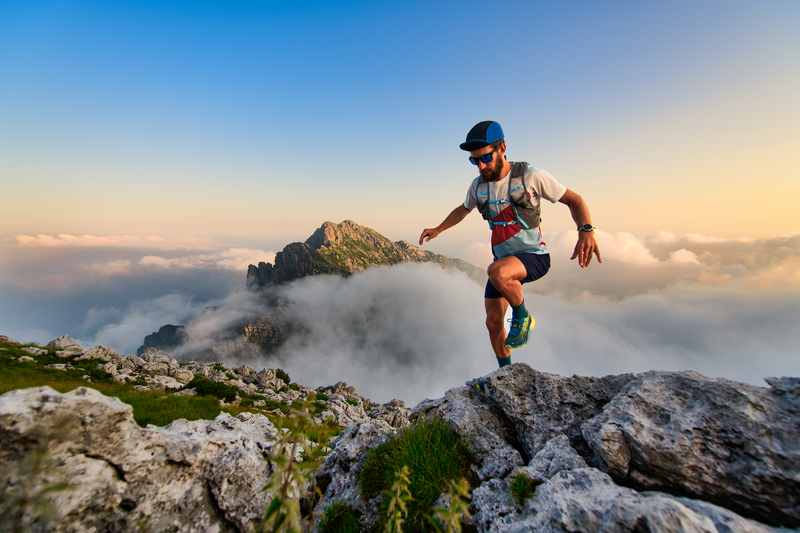
95% of researchers rate our articles as excellent or good
Learn more about the work of our research integrity team to safeguard the quality of each article we publish.
Find out more
ORIGINAL RESEARCH article
Front. Chem. , 15 December 2022
Sec. Medicinal and Pharmaceutical Chemistry
Volume 10 - 2022 | https://doi.org/10.3389/fchem.2022.1091566
This article is part of the Research Topic Advances in Natural Product Chemistry: Yunnan University 100th Anniversary View all 11 articles
Aryl acrylonitriles are an important subclass of acrylonitriles in the medicinal chemistry and pharmaceutical industry. Herein, an efficient synthesis of aryl acrylonitrile derivatives using a Palladium/NIXANTPHOS-based catalyst system was developed. This approach furnishes a variety of substituted and functionalized aryl acrylonitriles (up to 95% yield). The scalability of the transformation and the synthetic versatility of aryl acrylonitrile were demonstrated.
Acrylonitriles, especially substituted acrylonitriles, are versatile building blocks widely occurring in the pharmaceutical industry, natural products and synthetic organic chemistry (Fringuelli et al., 1994; Fleming, 1999; Fleming et al., 2010; Carta et al., 2011; Shen et al., 2015; Baker et al., 2020; Sirim et al., 2020; Solangi et al., 2020; Baker et al., 2021). Among acrylonitrile-containing molecules, aryl acrylonitriles are an important subclass in the medicinal chemistry and pharmaceutical industry (ANI-7 (Tarleton et al., 2011), CDCPA (Baker et al., 2018), TPAT-AN-XF (Niu et al., 2019), CC-5079 (Zhang et al., 2006), Entacapone (Seeberger and Hauser, 2009), and Rilpivirine (Clercq, 2005) Figure 1). Therefore, the development of efficient and practical approaches for the synthesis of aryl acrylonitriles remains in demand.
