- 1Shandong Technology Innovation Center of Molecular Targeting and Intelligent Diagnosis and Treatment, Binzhou Medical University, Yantai, Shandong, China
- 2Yale Center for Molecular and Systems Metabolism, Department of Comparative Medicine, Department of Cellular and Molecular Physiology, Yale University School of Medicine, New Haven, CT, United States
Post-translational modification (PTM) has a significant impact on cellular signaling and function regulation. In pancreatic β cells, PTMs are involved in insulin secretion, cell development, and viability. The dysregulation of PTM in β cells is clinically associated with the development of diabetes mellitus. Here, we summarized current findings on major PTMs occurring in β cells and their roles in insulin secretion. Our work provides comprehensive insight into understanding the mechanisms of insulin secretion and potential therapeutic targets for diabetes from the perspective of protein PTMs.
1 Introduction
Insulin is an anabolic hormone released from pancreatic islet β cells with the distinct capacity to maintain blood glucose homeostasis (Cabrera et al., 2006; Da Silva Xavier, 2018). The secretion of insulin in response to glucose and other nutrients (such as amino acids and free fatty acids) is a complex process involving the coordination of multiple signaling pathways (Nolan et al., 2006; Henquin, 2011). Impairment of this process is directly associated with the development of diabetes mellitus (Schwartz et al., 2013). Thus, the insulin secretion process is considered as a promising target for the treatment of diabetes mellitus (DeFronzo et al., 2014). But the detail of insulin secretion regulation is still an unanswered question.
Post-translational modification (PTM) is the covalent modification with addition or removal of chemical groups on proteins (Walsh et al., 2005). It is closely associated with almost all physiological and pathological processes by regulating protein localization, degradation, and functions (Walsh and Jefferis, 2006; Khan et al., 2016; Morales-Tarre et al., 2021; Zhu and Hart, 2021). Accumulating evidence suggests that PTMs are extensively involved in the insulin secretion process, Currently, at least eight types of PTMs are known associated with insulin secretion. For example, phosphorylation is required for signaling cascades mediating insulin secretion (Campbell and Newgard, 2021). SUMOylation and palmitoylation have been reported to regulate insulin secretion at multiple stages (Davey et al., 2019; Chamberlain et al., 2021). Acetylation, ubiquitination and O-GlcNAcylation are involved in insulin gene transcription (Mounier and Posner, 2006; Ozcan et al., 2010; Sampley and Ozcan, 2012). Even some understudied PTMs such as citrullination and deamidation are recently reported to be linked with insulin secretion. These indicate that protein PTM plays a critical role in the regulation of insulin secretion in β cells. However, a systematic review of current findings of PTM in insulin secretion is still missing. Here, we review the current understanding of the functional roles of these PTMs in the insulin secretion process.
2 Insulin secretion
The main physiological stimulus for insulin secretion is blood glucose. Activation of insulin secretion by elevated glucose concentration is called glucose-stimulated insulin secretion (GSIS) (Figure 1). It includes two tandem pathways, the triggering pathway and the amplifying pathway. In the triggering pathway, glucose is transported into islet β cells via glucose transporters (GLUTs) and converted to glucose-6-phosphate (G-6-P) by glucokinase (GK). G-6-P further enters the tricarboxylic acid (TCA) cycle through glycolysis, leading to the production of adenosine triphosphate (ATP). Increased ATP/adenosine diphosphate (ADP) ratio in the cytoplasm causes the closure of the KATP channel (composed of SUR1 subunits and Kir6.2 subunits) in the cell membrane (Seino, 1999). This leads to the generation of electro-voltage between the inside and outside of the cell membrane, opening voltage-dependent Ca2+ channels (VDCCs) and inducing calcium influx (Lim et al., 2009). Subsequently, calcium influx is sensed by multiple calcium-binding proteins and triggers insulin granules exocytosis. The amplifying pathway increases the sensitivity of insulin secretion to the induced calcium influx, which is independent of the KATP channel (Kalwat and Cobb, 2017). Therefore, the amplifying pathway is also referred to the KATP-independent pathway. However, the exact mechanisms of action of the amplifying pathway are not fully clear (Kalwat and Cobb, 2017). Store-operated calcium channels (SOCs) are believed as a critical compartment of the amplifying pathway. SOCs can be activated by Ca2+ depletion from the endoplasmic reticulum (ER) (Prakriya and Lewis, 2015; Lopez et al., 2020). Upon the depletion of ER Ca2+ store, stromal interaction molecule 1 (STIM1) aggregates and translocates to the plasma membrane, where it interacts directly with Calcium Release-Activated Calcium Modulator 1 (Orai1) and transient receptor potential canonical channel-1 (TRPC1). This interaction opens SOCs and initiates Ca2+ influx, which induces insulin secretion (Tian et al., 2012b). Another critical compartment in the amplifying pathway is the second messenger cyclic adenosine monophosphate (cAMP) (Tian et al., 2015). The cAMP signaling mediates insulin secretion induced by glucose, free fatty acid (FFA) and Glucagon and glucagon-like peptide-1 (GLP-1) (Dyachok et al., 2008; Tian et al., 2011; Tian et al., 2012a).
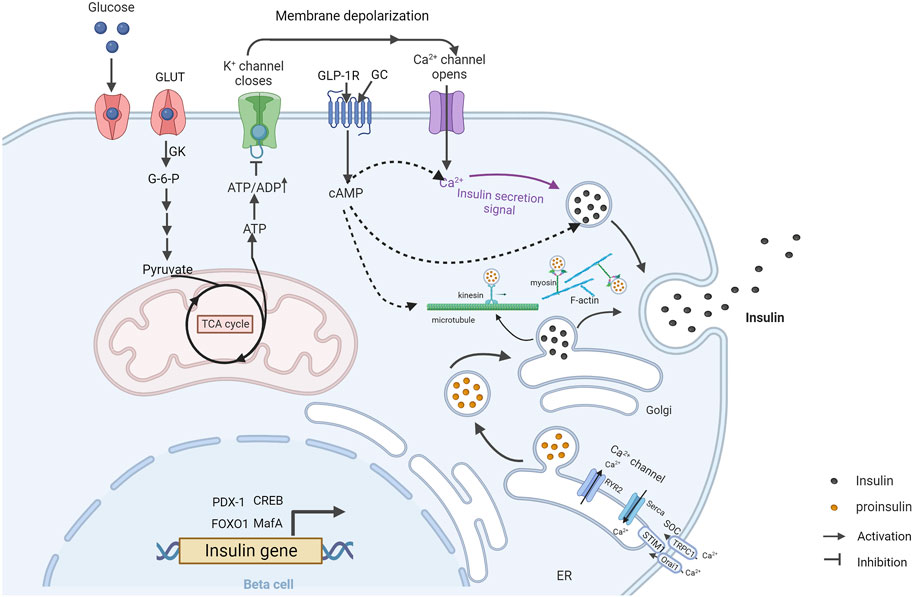
FIGURE 1. Overview of glucose-stimulated insulin secretion in β cells (Created with BioRender.com). GSIS is mediated by the triggering pathway (solid arrows) and amplification pathways (dashed arrows). At high blood glucose levels, glucose is taken up and converted to glucose-6-phosphate through GK. Glucose-6-phosphate is metabolized by glycolysis and subsequently enters the tricarboxylic acid cycle, triggering an increase in intracellular ATP. Increasing ATP/ADP ratio leads to the closure of the KATP channel and the opening of Ca2+ channels, allowing calcium influx and triggering insulin granules exocytosis. Calcium depletion from ER causes STIM1 to accumulate at ER-plasma membrane junctions where it gates Orai1 and TRPC1, leading to calcium influx and subsequent insulin granules exocytosis. GLP-1 stimulates insulin secretion through cAMP signal pathway. Insulin-vesicles are transported by motor proteins along cytoskeletal biopolymers. Insulin gene transcription is regulated by multiple transcription factors. GC, glucagon; GK, glucokinase; ER, endoplasmic reticulum.
