funding acquisition.
This work is supported by National Natural Science Foundation of China (Project No. 51772110 and 82102513).
The authors declare that the research was conducted in the absence of any commercial or financial relationships that could be construed as a potential conflict of interest.
The reviewer SW declared a shared affiliation with the authors HW and YX to the handling editor at the time of the review.
All claims expressed in this article are solely those of the authors and do not necessarily represent those of their affiliated organizations, or those of the publisher, the editors and the reviewers. Any product that may be evaluated in this article, or claim that may be made by its manufacturer, is not guaranteed or endorsed by the publisher.
We thank all members of the laboratory for their technical support and academic discussions.
The Supplementary Material for this article can be found online at: https://www.frontiersin.org/articles/10.3389/fchem.2022.873187/full#supplementary-material
Asif, M., Aziz, A., Ashraf, G., Iftikhar, T., Sun, Y., Xiao, F., et al. (2022). Unveiling Microbiologically Influenced Corrosion Engineering to Transfigure Damages into Benefits: A Textile Sensor for H2O2 Detection in Clinical Cancer Tissues. Chem. Eng. J. 427, 131398. doi:10.1016/j.cej.2021.131398
Asif, M., Aziz, A., Ashraf, G., Wang, Z., Wang, J., Azeem, M., et al. (2018a). Facet-Inspired Core-Shell Gold Nanoislands on Metal Oxide Octadecahedral Heterostructures: High Sensing Performance toward Sulfide in Biotic Fluids. ACS Appl. Mater. Inter. 10, 36675–36685. doi:10.1021/acsami.8b12186
Asif, M., Aziz, A., Azeem, M., Wang, Z., Ashraf, G., Xiao, F., et al. (2018b). A Review on Electrochemical Biosensing Platform Based on Layered Double Hydroxides for Small Molecule Biomarkers Determination. Adv. Colloid Interf. Sci. 262, 21–38. doi:10.1016/j.cis.2018.11.001
Asif, M., Aziz, A., Wang, Z., Ashraf, G., Wang, J., Luo, H., et al. (2019). Hierarchical CNTs@CuMn Layered Double Hydroxide Nanohybrid with Enhanced Electrochemical Performance in H2S Detection from Live Cells. Anal. Chem. 91, 3912–3920. doi:10.1021/acs.analchem.8b04685
Aziz, A., Asif, M., Ashraf, G., Azeem, M., Majeed, I., Ajmal, M., et al. (2019a). Advancements in Electrochemical Sensing of Hydrogen Peroxide, Glucose and Dopamine by Using 2D Nanoarchitectures of Layered Double Hydroxides or Metal Dichalcogenides. A Review. Microchim Acta 186, 671. doi:10.1007/s00604-019-3776-z
Aziz, A., Asif, M., Ashraf, G., Farooq, U., Yang, Q., and Wang, S. (2021a). Trends in Biosensing Platforms for SARS-CoV-2 Detection: A Critical Appraisal against Standard Detection Tools. Curr. Opin. Colloid Interf. Sci. 52, 101418. doi:10.1016/j.cocis.2021.101418
Aziz, A., Asif, M., Ashraf, G., Iftikhar, T., Hu, J., Xiao, F., et al. (2021b). Boosting Electrocatalytic Activity of Carbon Fiber@fusiform-like Copper-Nickel LDHs: Sensing of Nitrate as Biomarker for NOB Detection Copper-Nickel LDHs: Sensing of Nitrate as Biomarker for NOB Detection. J. Hazard. Mater. 422, 126907. doi:10.1016/j.jhazmat.2021.126907
Aziz, A., Asif, M., Azeem, M., Ashraf, G., Wang, Z., Xiao, F., et al. (2019b). Self-stacking of Exfoliated Charged Nanosheets of LDHs and Graphene as Biosensor with Real-Time Tracking of Dopamine from Live Cells. Analytica Chim. Acta 1047, 197–207. doi:10.1016/j.aca.2018.10.008