Classical synthetic routes to acrylonitrile derivatives include the Wittig/Horner−Wadsworth−Emmons reaction (Zhang et al., 1998; Kojima et al., 2002; Fang et al., 2011; Ando et al., 2013) and Peterson type reactions (Kojima et al., 2004; Pabmo’ et al., 1990; Palomo et al., 1990). However, these procedures suffer from limitations such as a poor substrate scope, low efficiency for the synthesis of polysubstituted acrylonitriles. During the past decade, organic chemists keep searching new and efficient reactions, including oxidative Heck-type reactions (Zou et al., 2003; Zhang and Liebeskind, 2006), cyanation of alkenyl halides (Stuhl, 1985; Alterman and Hallberg, 2000; Pradal and Evano, 2014; Ahuja and Sudalai, 2015; Chaitanya and Anbarasan, 2015; Yang et al., 2018), alcohols (Oishi et al., 2009; Rokade et al., 2012; Thiyagarajan and Gunanathan, 2018; Yadav et al., 2020), aldehydes (Tomioka et al., 2011; Laulhe et al., 2012; Del Fiandra et al., 2016; Wu et al., 2016), acrylamide/oxime dehydration (Yamaguchi et al., 2007; Zhou et al., 2009), carbocyanation of alkynes (Nakao et al., 2007; Cheng et al., 2008; Minami et al., 2013; Yang et al., 2013; He et al., 2016; Qi et al., 2017), cross-metathesis (Crowe and Goldberg, 1995; Randl et al., 2001; Mu et al., 2019), and direct conversion of allylic carbon to nitrile (Qin and Jiao, 2010; Zhou et al., 2010) have been developed and could be applied for the synthesis of acrylonitriles. For example, Jiao developed a series of powerful synthesis of substituted acrylonitriles, which used allyl esters or halides and NaN3 or TMSN3 by a tandem Pd-catalyzed azidation and the subsequent oxidative rearrangement process (Scheme 1A) (Qin and Jiao, 2010; Zhou et al., 2010; Jiao et al., 2011; Wang and Jiao, 2014). Engle reported a direct oxidative cyanation of terminal and internal alkenes to prepare substituted acrylonitriles using a homogeneous copper catalyst and a bystanding N–F oxidant (Scheme 1B) (Gao et al., 2018). Recently, Liu reported an elegant synthesis of aryl substituted terminal acrylonitriles through Ni/Mn-catalyzed hydrocyanation of terminal alkynes with Zn(CN)2 (Scheme 1C) (Zhang et al., 2018). Milstein reported an effective synthesis of aryl acrylonitriles through dehydrogenative coupling of alcohols with nitriles catalyzed by a pincer complex of manganese at 135°C for 43–60 h (Scheme 1D) (Chakraborty et al., 2017).
Despite these advances, these motheds are generally restricted by the addition of dangerous reagents (cyanide reagents, azide reagents) and stoichiometric amount of oxidants (DDQ, Selectfluor), high catalyst loading, tedious synthetic procedures, low yielding and high reaction temperatures. Therefore, an optional method for the efficient synthesis of aryl acrylonitrile derivatives under mild reaction conditions using simple, easily available substrates are very necessary. Herein, we report an efficient synthesis of aryl acrylonitrile derivatives using a Palladium/NIXANTPHOS-based catalyst system. This approach furnishes efficient access to a variety of substituted and functionalized aryl acrylonitriles (21 examples, up to 95%). The scalability of the transformation was demonstrated and the derivatizations of the aryl acrylonitrile were conducted.
We initiated our reaction optimization by using phenylacetonitrile 1a and 2-bromoprop-1-ene 2a as the model substrates. At the outset, based on our experience with deprotonative cross-coupling processes of weakly acidic substrates (Yang et al., 2016; Duan et al., 2018; Liu et al., 2018), we have found that NIXANTPHOS can effectively implement these conversions. The high reactivity of the Pd/NIXANTPHOS-based system may be due to the presence of the main group metal and the deprotonation of the ligand N−H moiety under basic reaction conditions (Zhang et al., 2014). A variety of palladium source including different Pd0 and PdII precursors, phosphine ligands and six bases (LiN(SiMe3)2, NaN(SiMe3)2, KN(SiMe3)2, LiOtBu, NaOtBu and KOtBu) were examined the coupling of phenylacetonitrile 1a and 2-bromoprop-1-ene 2a in DME at 65°C for 1 h (Table 1, entries 1–10) (see the optimization of reaction conditions on page S2 in Supplementary Material). The top Pd/L/base combination from this screen was Pd(OAc)2/NIXANTPHOS/NaOtBu resulted in 20% assay yield (AY, determined by 1H NMR analysis). Other four solvents (dioxane, CPME, THF and Toluene) were tested, which only afforded trace amount of product (0%–13%) (entries 11–14). Raising the reaction temperature to 80 and 100°C led to increases to 57 and 28% AY, respectively (entries 15 and 16). Changing the equivalent of 2a from 2 to 4 led to increases of AY (entries 17–19). When a 3 equivalent was employed, the AY increased to 77% (75% isolated yield, entry 18). Reducing the Pd/ligand ratio to 5:10, AY dropped to 57% (entry 20).