Insulin secretion in response to glucose stimulation occurs in a biphasic manner composed of a transient first phase and followed a prolonged second phase. Released insulin granules in the first-phase are mainly from a “readily releasable pool” (RRP) in the vicinity of the plasma membrane. Released insulin granules in the second phase are mainly recruited from intracellular storage pools. Actin filaments and microtubules are two main transport routes for insulin granules in β cells (Thurmond et al., 2003; Varadi et al., 2003; Varadi et al., 2005; Cui et al., 2011). Actin filaments mainly contribute to the short-range movement near the plasma membrane, whereas microtubules contribute to the long-range movements (Omar-Hmeadi and Idevall-Hagren, 2021). The remodeling of F-actin cytoskeleton and microtubule network is critical for GSIS in β cells (Thurmond et al., 2003; Bracey et al., 2020; Ho et al., 2020; Wang et al., 2020). Moreover, the formation of SNARE complex which is composed of t-SNARE proteins (SNAP-25 and syntaxin) in the plasma membrane and v-SNARE protein (VAMP) on the insulin granules membrane, is also important for insulin exocytosis.
3 Regulatory effect of PTMs on insulin secretion
Various PTMs related to insulin secretion have been reported. In the following sections, we will outline currently known PTMs and related substrates in β cells (Table 1), and discuss the roles of individual PTMs in the regulation of insulin secretion (Figure 2).
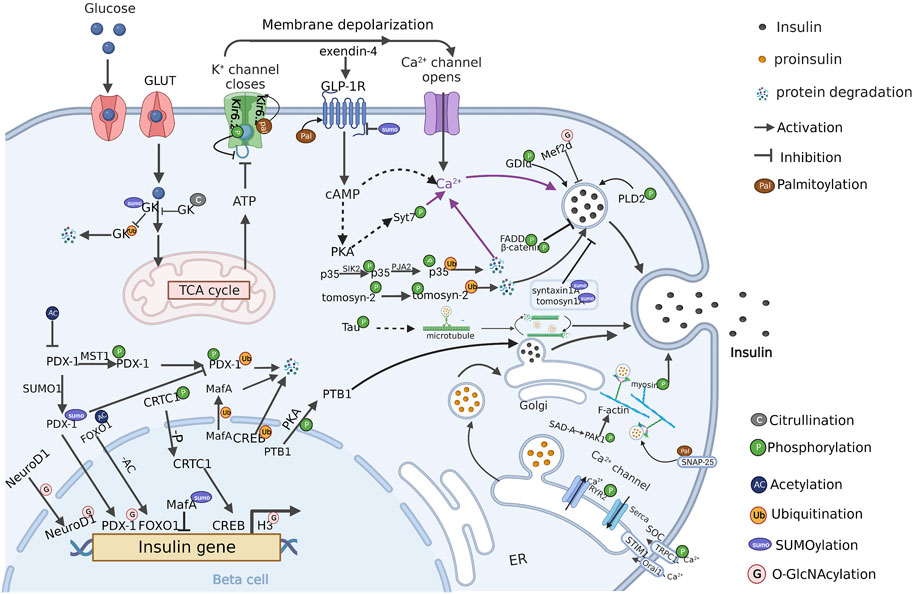
FIGURE 2. PTM regulation of insulin secretion in β cells (Created with BioRender.com). PTMs are ubiquitously engaged in the various stages of insulin secretion, including signal regulation, insulin gene transcription, insulin-vesicles transportation. Multiple PTMs synergistically regulate insulin secretion in pancreatic β cells. The crosstalk between these PTMs and the modified protein substrates currently known is present in the figure. P: Phosphorylation, AC: Acetylation, Ub: Ubiquitination, SUMO: SUMOylation, G: O-GlcNAcylation, Pal: Palmitoylation, C: Citrullination.
3.1 Phosphorylation
Phosphorylation is a ubiquitous PTM regulated by protein kinases and phosphatases that transfer and remove phosphate groups from ATP or GTP to amino acid residues (Ser, Tyr, Thr) of proteins, respectively. It is widely involved in signal transduction pathways and associated with various physiological and pathological processes by regulating cell proliferation, development, differentiation, apoptosis, and other cell processes (Humphrey et al., 2015). Phosphorylation mediated signaling cascades play an important role in maintaining glucose homeostasis (Roder et al., 2016). The insulin/insulin receptor and their downstream proteins, such as PI3K/AKT and ERK, regulate various metabolic pathways including improving glucose tolerance and protecting against insulin resistance (Dall'Agnese et al., 2022). In the insulin secretion process, phosphorylation is wildly existing in the insulin biosynthesis and signaling cascade of insulin exocytosis (Sacco et al., 2016).
Transcription factors are required in insulin gene transcription, and regulated by phosphorylation (Knoch et al., 2006; Ch’ng et al., 2012; Malm et al., 2016). cAMP-Regulated Transcriptional Co-activator-1 (CRTC1) is a cAMP-responsive element binding protein (CREB)-mediated transcription regulator. Under basal conditions, CRTC is phosphorylated by Salt Inducible Kinase (SIK) and binds to 14-3-3 proteins, sequestering in the cytoplasm (Ch’ng et al., 2012; Malm et al., 2016). Dephosphorylation of CRTC by activating CRTC phosphatase or inhibiting CRTC kinases triggers its dissociation from 14-3-3 and translocation into the nucleus, where it activates CREB-mediated transcription of Ins1 gene (Oetjen et al., 1994; Altarejos et al., 2008; Altarejos and Montminy, 2011). It has been reported that this process is involved in GLP-1-stimulated insulin secretion (Shin et al., 2014). Cytosolic polypyrimidine tract-binding protein 1 (PTB1) binds and stabilizes mRNA encoding secretory granules (SGs) (Knoch et al., 2004). PKA-dependent PTB1 phosphorylation is promoted by elevated cAMP and in turn promotes SG expression and insulin secretion (Knoch et al., 2006).