Cai, W., Lai, J., Lai, T., Xie, H., and Ye, J. (2016). Controlled Functionalization of Flexible Graphene Fibers for the Simultaneous Determination of Ascorbic Acid, Dopamine and Uric Acid. Sensors Actuators B: Chem. 224, 225–232. doi:10.1016/j.snb.2015.09.079
Chen, L., Ding, X., Zeng, J., Jiao, L., Wu, C., Wang, Y., et al. (2019). A Three-Dimensional Hollow Graphene Fiber Microelectrode with Shrink-Effect-Enabled Enzyme Immobilization for Sensor Applications. Sci. Bull. 64, 718–722. doi:10.1016/j.scib.2019.04.031
Cheng, Y., Wang, R., Sun, J., and Gao, L. (2015). A Stretchable and Highly Sensitive Graphene-Based Fiber for Sensing Tensile Strain, Bending, and Torsion. Adv. Mater. 27, 7365–7371. doi:10.1002/adma.201503558
Ciucci, F. (2019). Modeling Electrochemical Impedance Spectroscopy. Curr. Opin. Electrochemistry 13, 132–139. doi:10.1016/j.coelec.2018.12.003
Cui, Y., Li, B., He, H., Zhou, W., Chen, B., and Qian, G. (2016). Metal-Organic Frameworks as Platforms for Functional Materials. Acc. Chem. Res. 49, 483–493. doi:10.1021/acs.accounts.5b00530
Ding, X., Bai, J., Xu, T., Li, C., Zhang, H.-M., and Qu, L. (2016). A Novel Nitrogen-Doped Graphene Fiber Microelectrode with Ultrahigh Sensitivity for the Detection of Dopamine. Electrochemistry Commun. 72, 122–125. doi:10.1016/j.elecom.2016.09.021
Guo, X., Lin, C., Zhang, M., Duan, X., Dong, X., Sun, D., et al. (2021). 2D/3D Copper-Based Metal-Organic Frameworks for Electrochemical Detection of Hydrogen Peroxide. Front. Chem. 9, 743637. doi:10.3389/fchem.2021.743637
Huang, L., Niu, Y., Li, R., Liu, H., Wang, Y., Xu, G., et al. (2019). VOx Quantum Dots with Multienzyme-Mimic Activities and the Application in Constructing a Three-Dimensional (3D) Coordinate System for Accurate Discrimination of the Hydrogen Peroxide over a Broad Concentration Range. Anal. Chem. 91, 5753–5761. doi:10.1021/acs.analchem.8b05923
Huang, L., Santiago, D., Loyselle, P., and Dai, L. (2018). Graphene‐Based Nanomaterials for Flexible and Wearable Supercapacitors. Small 14, 1800879. doi:10.1002/smll.201800879
Ji, S., Jiang, B., Hao, H., Chen, Y., Dong, J., Mao, Y., et al. (2021). Matching the Kinetics of Natural Enzymes with a Single-Atom Iron Nanozyme. Nat. Catal. 4, 407–417. doi:10.1038/s41929-021-00609-x
Labib, M., Sargent, E. H., and Kelley, S. O. (2016). Electrochemical Methods for the Analysis of Clinically Relevant Biomolecules. Chem. Rev. 116, 9001–9090. doi:10.1021/acs.chemrev.6b00220
Li, P., Sun, X.-Y., and Shen, J.-S. (2021). A Multi-Catalytic Sensing for Hydrogen Peroxide, Glucose, and Organophosphorus Pesticides Based on Carbon Dots. Front. Chem. 9, 713104. doi:10.3389/fchem.2021.713104
Lin, M., Song, P., Zhou, G., Zuo, X., Aldalbahi, A., Lou, X., et al. (2016). Electrochemical Detection of Nucleic Acids, Proteins, Small Molecules and Cells Using a DNA-Nanostructure-Based Universal Biosensing Platform. Nat. Protoc. 11, 1244–1263. doi:10.1038/nprot.2016.071
Ling, P., Cheng, S., Chen, N., Qian, C., and Gao, F. (2020). Nanozyme-Modified Metal-Organic Frameworks with Multienzymes Activity as Biomimetic Catalysts and Electrocatalytic Interfaces. ACS Appl. Mater. Inter. 12, 17185–17192. doi:10.1021/acsami.9b23147
Lippert, A. R., Van de Bittner, G. C., and Chang, C. J. (2011). Boronate Oxidation as a Bioorthogonal Reaction Approach for Studying the Chemistry of Hydrogen Peroxide in Living Systems. Acc. Chem. Res. 44, 793–804. doi:10.1021/ar200126t