L1: NIXANTPHOS, L2: XANTPHOS, L3: PPh3, L4: P (o-TOL)3, L5: P (1-NAP)3, L6: rac-BINAP, L7: JOHNPHOS, L8: PCy3
B1: LiN(SiMe3)2, B2: NaN(SiMe3)2, B3: KN(SiMe3)2, B4: LiOtBu, B5: NaOtBu, B6: KOtBu
With the optimized reaction conditions (Table 1, entry 18), we explored the structural diversity of vinyl halides/triflates using phenylacetonitrile 1a as the model substrate. As shown in Table 2, 2-bromo-1-ene 2a delivered aryl acrylonitrile 3aa in 75% yield, while 2-chloro-1-ene 2a’ gave 55% yield. Vinyl chloride 1-chloro-2-methylprop-1-ene 2b led to product 3ab in 54% yield. (E)-(1-bromoprop-1-en-2-yl)benzene 2c provided product 3ac in 81% yield (67% yield for 5% Pd/10% L). Sterically hindered bromomethylenecyclohexane 2d rendered product 3ad with excellent yield of 84% yield (70% yield for 5% Pd/10% L). Trans- and cis-2-bromobut-2-ene (2e and 2f) furnished products 3ae and 3af in overall 57% and 51% yields. 2-Bromo-3-methylbut-2-ene 2g afforded product 3ag in overall 65% yield. Cycloolefin halides/triflates were all suitable reaction partners in this transformation and provided a series of cycloalkane-functionalized aryl acrylonitriles in moderate yields. 1-Chlorocyclopent-1-ene 2h led to product 3ah in 50% yield. Six/seven/eight-membered cycloolefin triflates proceeded the corresponding products 3ai, 3aj, and 3ak in 65%, 61% and 55% yields, respectively.
We next explored the scope of arylacetonitriles using sterically hindered bromomethylenecyclohexane 2d as the model substrate. As shown in Table 3, in general, arylacetonitriles bearing electron-donating and electron-withdrawing Ar groups or heterocyclic rendered good to excellent yields under the standard conditions (Table 3). Arylacetonitriles possessing alkyl 4-Me (1b) and 2-Me (1c) reacted with bromomethylenecyclohexane 2d to give aryl acrylonitriles 3bd and 3cd in 84% and 81% yields (69% and 63% yields for 5% Pd). Arylacetonitrile with electro-donating (4-OMe, 1d) substituents provided product 3dd in 67% yield. Arylacetonitriles bearing electron-withdrawing 4-F (1e), 4-Cl (1f) and 4-Br (1g) generated the products 3ed, 3fd and 3gd in 83% (78% yield for 5% Pd), 95% (79% yield for 5% Pd) and 50% yields, respectively. The sterically demanding 2-naphthyl acetonitrile (1h) was well tolerated, led to product 3hd in 67% yield. Interesting, medicinally important heterocyclic-containing acetonitriles were suitable reaction partners. 2-(1-Methyl-1H-indol-3-yl)acetonitrile (1i) reacted with 2d to generate the aryl acrylonitrile 3id with excellent yield of 93% (79% yield for 5% Pd). 2-(Thiophen-2-yl)acetonitrile (1j) provided product 3jd in 47% yield.
To evaluate the scalability of our transformation, we next carried out the reaction of phenylacetonitrile 1a and 2-bromo-1-ene 2a on a gram-scale under the optimal conditions (Scheme 2). The desired aryl acrylonitrile 3aa was isolated in 1.03 g (70% yield), demonstrating the scalability of our method.
Finally, to illustrate further the synthetic versatility of the resulting aryl acrylonitrile, a series of derivatizations were performed on 3aa (Scheme 3). Thus, the selective reduction of the carbon-carbon double bond of aryl acrylonitrile 3aa using Pd/C and hydrogen led to the substituted saturated phenylacetonitrile 4aa in 95% yield. Then, the selective reduction of the nitrile group of 3aa employing DIBAL-H in toluene at 0°C generated the corresponding α,β-unsaturated aldehyde 4ab in 39% yield (Chen et al., 2019). Meanwhile, the hydrolysis of the nitrile group of 3aa using 30% H2O2 and NaOH in MeOH rendered the corresponding α,β-unsaturated amide 4ac in 78% yield. Furthermore, the epoxidation of the carbon-carbon double bond and hydrolysis of the nitrile group of 3aa using 30% H2O2 and K2CO3 in DMSO afforded the corresponding α,β-epoxy amide 4ad in 57% yield.
A possible catalytic cycle is shown in Scheme 4 based on Walsh’s work on the palladium-catalyzed deprotonative cross-coupling processes (Hussain et al., 2014; Jia et al., 2014; Mao et al., 2014; Jia et al., 2015). The deprotonation of aryl acetonitrile by NaOtBu gives benzyl anions. After oxidative addition of the vinyl bromide to Pd (0), the vinyl palladium intermediate is proposed to bind the benzyl anions to form the palladium complex. Then, reductive elimination occurs to afford the enenitrile and regenerates Pd (0). Finally, enenitrile isomerizes to obtain aryl acrylonitrile.