In β cells, Ca2+ is considered as triggering signal of insulin exocytosis. Phosphorylation is involved in the depolarization of membrane potential and calcium influx. Kir6.2, the KATP channel component, can be phosphorylated on Ser385 by AMPK. Phosphorylated Kir6.2 restrains KATP channel activity and leads to depolarization of membrane potential. This depolarization results in the opening of VDCCs, leading to elevated intracellular calcium levels and ultimately induced insulin secretion (Chang et al., 2009). Synaptotagmin-7 (Syt7) is a major cytoplasmic Ca2+ sensor for exocytosis by triggering secretory granule fusion and insulin secretion (Gao et al., 2000; Gauthier et al., 2008). It is reported that GLP-1 stimulates PKA-dependent phosphorylation of Syt7 at Ser103. Such modification enhances Ca2+-triggered exocytosis, whereas dephosphorylation of Syt7 disrupts GLP-1 potentiation of insulin secretion (Wu et al., 2015). Besides cytoplasmic Ca2+ balance, calcium homeostasis in the ER also has a pivotal role in insulin secretion (Sabourin et al., 2015; Li et al., 2021). Ryanodine receptors (RyRs) are vital components of ER intracellular calcium channels which mediate Ca2+ release from ER into the cytoplasm (Doser et al., 2020). RyR2 is one of the RyRs isoforms that is expressed in β cells (Johnson et al., 2004; Dror et al., 2008; Takasawa et al., 2010). It has been shown that sufficient phosphorylation of RyR2 and subsequent Ca2+ release are essential steps in GSIS (Dixit et al., 2013; Llanos et al., 2015). However, hyperphosphorylation of RyR2 induced by CaMKII leads to glucose intolerance, impaired GSIS and lowered [(Ca2+)]cyt transients. This is due to the increased basal RyR2-mediated Ca2+ leak and basal hyperinsulinemia (Dixit et al., 2013). Moreover, components of SOCs can be phosphorylated. TRPC1, as a component of SOCs, can be phosphorylated by protein kinase C (PKC). Inhibition of PKC activity reduces TRPC1 phosphorylation and decreases insulin secretion rapidly, which could be restored by the TRPC1 activator (Xu et al., 2019).
Insulin granules transport and release also can be regulated by protein phosphorylation (Ho et al., 2020). Myosin is a motor protein that is responsible for insulin granules transportation dependent on F-actin (Varadi et al., 2005; Sweeney and Holzbaur, 2018; Omar-Hmeadi and Idevall-Hagren, 2021). It is composed of heavy chains (MHC) and two types of light chains (MLC) (Penn et al., 1982; Niki et al., 1993). Both MHC and MLC can be phosphorylated by multiple protein kinase including Myosin light-chain kinase (MLCK), and promote insulin secretion (Tan et al., 1992; Iida et al., 1997; Wilson et al., 1999). Tau is a microtubule-associated protein (MAP) involved with insulin secretion and glycemic control (Maj et al., 2016; Wijesekara et al., 2018). High glucose induces hyper-phosphorylation of tau by multiple kinases including GSK3, PKA, PKC, and CDK5, which enhances microtubule turnover to acutely induces GSIS (Ho et al., 2020). β-catenin, as a cell-cell adhesion protein, increases GSIS through promoting the rearrangement of actin cytoskeleton (Sorrenson et al., 2016). It can be phosphorylated at Ser552 by p21-activated protein kinase-1 (PAK1) in response to glucose and GLP-1 stimulation. A mutation of Ser552 to Ala on β-catenin attenuates GSIS, suggesting a critical role for β-catenin Ser552 phosphorylation in insulin secretion (Sorrenson et al., 2021). Tomosyn is a negative regulator of cellular exocytosis and can hinder insulin secretion (Zhang et al., 2006; Bhatnagar et al., 2011). In response to high glucose, enhanced tomosyn-2 phosphorylation targets tomosyn-2 for Hrd-1-mediated ubiquitination and degradation, causing increased insulin secretion (Bhatnagar et al., 2014). Activated Phospholipase D2 (PLD2) can stimulate insulin secretion (Jones et al., 1999). PLD2 phosphorylation at Ser134 via cell cycle protein-dependent kinase 5 (CDK5) plays an influential role in EGF-dependent insulin secretion (Lee et al., 2008). Brain-selective kinase 2 (BRSK2), also known as SAD-A, is a member of the AMPK-related kinase family. It is abundantly expressed in pancreatic islets β cells and acts a key function in insulin secretion (Lizcano et al., 2004). Overexpression of SAD-A significantly enhances GSIS and further potentiates GLP-1’s effect on GSIS in isolated mouse islets (Nie et al., 2013). Phosphorylation of PAK1 at Thr423 via SAD-A triggers the onset of GSIS in islet β cells (Nie et al., 2012). Phosphorylation of GDP-dissociation inhibitors (GDIα) at Ser174 by SAD-A leads to the dissociation of Rho GTPases from GDIα complexes, culminating in insulin exocytosis (Nie et al., 2018).
Phosphorylation also presents an inhibitory role in insulin secretion. Fas-associated death domain protein (FADD) is a classical adaptor in Fas-FasL signaling, which can regulate islet mass and insulin secretion. In the mouse model of FADD-D (S191D), which mimics the constitutive expression of phosphorylated FADD in mice, the area of pancreatic islets is shrunken, and GSIS is impaired. This suggests that FADD phosphorylation negatively regulates islet development and insulin secretion (Yao et al., 2015).
3.2 Acetylation
Acetylation is a chemical reaction in which an acetyl group is added to a compound in place of a hydrogen atom, which can be regulated by histone acetyltransferases (HATs) and histone deacetylases (HDACs) (Gao et al., 2017). Acetylation not only regulates histones, transcription factors, and epigenetic regulators, but also regulates many enzymes in metabolic pathways such as glycolysis, gluconeogenesis, tricarboxylic acid cycle, and fatty acid oxidation (Guan and Xiong, 2011).
Protein acetylation is tightly linked to insulin secretion and functional regulation of pancreatic β cells (Zhang et al., 2019). As a class of highly conserved deacetylases, Sirtuins (SIRTs) significantly contribute to insulin secretion. Among the SIRT family, SIRT1 is located in the nucleus, and ameliorates hyperglycemia by promoting insulin secretion and β cell expansion (Luu et al., 2013). SIRT2 is the only predominantly cytoplasmic isoform but is also found in the nucleus and mitochondria (Vaquero et al., 2006; Liu et al., 2017). SIRT2 knockout rats exhibit impaired glucose tolerance and decreased GSIS (Moynihan et al., 2005). SIRT3 and SIRT4 are mainly localized in the mitochondria. Overexpression of SIRT3 inhibits acetylation and degradation of trifunctional enzyme subunit alpha (ECHA) that participated in fatty acid β-oxidation, resulting in increased β-oxidation of fatty acid and reduced oxidation of glucose in β cells. SIRT3 knockout mice show increased insulin secretion upon glucose stimulation (Zhang et al., 2019). SIRT4 regulates amino acid catabolism and insulin secretion, maintaining glucose homeostasis during aging (Haigis et al., 2006; Anderson et al., 2017). SIRT6 is mainly localized in the nucleus. Pancreatic β cell-specific SIRT6 knockout mice show significantly reduced GSIS (Xiong et al., 2016).
Most known acetylated proteins contributing to insulin secretion are transcription factors. Pancreatic and duodenal homeobox-1 (PDX-1) and FOXO1 are two transcription factors associated with insulin gene transcription (Sussel et al., 1998; Berneman-Zeitouni et al., 2014). The acetylated form of FOXO1 resides in the cytoplasm while the deacetylated form is mainly in the nucleus. Deacetylation of FOXO1 enhances its transcriptional activity and plays an essential role in insulin signaling (Accili and Arden, 2004). Moreover, insulin secretion is enhanced in deacetylated FOXO1 (6 KR) knock-in mice (Kim-Muller et al., 2016). lncRNA MALAT1 impairs insulin secretion by reducing histone H3 acetylation at the Pdx-1 promoter and subsequently inhibiting Pdx-1 expression (Ding et al., 2020).