Munyemana, J. C., Chen, J., Wei, X., Ali, M. C., Han, Y., and Qiu, H. (2020). Deep Eutectic Solvent-Assisted Facile Synthesis of Copper Hydroxide Nitrate Nanosheets as Recyclable Enzyme-Mimicking Colorimetric Sensor of Biothiols. Anal. Bioanal. Chem. 412, 4629–4638. doi:10.1007/s00216-020-02712-7
Peng, Y., Lin, D., Justin Gooding, J., Xue, Y., and Dai, L. (2018). Flexible Fiber-Shaped Non-enzymatic Sensors with a Graphene-Metal Heterostructure Based on Graphene Fibres Decorated with Gold Nanosheets. Carbon 136, 329–336. doi:10.1016/j.carbon.2018.05.004
Sajjad, A., Bhatti, S. H., Ali, Z., Jaffari, G. H., Khan, N. A., Rizvi, Z. F., et al. (2021). Photoinduced Fabrication of Zinc Oxide Nanoparticles: Transformation of Morphological and Biological Response on Light Irradiance. ACS Omega 6, 11783–11793. doi:10.1021/acsomega.1c01512
Shen, X., Liu, W., Gao, X., Lu, Z., Wu, X., and Gao, X. (2015). Mechanisms of Oxidase and Superoxide Dismutation-like Activities of Gold, Silver, Platinum, and Palladium, and Their Alloys: A General Way to the Activation of Molecular Oxygen. J. Am. Chem. Soc. 137, 15882–15891. doi:10.1021/jacs.5b10346
Sun, Y., He, K., Zhang, Z., Zhou, A., and Duan, H. (2015). Real-Time Electrochemical Detection of Hydrogen Peroxide Secretion in Live Cells by Pt Nanoparticles Decorated Graphene-Carbon Nanotube Hybrid Paper Electrode. Biosens. Bioelectron. 68, 358–364. doi:10.1016/j.bios.2015.01.017
Sun, Y., Zheng, H., Wang, C., Yang, M., Zhou, A., and Duan, H. (2016). Ultrasonic-Electrodeposition of PtPd Alloy Nanoparticles on Ionic Liquid-Functionalized Graphene Paper: Towards a Flexible and Versatile Nanohybrid Electrode. Nanoscale 8, 1523–1534. doi:10.1039/C5NR06912B
Wang, H., Wan, K., and Shi, X. (2018). Recent Advances in Nanozyme Research. Adv. Mater. 31, 1805368. doi:10.1002/adma.201805368
Wang, J., Liu, L., Cai, Y., Gao, Y., Guo, Z., Yu, F., et al. (2021). Trends in the Age-Related Incidence of colon and Rectal Cancers in China, 2005-2015. Dig. Liver Dis. 53, 908–914. doi:10.1016/j.dld.2021.01.009
Wang, L., Wang, L., Zhang, Y., Pan, J., Li, S., Sun, X., et al. (2018). Weaving Sensing Fibers into Electrochemical Fabric for Real-Time Health Monitoring. Adv. Funct. Mater. 28, 1804456. doi:10.1002/adfm.201804456
Wu, J., Wang, X., Wang, Q., Lou, Z., Li, S., Zhu, Y., et al. (2019). Nanomaterials with Enzyme-like Characteristics (Nanozymes): Next-Generation Artificial Enzymes (II). Chem. Soc. Rev. 48, 1004–1076. doi:10.1039/C8CS00457A
Xiao, F., Wang, L., and Duan, H. (2016). Nanomaterial Based Electrochemical Sensors for In Vitro Detection of Small Molecule Metabolites. Biotechnol. Adv. 34, 234–249. doi:10.1016/j.biotechadv.2016.01.006
Xin, G., Yao, T., Sun, H., Scott, S. M., Shao, D., Wang, G., et al. (2015). Highly Thermally Conductive and Mechanically Strong Graphene Fibers. Science 349, 1083–1087. doi:10.1126/science.aaa6502
Xin, G., Zhu, W., Deng, Y., Cheng, J., Zhang, L. T., Chung, A. J., et al. (2019). Microfluidics-Enabled Orientation and Microstructure Control of Macroscopic Graphene Fibres. Nat. Nanotech 14, 168–175. doi:10.1038/s41565-018-0330-9
Xu, Q., Yuan, H., Dong, X., Zhang, Y., Asif, M., Dong, Z., et al. (2018). Dual Nanoenzyme Modified Microelectrode Based on Carbon Fiber Coated with AuPd Alloy Nanoparticles Decorated Graphene Quantum Dots Assembly for Electrochemical Detection in Clinic Cancer Samples. Biosens. Bioelectron. 107, 153–162. doi:10.1016/j.bios.2018.02.026