In conclusion, we have successfully synthesized a series of aryl acrylonitrile derivatives employing a Pd/NIXANTPHOS-based catalyst system for the first time. In this protocol, commercially available arylacetonitriles and vinyl bromides/chlorides/triflates underwent palladium-catalyzed α-alkenylation to furnish efficient access to a variety of substituted and functionalized aryl acrylonitriles. The scalability of the mothed was demonstrated by the gram-scale reaction. A series of derivatization of aryl acrylonitrile were performed, including the selective reduction of the double bond or nitrile group, the hydrolysis of the nitrile group, and the epoxidation of the double bond, which demonstrated the synthetic versatility of aryl acrylonitrile. It is noteworthy that this approach does not require dangerous reagents and stoichiometric amount of oxidants, which enables the synthesis of a range of aryl acrylonitriles in an effective and straightforward means.
The original contributions presented in the study are included in the article/Supplementary Material, further inquiries can be directed to the corresponding authors.
YJ and BW contributed equally to this work. XY conceived of the project. GD and XY supervised the project. DL, DX, ZL, and LL performed the research. GD and XY wrote the manuscript.
This work was supported by grants from the National Key R&D Program of China (2019YFE0109200), NSFC (21662043), NSF of Yunnan (202207AA110007, 202207AB110002), Yunnan Science and Technology Department and Yunnan University Joint Fund Project (2019FY003010), Ling-Jun Scholars Yunnan Province (202005AB160003), Program for Xingdian Talents (Yun-Ling Scholars) and IRTSTYN.
The authors declare that the research was conducted in the absence of any commercial or financial relationships that could be construed as a potential conflict of interest.
The handling editor JD declared a past co-authorship with the author XY, YJ.
All claims expressed in this article are solely those of the authors and do not necessarily represent those of their affiliated organizations, or those of the publisher, the editors and the reviewers. Any product that may be evaluated in this article, or claim that may be made by its manufacturer, is not guaranteed or endorsed by the publisher.
The Supplementary Material for this article can be found online at: https://www.frontiersin.org/articles/10.3389/fchem.2022.1091566/full#supplementary-material
Ahuja, B. B., and Sudalai, A. (2015). Cu-Catalyzed debrominative cyanation of gem-dibromoolefins: A facile access to α, β-unsaturated nitriles. Org. Biomol. Chem. 13, 5918–5923. doi:10.1039/c5ob00394f
Alterman, M., and Hallberg, A. (2000). Fast microwave-assisted preparation of aryl and vinyl nitriles and the corresponding tetrazoles from organo-halides. J. Org. Chem. 65, 7984–7989. doi:10.1021/jo0009954
Ando, K., Okumura, M., and Nagaya, S. (2013). Highly Z-selective synthesis of α, β-unsaturated nitriles using the Horner–Wadsworth–Emmons reaction. Tetrahedron Lett. 54, 2026–2028. doi:10.1016/j.tetlet.2013.02.020
Baker, J. R., Gilbert, J., Paula, S., Zhu, X., Sakoff, J. A., and McCluskey, A. (2018). Dichlorophenylacrylonitriles as AhR ligands that display selective breast cancer cytotoxicity in vitro. ChemMedChem 13, 1447–1458. doi:10.1002/cmdc.201800256
Baker, J. R., Russell, C. C., Gilbert, J., McCluskey, A., and Sakoff, J. A. (2021). Amino alcohol acrylonitriles as broad spectrum and tumour selective cytotoxic agents. RSC Med. Chem. 12, 929–942. doi:10.1039/d1md00021g