3.3 Ubiquitination
Ubiquitination is regulated by a three-step cascade: ubiquitin activation by E1 enzymes, conjugation by E2 enzymes, and ligation by E3 ligases (Komander and Rape, 2012). The Ubiquitin-proteasome system (UPS) is the main pathway for intracellular protein degradation. It plays a crucial role in the regulation of glucose homeostasis, insulin secretion, and the pathogenesis of diabetes mellitus (Price et al., 1996; Kawaguchi et al., 2006; Song et al., 2013; Uruno et al., 2013; Al-Khalili et al., 2014; Yang et al., 2016; Balaji et al., 2018; Yamada et al., 2018).
A group of critical proteins involved in insulin secretion is regulated by ubiquitination-mediated degradation. GK degradation by ubiquitination inhibits insulin secretion by decreasing G-6-P production (Cho et al., 2020). The proteasome inhibitor lactacystin has been shown to enhance GSIS in a 2-h short-term treatment (Lopez-Avalos et al., 2006). The v-maf musculoaponeurotic fibrosarcoma oncogene homolog A (MafA) is a key transcription factor required for β cells formation and function (Zhang et al., 2005; Andrali et al., 2008; Kaneto and Matsuoka, 2015). MafA deletion in mice causes glucose intolerance and induces diabetes. The E3 ubiquitin ligase Hrd-1 targets MafA for ubiquitination and degradation in β cells, which results in cytoplasmic accumulation of MafA. Such accumulation impairs its function in the nucleus, causing reduced insulin secretion (Wu et al., 2020). CREB is a transcription factor that is essential for glucose homeostasis and β cell survival (Jambal et al., 2003; Jhala et al., 2003; Sarkar et al., 2007). Chronic hyperglycemia increases CREB ubiquitination and decreases protein expression which ultimately inhibits insulin secretion (Costes et al., 2009). Somatostatin receptor subtype 5 (SSTR5) inhibits PDX-1 expression by downregulating Pdx-1 transcription and enhancing PDX-1 ubiquitination at post-translational level, thus reducing insulin secretion (Zhou et al., 2012). Phosphorylation of PDX-1 at Thr11 by Macrophage Stimulating 1 (MST1) in β cells leads to PDX-1 ubiquitination and degradation, resulting in impaired insulin secretion (Ardestani et al., 2014). A previous report showed that silencing CDK5 activator CDK5R1 (also known as p35) enhances insulin secretion in MIN6 cells in high glucose culture (Wei et al., 2005). Subsequent research showed that p35 phosphorylation via Salt-inducible kinase 2 (SIK2) induces p35 ubiquitylation. The modification is mediated by the E3 ubiquitin ligase PJA2, which leads to the activation of calcium entry and insulin secretion (Sakamaki et al., 2014).
3.4 SUMOylation
SUMOylation is a post-translational modification of small SUMO proteins that is catalyzed by activating enzyme (E1), conjugating enzyme (E2), and ligase (E3), and reversed by specific proteases such as Sentrin-specific SUMO proteases (SENPs) (Li et al., 2005; Flotho and Melchior, 2013). It plays an important role in modulating protein activity, protein-protein interactions, and subcellular localization (Shao and Cobb, 2009; Gareau and Lima, 2010). It has been reported that the homeostasis of SUMOylation plays an essential role in maintaining β cell function (Dai et al., 2011; Rajan et al., 2012; Vergari et al., 2012; Davey et al., 2019).
E2 SUMO-conjugating enzyme (UBC9) is the only conjugating enzyme essential for the SUMO system. Mice lacking UBC9 in β cells exhibit decreased insulin content and loss of β cell mass. In contrast, overexpression of UBC9 in β cells leads to an increased antioxidant ability but impaired insulin secretion (He et al., 2018). Protein deSUMOylation by SENPs regulates the conjugation/deconjugation balance of target proteins. Studies on islet-specific SENP1 deletion in mice further demonstrated that the knockdown of SENP1 reduces Ca2+-triggered β cell exocytosis. Conversely, overexpression of SENP1 augments β cell exocytosis (Vergari et al., 2012). It suggests a key role for SUMOylation/deSUMOylation balance in GSIS.
SUMOylation regulates insulin secretion at multiple stages. In the insulin gene transcription process, SUMOylation reduced transcriptional activity of MafA toward the insulin gene promoter in low glucose (2 mm) or exposure to hydrogen peroxide (Shao and Cobb, 2009). However, SUMOylation protects PDX-1 from proteasomal degradation and promotes its entry into the nucleus, where it activates insulin gene transcription (Kishi et al., 2003). SUMOylation also participates in triggering insulin secretion pathway. Overexpression of SUMO-1 in β cells increases the stability and activity of GK to induce the closure of the KATP channel (Aukrust et al., 2013), meanwhile exert a strong inhibitory action on the Kv2.1 voltage-dependent K+ channel (MacDonald et al., 2001; MacDonald et al., 2002; Dai et al., 2009). The depolarization of cell membrane promotes the activation of calcium channels and subsequent insulin secretion. GLP-1 activates the GLP-1 signaling by the interaction with GLP1 receptor (GLP-1R) in β cells, resulting in a rapid increase in intracellular cAMP that promotes insulin secretion. Overexpression of SUMO-1 attenuates GLP-1R function by preventing GLP-1R oligomerization which is essential for forward trafficking, leading to a significant reduction in insulin secretion (Rajan et al., 2012). SUMOylation has been proposed to act as a ‘brake’ on insulin exocytosis. Some proteins associated with insulin exocytosis have been reported to be SUMOylated such as tomosyn1A and syntaxin 1A. SUMOylation increases the interaction between tomosyn1A and syntaxin 1A, which sequesters syntaxin 1A to inhibits the formation of SNARE complex and subsequent insulin exocytosis (Ferdaoussi et al., 2017; Davey et al., 2019). Moreover, SUMOylation suppresses Syt7-mediated insulin secretion, which is transiently lost upon glucose stimulation and returns within 30–60 min (Dai et al., 2011).
3.5 O-GlcNAcylation
O-GlcNAcylation is a unique protein glycosylation that is controlled bidirectionally by two enzymes: the writer O-GlcNAc transferase (OGT) and the eraser O-GlcNAcase (OGA). As a nutrition sensor, O-GlcNAcylation is closely associated with type 2 diabetes and associated complications (Dias and Hart, 2007).
O-GlcNAc homeostasis in β cells plays a notable role in insulin secretion (Sireesh et al., 2014; Humphrey et al., 2015; Wende, 2015; Yang and Qian, 2017; Yoshida et al., 2022). OGT is expressed virtually in all cell types but is particularly high in pancreatic β cells (Hanover et al., 1999; Hart and Akimoto, 2009). β cell-specific OGT deletion leads to reduced insulin secretion, and this effect is more pronounced in high fat diet-fed mice (Lockridge et al., 2020). OGA acts in opposition to OGT to regulate protein O-GlcNAcylation. Research has shown that overexpression of OGA in β cells decreases insulin secretion and impairs glucose tolerance in mice (Soesanto et al., 2011). Paradoxically, OGA deletion in pancreatic β cells also impairs insulin secretion in vivo and in vitro (Yoshida et al., 2022).