Xu, Z., Peng, L., Liu, Y., Liu, Z., Sun, H., Gao, W., et al. (2016). Experimental Guidance to Graphene Macroscopic Wet-Spun Fibers, Continuous Papers, and Ultralightweight Aerogels. Chem. Mater. 29, 319–330. doi:10.1021/acs.chemmater.6b02882
Yi, J. D., Xie, R., Xie, Z. L., Chai, G. L., Liu, T. F., Chen, R. P., et al. (2020). Highly Selective CO 2 Electroreduction to CH 4 by In Situ Generated Cu 2 O Single‐Type Sites on a Conductive MOF: Stabilizing Key Intermediates with Hydrogen Bonding. Angew. Chem. Int. Ed. 59, 23641–23648. doi:10.1002/anie.202010601
Zeng, J., Xu, R., Jiao, L., Wang, Y., Chen, L., Windle, C. D., et al. (2019). A 3D-Graphene Fiber Electrode Embedded with Nitrogen-Rich-Carbon-Coated ZIF-67 for the Ultrasensitive Detection of Adrenaline. J. Mater. Chem. B 7, 5291–5295. doi:10.1039/C9TB01223K
Zhang, T., Xing, Y., Song, Y., Gu, Y., Yan, X., Lu, N., et al. (2019). AuPt/MOF-Graphene: A Synergistic Catalyst with Surprisingly High Peroxidase-like Activity and its Application for H2O2 Detection. Anal. Chem. 91, 10589–10595. doi:10.1021/acs.analchem.9b01715
Zhang, Y., Li, Y., Ming, P., Zhang, Q., Liu, T., Jiang, L., et al. (2016). Ultrastrong Bioinspired Graphene-Based Fibers via Synergistic Toughening. Adv. Mater. 28, 2834–2839. doi:10.1002/adma.201506074
Zhao, A., She, J., Manoj, D., Wang, T., Sun, Y., Zhang, Y., et al. (2020). Functionalized Graphene Fiber Modified by Dual Nanoenzyme: Towards High-Performance Flexible Nanohybrid Microelectrode for Electrochemical Sensing in Live Cancer Cells. Sensors Actuators B: Chem. 310, 127861. doi:10.1016/j.snb.2020.127861
Zhao, A., She, J., Xiao, C., Xi, J., Xu, Y., Manoj, D., et al. (2021). Green and Controllable Synthesis of Multi-Heteroatoms Co-doped Graphene Fiber as Flexible and Biocompatible Microelectrode for In Situ Electrochemical Detection of Biological Samples. Sensors Actuators B: Chem. 335, 129683. doi:10.1016/j.snb.2021.129683
Keywords: graphene fibers, hierarchical structure, flexible microelectrode, nanozymatic electrochemical sensor, in situ cancer cell detection
Citation: Huang W, Xu Y and Sun Y (2022) Functionalized Graphene Fiber Modified With MOF-Derived Rime-Like Hierarchical Nanozyme for Electrochemical Biosensing of H2O2 in Cancer Cells. Front. Chem. 10:873187. doi: 10.3389/fchem.2022.873187
Received: 10 February 2022; Accepted: 17 February 2022;
Published: 22 March 2022.
Edited by:
Muhammad Asif, Wuhan Institute of Technology, ChinaReviewed by:
Shenqi Wang, Huazhong University of Science and Technology, ChinaCopyright © 2022 Huang, Xu and Sun. This is an open-access article distributed under the terms of the Creative Commons Attribution License (CC BY). The use, distribution or reproduction in other forums is permitted, provided the original author(s) and the copyright owner(s) are credited and that the original publication in this journal is cited, in accordance with accepted academic practice. No use, distribution or reproduction is permitted which does not comply with these terms.
*Correspondence: Yimin Sun, eW1zdW5Ad2l0LmVkdS5jbg==
Disclaimer: All claims expressed in this article are solely those of the authors and do not necessarily represent those of their affiliated organizations, or those of the publisher, the editors and the reviewers. Any product that may be evaluated in this article or claim that may be made by its manufacturer is not guaranteed or endorsed by the publisher.
Research integrity at Frontiers
Learn more about the work of our research integrity team to safeguard the quality of each article we publish.