Baker, J. R., Russell, C. C., Gilbert, J., Sakoff, J. A., and McCluskey, A. (2020). Amino alcohol acrylonitriles as activators of the aryl hydrocarbon receptor pathway: An unexpected MTT phenotypic screening outcome. ChemMedChem 15, 490–505. doi:10.1002/cmdc.201900643
Carta, A., Briguglio, I., Piras, S., Boatto, G., La Colla, P., Loddo, R., et al. (2011). 3-Aryl-2-[1H-benzotriazol-1-yl]acrylonitriles: A novel class of potent tubulin inhibitors. Eur. J. Med. Chem. 46, 4151–4167. doi:10.1016/j.ejmech.2011.06.018
Chaitanya, M., and Anbarasan, P. (2015). Rhodium-catalyzed cyanation of C(sp2)-H bond of alkenes. Org. Lett. 17, 3766–3769. doi:10.1021/acs.orglett.5b01746
Chakraborty, S., Das, U. K., Ben-David, Y., and Milstein, D. (2017). Manganese catalyzed α-olefination of nitriles by primary alcohols. J. Am. Chem. Soc. 139, 11710–11713. doi:10.1021/jacs.7b06993
Chen, L. L., Zhang, J. W., Chen, P., Zhang, S., Yang, W. W., Fu, J. Y., et al. (2019). Base-controlled divergent synthesis of 5-cyanobenzoxepines and benzofuro[2, 3-b]pyridines from 2-bromophenylacetonitriles and ynones. Org. Lett. 21, 5457–5461. doi:10.1021/acs.orglett.9b01700
Cheng, Y., Duan, Z., Yu, L., Li, Z., Zhu, Y., and Wu, Y. (2008). Palladium-catalyzed three-component arylcyanation of internal alkynes with aryl bromides and K4[Fe(CN)6]. Org. Lett. 10, 901–904. doi:10.1021/ol703018t
Clercq, E. D. (2005). Emerging anti-HIV drugs. Expert Opin. Emerg. Drugs 10, 241–274. doi:10.1517/14728214.10.2.241
Crowe, W. E., and Goldberg, D. R. (1995). Acrylonitrile cross-metathesis: Coaxing olefin metathesis reactivity from a reluctant substrate. J. Am. Chem. Soc. 117, 5162–5163. doi:10.1021/ja00123a023
Del Fiandra, C., Moccia, M., Cerulli, V., and Adamo, M. F. (2016). Catalytic asymmetric conjugate addition of isocyanoacetate to (Z)-3-substituted-2-(4-pyridyl)-acrylonitrile, a reactive class of Michael acceptor. Chem. Commun. 52, 1697–1700. doi:10.1039/c5cc08105j
Duan, S., Li, M., Ma, X., Chen, W., Li, L., Zhang, H., et al. (2018). Palladium-catalyzed alkenylation of azaarylmethylamines with vinyl halides. Adv. Synth. Catal. 360, 4837–4842. doi:10.1002/adsc.201801045
Fang, F., Li, Y., and Tian, S. K. (2011). Stereoselective olefination of N-sulfonyl imines with stabilized phosphonium ylides for the synthesis of electron-deficient alkenes. Eur. J. Org. Chem. 2011, 1084–1091. doi:10.1002/ejoc.201001379
Fleming, F. F. (1999). Nitrile-containing natural products. Nat. Prod. Rep. 16, 597–606. doi:10.1039/A804370A
Fleming, F. F., Yao, L., Ravikumar, P. C., Funk, L., and Shook, B. C. (2010). Nitrile-containing pharmaceuticals: Efficacious roles of the nitrile pharmacophore. J. Med. Chem. 53, 7902–7917. doi:10.1021/jm100762r
Fringuelli, F., Pani, G., Piermatti, O., and Pizzo, F. (1994). Condensation reactions in water of active methylene compounds with arylaldehydes. One-pot synthesis of flavonols. Tetrahedron 50, 11499–11508. doi:10.1016/S0040-4020(01)89287-5
Gao, D. W., Vinogradova, E. V., Nimmagadda, S. K., Medina, J. M., Xiao, Y., Suciu, R. M., et al. (2018). Direct access to versatile electrophiles via catalytic oxidative cyanation of alkenes. J. Am. Chem. Soc. 140, 8069–8073. doi:10.1021/jacs.8b03704
He, Y. T., Li, L. H., Wang, Q., Wu, W., and Liang, Y. M. (2016). Synthesis of β-difluoroalkylated acrylonitriles in the presence of copper powder. Org. Lett. 18, 5158–5161. doi:10.1021/acs.orglett.6b02627
Hussain, N., Frensch, G., Zhang, J., and Walsh, P. J. (2014). Chemo- and regioselective C(sp3)-H arylation of unactivated allylarenes by deprotonative cross-coupling. Angew. Chem. Int. Ed. 53, 3693–3697. doi:10.1002/anie.201309084
Jia, T., Bellomo, A., Montel, S., Zhang, M., El Baina, K., Zheng, B., et al. (2014). Diaryl sulfoxides from aryl benzyl sulfoxides: A single palladium-catalyzed triple relay process. Angew. Chem. Int. Ed. 53, 260–264. doi:10.1002/anie.201307172