Currently, most identified substrates of O-GlcNAcylation are related to insulin gene transcription. Increased nuclear O-GlcNAcylation increases intracellular insulin levels and reserves GSIS in part by boosting histone H3 transcriptional activation to promote Ins1 and Ins2 gene transcription in Min6 cells (Durning et al., 2016). Glucose induced PDX-1 O-GlcNAcylation leads to increased PDX-1 DNA binding activity and insulin secretion in Min6 cells (Gao et al., 2003). Further studies demonstrated that OGT interacts with phosphatidylinositol 3,4,5-trisphosphate (PIP3), which enables OGT to catalyze O-GlcNAcylation of nuclear proteins, including PDX-1 (Yang et al., 2008; Kebede et al., 2012). The localization of NeuroD1, a transcription factor of the insulin gene, is regulated by O-GlcNAcylation (Peterson and Hart, 2016). Under low glucose conditions, NeuroD1 is mainly in the cytosol. However, OGA inhibitor treatment induces NeuroD1 translocation into the nucleus, leading to enhanced insulin expression (Andrali et al., 2007). Another transcription factor, myocyte enhancer factor 2D (Mef2d), has been reported to negatively regulate insulin secretion through O-GlcNAcylation (Yoshida et al., 2022).
3.6 Palmitoylation
Palmitoylation, the attachment of fatty acyl chains to cysteine residues, is a reversible process mediated by the opposing activities of acyltransferases and thioesterases (Chamberlain and Shipston, 2015). Palmitoylation is a recently discovered PTM that plays a significant role in the regulation of cellular functions (Blaskovic et al., 2014; Chamberlain and Shipston, 2015). Recent studies have revealed the emerging importance of palmitoylation in insulin secretion and insulin response pathways (Chamberlain et al., 2021; Dong et al., 2023). Acyl-protein thioesterase 1 (APT1) is a depalmitoylation enzyme. The function of APT1 was blocked in chronic hyperglycemia, leading to defective insulin secretion (Chamberlain et al., 2021). APT1 knockdown in islets caused insulin hypersecretion (Dong et al., 2023).
In β-cells, palmitoylation are widely present in ion channels and insulin exocytosis. Kir6.2 is palmitoylated at cysteine-166, which increase the open state of the KATP channel and lead to inhibitory insulin secretion (Chamberlain et al., 2021). β2a, an important auxiliary subunit of VDCCs, is palmitoylated at cysteine-3/4, thus increasing plasma membrane trafficking of β2a and Cav subunits of VDCCs. However, excessive number of palmitoylated CaVβ2a leads to Ca2+ overload and β cell death (Kazim et al., 2017). Palmitoylation of SNAP-25 in the central region increase its membrane localization, which may help insulin exocytosis (Gonelle-Gispert et al., 2000). Scamp1, which is localized in insulin secretory granules, can be palmitoylated at Cys132. Palmitoylation-defective Scamp1 mutant C132S rescues insulin hypersecretion and nutrient-induced apoptosis in APT1-deficient cells (Dong et al., 2023). GLP-1R is palmitoylated mainly at Cys438 in response to agonists, inducing GLP-1R clustering, nanodomain signaling, and internalization. These result in enhanced GLP-1R signaling and insulin secretion (Buenaventura et al., 2019).
3.7 Other PTMs
Besides the PTM types described above, a group of less-studied PTM types is also related to insulin secretion. Citrullination is mediated by calcium-dependent peptidyl arginine deiminases (PADs), which catalyze deimination, the conversion of arginine into non-classical amino acid citrulline (Kunieda et al., 2018). A major role for PADs and citrullinated proteins has emerged in type 1 diabetes (Yang et al., 2021). Citrullination alters the enzyme kinetics of GK and suppresses GSIS (Yang et al., 2022).
Deamidation is the conversion of glutamine to glutamic acid by transglutaminase (TGM) enzymes (Callebaut et al., 2022). Deamidated peptides have been reported as autoantigens in type 1 diabetes (Callebaut et al., 2022). Islet amyloid polypeptide (IAPP) accelerates the pathogenesis of type 2 diabetes by exacerbating β cell degeneration and ultimately compromising insulin secretion. Studies have revealed that deamidation can modulate IAPP amyloid formation and fibril morphology, which induces its cytotoxicity (Nguyen et al., 2017).
4 Conclusion and perspectives
In this review, we summarized the current progress of PTM regulation in insulin secretion. Eight PTMs and at least twenty-nine modified protein substrates have been reported to be associated with insulin secretion, suggesting that insulin secretion is extensively regulated by PTMs. However, there remains numerous unanswered questions about the role of PTMs in insulin secretion. For example, currently more than 600 PTMs have been identified in eukaryotes (Bradley, 2022). Whether there are more PTMs and more modified substrates present in β cells? What’s the function? Furthermore, it is noticed that some key proteins are regulated by multiple PTMs with consistent or contrary effect. What’s the crosstalk between the different PTMs? How these PTMs synergistically regulate the function of protein? At last, defective or excessive PTMs may induce insulin deficiency, or insulin hypersecretion which may lead to β cells failure, resulting impaired insulin secretion. How the PTMs are dynamically regulated in the physiologic or pathological insulin secretion process? Moreover, several drug candidates for T2DM have been proved to regulate protein PTMs. For example, Glucokinase activators (GKAs), including dorzagliatin, MK-0941 and AZD1656, also promotes SUMOylation of pancreatic glucokinase, (Wu et al., 2023), implying PTM can be the potential drug targets for T2DM. Thus, further in-depth studies of PTMs in insulin secretion are in need.
Author contributions
The design of study was done by GT and XY; data mining was performed by CY, MW, YZ, ZY, and MS; interpretation of the obtained information was done by CY, MW, and YZ; figures were drawn by MW and YZ; the manuscript was written by CY, and MW, and revised by JM, GT, and XY; all authors contributed to the article and approved the submitted version.
Funding
This research was funded by Taishan Scholars Construction Engineering, National Natural Science Foundation of China (32170989), Major Basic Research Project of Shandong Provincial Natural Science Foundation (ZR2019ZD27), Natural Science Foundation of Shandong Province (ZR2021MH141) and Shandong Province Higher Educational Youth Innovation Science and Technology Program (2019KJE013).
Conflict of interest
The authors declare that the research was conducted in the absence of any commercial or financial relationships that could be construed as a potential conflict of interest.
Publisher’s note
All claims expressed in this article are solely those of the authors and do not necessarily represent those of their affiliated organizations, or those of the publisher, the editors and the reviewers. Any product that may be evaluated in this article, or claim that may be made by its manufacturer, is not guaranteed or endorsed by the publisher.