Jia, T., Zhang, M., Sagamanova, I. K., Wang, C. Y., and Walsh, P. J. (2015). Palladium catalyzed diaryl sulfoxide generation from aryl benzyl sulfoxides and aryl chlorides. Org. Lett. 17, 1168–1171. doi:10.1021/acs.orglett.5b00092
Jiao, N., Zhou, W., Xu, J., and Zhang, L. (2011). An efficient approach to alkenyl nitriles from allyl esters. Synlett 2011, 887–890. doi:10.1055/s-0030-1259719
Kojima, S., Fukuzaki, T., Yamakawa, A., and Murai, Y. (2004). Highly (Z)-selective synthesis of β-monosubstituted-α, β-unsaturated cyanides using the Peterson reaction. Org. Lett. 6, 3917–3920. doi:10.1021/ol0486728
Kojima, S., Kawaguchi, K., Matsukawa, S., Uchida, K., and Akiba, K.-y. (2002). Highly Z-selective synthesis of disubstituted α, β-Unsaturated cyanides and amides using 10-P-5 Wittig type reagents. Chem. Lett. 31, 170–171. doi:10.1246/cl.2002.170
Laulhe, S., Gori, S. S., and Nantz, M. H. (2012). A chemoselective, one-pot transformation of aldehydes to nitriles. J. Org. Chem. 77, 9334–9337. doi:10.1021/jo301133y
Liu, Z., Li, M., Wang, B., Deng, G., Chen, W., Kim, B.-S., et al. (2018). Chemoselective synthesis of aryl(pyridinyl)methanol derivatives through Ni-NIXANTPHOS catalyzed α-arylation and tandem arylation/rearrangement of pyridylmethyl ethers. Org. Chem. Front. 5, 1870–1876. doi:10.1039/c8qo00207j
Mao, J., Jia, T., Frensch, G., and Walsh, P. J. (2014). Palladium-catalyzed debenzylative cross-coupling of aryl benzyl sulfides with aryl bromides: Synthesis of diaryl sulfides. Org. Lett. 16, 5304–5307. doi:10.1021/ol502470e
Minami, Y., Yoshiyasu, H., Nakao, Y., and Hiyama, T. (2013). Highly chemoselective carbon-carbon sigma-bond activation: Nickel/Lewis acid catalyzed polyfluoroarylcyanation of alkynes. Angew. Chem. Int. Ed. 52, 883–887. doi:10.1002/anie.201207880
Mu, Y., Nguyen, T. T., Koh, M. J., Schrock, R. R., and Hoveyda, A. H. (2019). E- and Z-di- and tri-substituted alkenyl nitriles through catalytic cross-metathesis. Nat. Chem. 11, 478–487. doi:10.1038/s41557-019-0233-x
Nakao, Y., Yada, A., Ebata, S., and Hiyama, T. (2007). A dramatic effect of lewis-acid catalysts on nickel-catalyzed carbocyanation of alkynes. J. Am. Chem. Soc. 129, 2428–2429. doi:10.1021/ja067364x
Niu, G., Zheng, X., Zhao, Z., Zhang, H., Wang, J., He, X., et al. (2019). Functionalized acrylonitriles with aggregation-induced emission: Structure tuning by Simple reaction-condition variation, efficient red emission, and two-photon bioimaging. J. Am. Chem. Soc. 141, 15111–15120. doi:10.1021/jacs.9b06196
Oishi, T., Yamaguchi, K., and Mizuno, N. (2009). Catalytic oxidative synthesis of nitriles directly from primary alcohols and ammonia. Angew. Chem. Int. Ed. 48, 6286–6288. doi:10.1002/anie.200900418
Palomo, C., Aiipurua, J. M., and Aurrekoetxea, N. (1990). A stereoselective synthesis of cis-alkenenitriles through Reformatsky-Peterson reaction. Tetrahedron Lett. 31, 2209–2210. doi:10.1016/0040-4039(90)80110-8
Palomo, C., Aizpurua, J. M., Garcia, J. M., Ganboa, I., Cossio, F. P., Lecea, B., et al. (1990). A new version of the Peterson Olefination using bis(trimethylsilyl)methyl derivatives and fluoride ion as catalyst. J. Org. Chem. 55, 2498–2503. doi:10.1021/jo00295a047
Pradal, A., and Evano, G. (2014). A vinylic Rosenmund-von Braun reaction: Practical synthesis of acrylonitriles. Chem. Commun. 50, 11907–11910. doi:10.1039/c4cc05557h
Qi, C., Peng, Y., Ouyang, L., Ren, Y., and Jiang, H. (2017). Base-promoted addition of arylacetonitriles to terminal alkynes: Regio- and stereoselective access to disubstituted acrylonitriles. Adv. Synth. Catal. 359, 1339–1350. doi:10.1002/adsc.201601024
Qin, C., and Jiao, N. (2010). Iron-facilitated direct oxidative C-H transformation of allylarenes or alkenes to alkenyl nitriles. J. Am. Chem. Soc. 132, 15893–15895. doi:10.1021/ja1070202
Randl, S., Gessler, S., Wakamatsu, H., and Blechert, S. (2001). ChemInform abstract: Highly selective cross metathesis with acrylonitrile using a phosphine free Ru-complex. ChemInform 32, 430–432. doi:10.1002/CHIN.200128080
Rokade, B. V., Malekar, S. K., and Prabhu, K. R. (2012). A novel oxidative transformation of alcohols to nitriles: An efficient utility of azides as a nitrogen source. Chem. Commun. 48, 5506–5508. doi:10.1039/c2cc31256e
Seeberger, L. C., and Hauser, R. A. (2009). Levodopa/carbidopa/entacapone in Parkinson’s disease. Expert Rev. Neurother. 9, 929–940. doi:10.1586/ern.09.64
Shen, Y., Zificsak, C. A., Shea, J. E., Lao, X., Bollt, O., Li, X., et al. (2015). Design, synthesis, and biological evaluation of sulfonyl acrylonitriles as novel inhibitors of cancer metastasis and spread. J. Med. Chem. 58, 1140–1158. doi:10.1021/jm501437v
Sirim, M. M., Krishna, V. S., Sriram, D., and Unsal Tan, O. (2020). Novel benzimidazole-acrylonitrile hybrids and their derivatives: Design, synthesis and antimycobacterial activity. Eur. J. Med. Chem. 188, 112010. doi:10.1016/j.ejmech.2019.112010
Solangi, M., Kanwal, , Khan, K. M., Saleem, F., Hameed, S., Iqbal, J., et al. (2020). Indole acrylonitriles as potential anti-hyperglycemic agents: Synthesis, α-glucosidase inhibitory activity and molecular docking studies. Bioorg. Med. Chem. 28, 115605. doi:10.1016/j.bmc.2020.115605
Stuhl, L. S. (1985). Reaction of [Co(CN)5]3- with alkenyl halides in an aprotic medium. J. Org. Chem. 50, 3934–3936. doi:10.1021/jo00220a055
Tarleton, M., Gilbert, J., Robertson, M. J., McCluskey, A., and Sakoff, J. A. (2011). Library synthesis and cytotoxicity of a family of 2-phenylacrylonitriles and discovery of an estrogen dependent breast cancer lead compound. Med. Chem. Commun. 2, 31–37. doi:10.1039/c0md00147c
Thiyagarajan, S., and Gunanathan, C. (2018). Ruthenium-catalyzed α-olefination of nitriles using secondary alcohols. ACS Catal. 8, 2473–2478. doi:10.1021/acscatal.7b04013
Tomioka, T., Sankranti, R., Vaughan, T. G., Maejima, T., and Yanase, T. (2011). An α-diaminoboryl carbanion assisted stereoselective single-pot preparation of α, β-disubstituted acrylonitriles. J. Org. Chem. 76, 8053–8058. doi:10.1021/jo201280x
Wang, T., and Jiao, N. (2014). Direct approaches to nitriles via highly efficient nitrogenation strategy through C-H or C-C bond cleavage. Acc. Chem. Res. 47, 1137–1145. doi:10.1021/ar400259e
Wu, Q., Luo, Y., Lei, A., and You, J. (2016). Aerobic copper-promoted radical-type cleavage of coordinated cyanide anion: Nitrogen transfer to aldehydes to form nitriles. J. Am. Chem. Soc. 138, 2885–2888. doi:10.1021/jacs.5b10945
Yadav, V., Landge, V. G., Subaramanian, M., and Balaraman, E. (2020). Manganese-catalyzed α-olefination of nitriles with secondary alcohols. ACS Catal. 10, 947–954. doi:10.1021/acscatal.9b02811
Yamaguchi, K., Fujiwara, H., Ogasawara, Y., Kotani, M., and Mizuno, N. (2007). A tungsten-tin mixed hydroxide as an efficient heterogeneous catalyst for dehydration of aldoximes to nitriles. Angew. Chem. Int. Ed. 46, 3922–3925. doi:10.1002/anie.200605004
Yang, J., Li, H., Qin, J., Song, F., Zhang, J., Qing, F.-L., et al. (2018). Ligand-accelerated, branch-selective oxidative cyanation of alkenes. Sci. Bull. (Beijing). 63, 1479–1484. doi:10.1016/j.scib.2018.09.005
Yang, X., Arai, S., and Nishida, A. (2013). Catalytic cyanation of carbon-carbon triple bonds through a three-component cross-coupling reaction under nickel catalysis. Adv. Synth. Catal. 355, 2974–2981. doi:10.1002/adsc.201300553