References
Accili, D., and Arden, K. C. (2004). FoxOs at the crossroads of cellular metabolism, differentiation, and transformation. Cell 117 (4), 421–426. doi:10.1016/s0092-8674(04)00452-0
Al-Khalili, L., de Castro Barbosa, T., Ostling, J., Massart, J., Cuesta, P. G., Osler, M. E., et al. (2014). Proteasome inhibition in skeletal muscle cells unmasks metabolic derangements in type 2 diabetes. Am. J. Physiol. Cell Physiol. 307 (9), C774–C787. doi:10.1152/ajpcell.00110.2014
Altarejos, J. Y., Goebel, N., Conkright, M. D., Inoue, H., Xie, J., Arias, C. M., et al. (2008). The Creb1 coactivator Crtc1 is required for energy balance and fertility. Nat. Med. 14 (10), 1112–1117. doi:10.1038/nm.1866
Altarejos, J. Y., and Montminy, M. (2011). CREB and the CRTC co-activators: Sensors for hormonal and metabolic signals. Nat. Rev. Mol. Cell Biol. 12 (3), 141–151. doi:10.1038/nrm3072
Anderson, K. A., Huynh, F. K., Fisher-Wellman, K., Stuart, J. D., Peterson, B. S., Douros, J. D., et al. (2017). SIRT4 is a lysine deacylase that controls leucine metabolism and insulin secretion. Cell Metab. 25 (4), 838–855.e15. doi:10.1016/j.cmet.2017.03.003
Andrali, S. S., Qian, Q., and Ozcan, S. (2007). Glucose mediates the translocation of NeuroD1 by O-linked glycosylation. J. Biol. Chem. 282 (21), 15589–15596. doi:10.1074/jbc.M701762200
Andrali, S. S., Sampley, M. L., Vanderford, N. L., and Ozcan, S. (2008). Glucose regulation of insulin gene expression in pancreatic beta-cells. Biochem. J. 415 (1), 1–10. doi:10.1042/BJ20081029
Ardestani, A., Paroni, F., Azizi, Z., Kaur, S., Khobragade, V., Yuan, T., et al. (2014). MST1 is a key regulator of beta cell apoptosis and dysfunction in diabetes. Nat. Med. 20 (4), 385–397. doi:10.1038/nm.3482
Aukrust, I., Bjorkhaug, L., Negahdar, M., Molnes, J., Johansson, B. B., Muller, Y., et al. (2013). SUMOylation of pancreatic glucokinase regulates its cellular stability and activity. J. Biol. Chem. 288 (8), 5951–5962. doi:10.1074/jbc.M112.393769
Balaji, V., Pokrzywa, W., and Hoppe, T. (2018). Ubiquitylation pathways in insulin signaling and organismal homeostasis. Bioessays 40 (5), e1700223. doi:10.1002/bies.201700223
Berneman-Zeitouni, D., Molakandov, K., Elgart, M., Mor, E., Fornoni, A., Dominguez, M. R., et al. (2014). The temporal and hierarchical control of transcription factors-induced liver to pancreas transdifferentiation. PLoS One 9 (2), e87812. doi:10.1371/journal.pone.0087812
Bhatnagar, S., Oler, A. T., Rabaglia, M. E., Stapleton, D. S., Schueler, K. L., Truchan, N. A., et al. (2011). Positional cloning of a type 2 diabetes quantitative trait locus; tomosyn-2, a negative regulator of insulin secretion. PLoS Genet. 7 (10), e1002323. doi:10.1371/journal.pgen.1002323
Bhatnagar, S., Soni, M. S., Wrighton, L. S., Hebert, A. S., Zhou, A. S., Paul, P. K., et al. (2014). Phosphorylation and degradation of tomosyn-2 de-represses insulin secretion. J. Biol. Chem. 289 (36), 25276–25286. doi:10.1074/jbc.M114.575985
Blaskovic, S., Adibekian, A., Blanc, M., and van der Goot, G. F. (2014). Mechanistic effects of protein palmitoylation and the cellular consequences thereof. Chem. Phys. Lipids 180, 44–52. doi:10.1016/j.chemphyslip.2014.02.001
Bracey, K. M., Ho, K. H., Yampolsky, D., Gu, G., Kaverina, I., and Holmes, W. R. (2020). Microtubules regulate localization and availability of insulin granules in pancreatic beta cells. Biophys. J. 118 (1), 193–206. doi:10.1016/j.bpj.2019.10.031
Bradley, D. (2022). The evolution of post-translational modifications. Curr. Opin. Genet. Dev. 76, 101956. doi:10.1016/j.gde.2022.101956
Buenaventura, T., Bitsi, S., Laughlin, W. E., Burgoyne, T., Lyu, Z., Oqua, A. I., et al. (2019). Agonist-induced membrane nanodomain clustering drives GLP-1 receptor responses in pancreatic beta cells. PLoS Biol. 17 (8), e3000097. doi:10.1371/journal.pbio.3000097
Cabrera, O., Berman, D. M., Kenyon, N. S., Ricordi, C., Berggren, P. O., and Caicedo, A. (2006). The unique cytoarchitecture of human pancreatic islets has implications for islet cell function. Proc. Natl. Acad. Sci. U. S. A. 103 (7), 2334–2339. doi:10.1073/pnas.0510790103
Callebaut, A., Bruggeman, Y., Zamit, C., Sodre, F. M. C., Irla, M., Mathieu, C., et al. (2022). Aberrant expression of transglutaminase 2 in pancreas and thymus of NOD mice underscores the importance of deamidation in neoantigen generation. Front. Endocrinol. (Lausanne) 13, 908248. doi:10.3389/fendo.2022.908248
Campbell, J. E., and Newgard, C. B. (2021). Mechanisms controlling pancreatic islet cell function in insulin secretion. Nat. Rev. Mol. Cell Biol. 22 (2), 142–158. doi:10.1038/s41580-020-00317-7
Ch'ng, T. H., Uzgil, B., Lin, P., Avliyakulov, N. K., O'Dell, T. J., and Martin, K. C. (2012). Activity-dependent transport of the transcriptional coactivator CRTC1 from synapse to nucleus. Cell 150 (1), 207–221. doi:10.1016/j.cell.2012.05.027
Chamberlain, L. H., Shipston, M. J., and Gould, G. W. (2021). Regulatory effects of protein S-acylation on insulin secretion and insulin action. Open Biol. 11 (3), 210017. doi:10.1098/rsob.210017
Chamberlain, L. H., and Shipston, M. J. (2015). The physiology of protein S-acylation. Physiol. Rev. 95 (2), 341–376. doi:10.1152/physrev.00032.2014
Chang, T. J., Chen, W. P., Yang, C., Lu, P. H., Liang, Y. C., Su, M. J., et al. (2009). Serine-385 phosphorylation of inwardly rectifying K+ channel subunit (Kir6.2) by AMP-dependent protein kinase plays a key role in rosiglitazone-induced closure of the K(ATP) channel and insulin secretion in rats. Diabetologia 52 (6), 1112–1121. doi:10.1007/s00125-009-1337-4
Cho, J., Horikawa, Y., Enya, M., Takeda, J., Imai, Y., Imai, Y., et al. (2020). L-Arginine prevents cereblon-mediated ubiquitination of glucokinase and stimulates glucose-6-phosphate production in pancreatic beta-cells. Commun. Biol. 3 (1), 497. doi:10.1038/s42003-020-01226-3
Costes, S., Vandewalle, B., Tourrel-Cuzin, C., Broca, C., Linck, N., Bertrand, G., et al. (2009). Degradation of cAMP-responsive element-binding protein by the ubiquitin-proteasome pathway contributes to glucotoxicity in beta-cells and human pancreatic islets. Diabetes 58 (5), 1105–1115. doi:10.2337/db08-0926
Cui, J., Wang, Z., Cheng, Q., Lin, R., Zhang, X. M., Leung, P. S., et al. (2011). Targeted inactivation of kinesin-1 in pancreatic beta-cells in vivo leads to insulin secretory deficiency. Diabetes 60 (1), 320–330. doi:10.2337/db09-1078
Da Silva Xavier, G. (2018). The cells of the islets of langerhans. J. Clin. Med. 7 (3), 54. doi:10.3390/jcm7030054
Dai, X. Q., Kolic, J., Marchi, P., Sipione, S., and Macdonald, P. E. (2009). SUMOylation regulates Kv2.1 and modulates pancreatic beta-cell excitability. J. Cell Sci. 122 (Pt 6), 775–779. doi:10.1242/jcs.036632
Dai, X. Q., Plummer, G., Casimir, M., Kang, Y., Hajmrle, C., Gaisano, H. Y., et al. (2011). SUMOylation regulates insulin exocytosis downstream of secretory granule docking in rodents and humans. Diabetes 60 (3), 838–847. doi:10.2337/db10-0440
Dall'Agnese, A., Platt, J. M., Zheng, M. M., Friesen, M., Dall'Agnese, G., Blaise, A. M., et al. (2022). The dynamic clustering of insulin receptor underlies its signaling and is disrupted in insulin resistance. Nat. Commun. 13 (1), 7522. doi:10.1038/s41467-022-35176-7
Davey, J. S., Carmichael, R. E., and Craig, T. J. (2019). Protein SUMOylation regulates insulin secretion at multiple stages. Sci. Rep. 9 (1), 2895. doi:10.1038/s41598-019-39681-6
DeFronzo, R. A., Triplitt, C. L., Abdul-Ghani, M., and Cersosimo, E. (2014). Novel agents for the treatment of type 2 diabetes. Diabetes Spectr. 27 (2), 100–112. doi:10.2337/diaspect.27.2.100
Dias, W. B., and Hart, G. W. (2007). O-GlcNAc modification in diabetes and Alzheimer's disease. Mol. Biosyst. 3 (11), 766–772. doi:10.1039/b704905f
Ding, H., Wang, F., Shi, X., Ma, H., Du, Y., Hou, L., et al. (2020). LncRNA MALAT1 induces the dysfunction of beta cells via reducing the histone acetylation of the PDX-1 promoter in type 1 diabetes. Exp. Mol. Pathol. 114, 104432. doi:10.1016/j.yexmp.2020.104432
Dixit, S. S., Wang, T., Manzano, E. J., Yoo, S., Lee, J., Chiang, D. Y., et al. (2013). Effects of CaMKII-mediated phosphorylation of ryanodine receptor type 2 on islet calcium handling, insulin secretion, and glucose tolerance. PLoS One 8 (3), e58655. doi:10.1371/journal.pone.0058655
Dong, G., Adak, S., Spyropoulos, G., Zhang, Q., Feng, C., Yin, L., et al. (2023). Palmitoylation couples insulin hypersecretion with beta cell failure in diabetes. Cell Metab. 35 (2), 332–344.e7. doi:10.1016/j.cmet.2022.12.012
Doser, R. L., Amberg, G. C., and Hoerndli, F. J. (2020). Reactive oxygen species modulate activity-dependent AMPA receptor transport in C. elegans. J. Neurosci. 40 (39), 7405–7420. doi:10.1523/JNEUROSCI.0902-20.2020
Dror, V., Kalynyak, T. B., Bychkivska, Y., Frey, M. H., Tee, M., Jeffrey, K. D., et al. (2008). Glucose and endoplasmic reticulum calcium channels regulate HIF-1beta via presenilin in pancreatic beta-cells. J. Biol. Chem. 283 (15), 9909–9916. doi:10.1074/jbc.M710601200
Durning, S. P., Flanagan-Steet, H., Prasad, N., and Wells, L. (2016). O-linked beta-N-acetylglucosamine (O-GlcNAc) acts as a glucose sensor to epigenetically regulate the insulin gene in pancreatic beta cells. J. Biol. Chem. 291 (5), 2107–2118. doi:10.1074/jbc.M115.693580
Dyachok, O., Idevall-Hagren, O., Sagetorp, J., Tian, G., Wuttke, A., Arrieumerlou, C., et al. (2008). Glucose-induced cyclic AMP oscillations regulate pulsatile insulin secretion. Cell Metab. 8 (1), 26–37. doi:10.1016/j.cmet.2008.06.003
Ferdaoussi, M., Fu, J., Dai, X., Manning Fox, J. E., Suzuki, K., Smith, N., et al. (2017). SUMOylation and calcium control syntaxin-1A and secretagogin sequestration by tomosyn to regulate insulin exocytosis in human ß cells. Sci. Rep. 7 (1), 248. doi:10.1038/s41598-017-00344-z
Flotho, A., and Melchior, F. (2013). Sumoylation: A regulatory protein modification in health and disease. Annu. Rev. Biochem. 82, 357–385. doi:10.1146/annurev-biochem-061909-093311
Gao, B., Kong, Q., Zhang, Y., Yun, C., Dent, S. Y. R., Song, J., et al. (2017). The histone acetyltransferase Gcn5 positively regulates T cell activation. J. Immunol. 198 (10), 3927–3938. doi:10.4049/jimmunol.1600312
Gao, Y., Miyazaki, J., and Hart, G. W. (2003). The transcription factor PDX-1 is post-translationally modified by O-linked N-acetylglucosamine and this modification is correlated with its DNA binding activity and insulin secretion in min6 beta-cells. Arch. Biochem. Biophys. 415 (2), 155–163. doi:10.1016/s0003-9861(03)00234-0
Gao, Z., Reavey-Cantwell, J., Young, R. A., Jegier, P., and Wolf, B. A. (2000). Synaptotagmin III/VII isoforms mediate Ca2+-induced insulin secretion in pancreatic islet beta -cells. J. Biol. Chem. 275 (46), 36079–36085. doi:10.1074/jbc.M004284200
Gareau, J. R., and Lima, C. D. (2010). The SUMO pathway: Emerging mechanisms that shape specificity, conjugation and recognition. Nat. Rev. Mol. Cell Biol. 11 (12), 861–871. doi:10.1038/nrm3011
Gauthier, B. R., Duhamel, D. L., Iezzi, M., Theander, S., Saltel, F., Fukuda, M., et al. (2008). Synaptotagmin VII splice variants alpha, beta, and delta are expressed in pancreatic beta-cells and regulate insulin exocytosis. FASEB J. 22 (1), 194–206. doi:10.1096/fj.07-8333com
Gonelle-Gispert, C., Molinete, M., Halban, P. A., and Sadoul, K. (2000). Membrane localization and biological activity of SNAP-25 cysteine mutants in insulin-secreting cells. J. Cell Sci. 113 (Pt 18), 3197–3205. doi:10.1242/jcs.113.18.3197
Guan, K. L., and Xiong, Y. (2011). Regulation of intermediary metabolism by protein acetylation. Trends Biochem. Sci. 36 (2), 108–116. doi:10.1016/j.tibs.2010.09.003
Haigis, M. C., Mostoslavsky, R., Haigis, K. M., Fahie, K., Christodoulou, D. C., Murphy, A. J., et al. (2006). SIRT4 inhibits glutamate dehydrogenase and opposes the effects of calorie restriction in pancreatic beta cells. Cell 126 (5), 941–954. doi:10.1016/j.cell.2006.06.057
Hanover, J. A., Lai, Z., Lee, G., Lubas, W. A., and Sato, S. M. (1999). Elevated O-linked N-acetylglucosamine metabolism in pancreatic beta-cells. Arch. Biochem. Biophys. 362 (1), 38–45. doi:10.1006/abbi.1998.1016
Hart, G. W., and Akimoto, Y. (2009). “The O-GlcNAc modification,” in Essentials of glycobiology. Editors A. Varki, R. D. Cummings, J. D. Esko, H. H. Freeze, P. Stanley, C. R. Bertozziet al. 2nd ed (New York, NY: Cold Spring Harbor). (NY)).