Yang, X., Kim, B. S., Li, M., and Walsh, P. J. (2016). Palladium-catalyzed selective α-alkenylation of pyridylmethyl ethers with vinyl bromides. Org. Lett. 18, 2371–2374. doi:10.1021/acs.orglett.6b00815
Zhang, J., Bellomo, A., Trongsiriwat, N., Jia, T., Carroll, P. J., Spencer, D., et al. (2014). NiXantphos: A deprotonatable ligand for room-temperature palladium-catalyzed cross-couplings of aryl chlorides. J. Am. Chem. Soc. 136, 6276–6287. doi:10.1021/ja411855d
Zhang, L. H., Wu, L., Raymon, H. K., Chen, R. S., Corral, L., Shirley, M. A., et al. (2006). The synthetic compound CC-5079 is a potent inhibitor of tubulin polymerization and tumor necrosis factor-α production with antitumor activity. Cancer Res. 66, 951–959. doi:10.1158/0008-5472.CAN-05-2083
Zhang, T. Y., O'Toole, J. C., and Dunigan, J. M. (1998). An efficient and practical synthesis of diphenyl cyanomethylenephosphonate: Applications to the stereoselective synthesis of cis-α, β-unsaturated nitriles. Tetrahedron Lett. 39, 1461–1464. doi:10.1016/S0040-4039(97)10836-X
Zhang, X., Xie, X., and Liu, Y. (2018). Nickel-catalyzed highly regioselective hydrocyanation of terminal alkynes with Zn(CN)2 using water as the hydrogen source. J. Am. Chem. Soc. 140, 7385–7389. doi:10.1021/jacs.8b02542
Zhang, Z., and Liebeskind, L. S. (2006). Palladium-catalyzed, copper(I)-mediated coupling of boronic acids and benzylthiocyanate. A cyanide-free cyanation of boronic acids. Org. Lett. 8, 4331–4333. doi:10.1021/ol061741t
Zhou, S., Addis, D., Das, S., Junge, K., and Beller, M. (2009). New catalytic properties of iron complexes: Dehydration of amides to nitriles. Chem. Commun. 32, 4883–4885. doi:10.1039/b910145d
Zhou, W., Xu, J., Zhang, L., and Jiao, N. (2010). An efficient transformation from benzyl or allyl halides to aryl and alkenyl nitriles. Org. Lett. 12, 2888–2891. doi:10.1021/ol101094u
Keywords: arylacetonitrile, palladium catalysis, alkenylation, isomerization, aryl acrylonitrile
Citation: Jiang Y, Wang B, Liu D, Xia D, Liu Z, Li L, Deng G and Yang X (2022) Aryl acrylonitriles synthesis enabled by palladium-catalyzed α-alkenylation of arylacetonitriles with vinyl halides/triflates. Front. Chem. 10:1091566. doi: 10.3389/fchem.2022.1091566
Received: 07 November 2022; Accepted: 05 December 2022;
Published: 15 December 2022.
Edited by:
Jian-Wei Dong, Qujing Normal University, ChinaReviewed by:
Keyume Ablajan, Xinjiang University, ChinaCopyright © 2022 Jiang, Wang, Liu, Xia, Liu, Li, Deng and Yang. This is an open-access article distributed under the terms of the Creative Commons Attribution License (CC BY). The use, distribution or reproduction in other forums is permitted, provided the original author(s) and the copyright owner(s) are credited and that the original publication in this journal is cited, in accordance with accepted academic practice. No use, distribution or reproduction is permitted which does not comply with these terms.
*Correspondence: Guogang Deng, Z2dkZW5nQHludS5lZHUuY24=; Xiaodong Yang, eGR5YW5nQHludS5lZHUuY24=
†These authors have contributed equally to this work
Disclaimer: All claims expressed in this article are solely those of the authors and do not necessarily represent those of their affiliated organizations, or those of the publisher, the editors and the reviewers. Any product that may be evaluated in this article or claim that may be made by its manufacturer is not guaranteed or endorsed by the publisher.
Research integrity at Frontiers
Learn more about the work of our research integrity team to safeguard the quality of each article we publish.