He, X., Lai, Q., Chen, C., Li, N., Sun, F., Huang, W., et al. (2018). Both conditional ablation and overexpression of E2 SUMO-conjugating enzyme (UBC9) in mouse pancreatic beta cells result in impaired beta cell function. Diabetologia 61 (4), 881–895. doi:10.1007/s00125-017-4523-9
Henquin, J. C. (2011). The dual control of insulin secretion by glucose involves triggering and amplifying pathways in beta-cells. Diabetes Res. Clin. Pract. 93 (Suppl. 1), S27–S31. doi:10.1016/S0168-8227(11)70010-9
Ho, K. H., Yang, X., Osipovich, A. B., Cabrera, O., Hayashi, M. L., Magnuson, M. A., et al. (2020). Glucose regulates microtubule disassembly and the dose of insulin secretion via tau phosphorylation. Diabetes 69 (9), 1936–1947. doi:10.2337/db19-1186
Humphrey, S. J., James, D. E., and Mann, M. (2015). Protein phosphorylation: A major switch mechanism for metabolic regulation. Trends Endocrinol. Metab. 26 (12), 676–687. doi:10.1016/j.tem.2015.09.013
Iida, Y., Senda, T., Matsukawa, Y., Onoda, K., Miyazaki, J. I., Sakaguchi, H., et al. (1997). Myosin light-chain phosphorylation controls insulin secretion at a proximal step in the secretory cascade. Am. J. Physiol. 273 (4), E782–E789. doi:10.1152/ajpendo.1997.273.4.E782
Jambal, P., Masterson, S., Nesterova, A., Bouchard, R., Bergman, B., Hutton, J. C., et al. (2003). Cytokine-mediated down-regulation of the transcription factor cAMP-response element-binding protein in pancreatic beta-cells. J. Biol. Chem. 278 (25), 23055–23065. doi:10.1074/jbc.M212450200
Jhala, U. S., Canettieri, G., Screaton, R. A., Kulkarni, R. N., Krajewski, S., Reed, J., et al. (2003). cAMP promotes pancreatic beta-cell survival via CREB-mediated induction of IRS2. Genes Dev. 17 (13), 1575–1580. doi:10.1101/gad.1097103
Johnson, J. D., Kuang, S., Misler, S., and Polonsky, K. S. (2004). Ryanodine receptors in human pancreatic beta cells: Localization and effects on insulin secretion. FASEB J. 18 (7), 878–880. doi:10.1096/fj.03-1280fje
Jones, D., Morgan, C., and Cockcroft, S. (1999). Phospholipase D and membrane traffic. Potential roles in regulated exocytosis, membrane delivery and vesicle budding. Biochim. Biophys. Acta 1439 (2), 229–244. doi:10.1016/s1388-1981(99)00097-9
Kalwat, M. A., and Cobb, M. H. (2017). Mechanisms of the amplifying pathway of insulin secretion in the beta cell. Pharmacol. Ther. 179, 17–30. doi:10.1016/j.pharmthera.2017.05.003
Kaneto, H., and Matsuoka, T. A. (2015). Role of pancreatic transcription factors in maintenance of mature beta-cell function. Int. J. Mol. Sci. 16 (3), 6281–6297. doi:10.3390/ijms16036281
Kawaguchi, M., Minami, K., Nagashima, K., and Seino, S. (2006). Essential role of ubiquitin-proteasome system in normal regulation of insulin secretion. J. Biol. Chem. 281 (19), 13015–13020. doi:10.1074/jbc.M601228200
Kazim, A. S., Storm, P., Zhang, E., and Renstrom, E. (2017). Palmitoylation of Ca2+ channel subunit CaVβ2a induces pancreatic beta-cell toxicity via Ca2+ overload. Biochem. Biophys. Res. Commun. 491 (3), 740–746. doi:10.1016/j.bbrc.2017.07.117
Kebede, M., Ferdaoussi, M., Mancini, A., Alquier, T., Kulkarni, R. N., Walker, M. D., et al. (2012). Glucose activates free fatty acid receptor 1 gene transcription via phosphatidylinositol-3-kinase-dependent O-GlcNAcylation of pancreas-duodenum homeobox-1. Proc. Natl. Acad. Sci. U. S. A. 109 (7), 2376–2381. doi:10.1073/pnas.1114350109
Khan, F. A., Pandupuspitasari, N. S., Huang, C. J., Hao, X., and Zhang, S. (2016). SUMOylation: A link to future therapeutics. Curr. Issues Mol. Biol. 18, 49–56. doi:10.21775/cimb.018.049
Kim-Muller, J. Y., Kim, Y. J., Fan, J., Zhao, S., Banks, A. S., Prentki, M., et al. (2016). FoxO1 deacetylation decreases fatty acid oxidation in beta-cells and sustains insulin secretion in diabetes. J. Biol. Chem. 291 (19), 10162–10172. doi:10.1074/jbc.M115.705608
Kishi, A., Nakamura, T., Nishio, Y., Maegawa, H., and Kashiwagi, A. (2003). Sumoylation of Pdx1 is associated with its nuclear localization and insulin gene activation. Am. J. Physiol. Endocrinol. Metab. 284 (4), E830–E840. doi:10.1152/ajpendo.00390.2002
Knoch, K. P., Bergert, H., Borgonovo, B., Saeger, H. D., Altkruger, A., Verkade, P., et al. (2004). Polypyrimidine tract-binding protein promotes insulin secretory granule biogenesis. Nat. Cell Biol. 6 (3), 207–214. doi:10.1038/ncb1099
Knoch, K. P., Meisterfeld, R., Kersting, S., Bergert, H., Altkruger, A., Wegbrod, C., et al. (2006). cAMP-dependent phosphorylation of PTB1 promotes the expression of insulin secretory granule proteins in beta cells. Cell Metab. 3 (2), 123–134. doi:10.1016/j.cmet.2005.12.008
Komander, D., and Rape, M. (2012). The ubiquitin code. Annu. Rev. Biochem. 81, 203–229. doi:10.1146/annurev-biochem-060310-170328
Kunieda, K., Yamauchi, H., Kawaguchi, M., Ieda, N., and Nakagawa, H. (2018). Development of a fluorescent probe for detection of citrulline based on photo-induced electron transfer. Bioorg Med. Chem. Lett. 28 (5), 969–973. doi:10.1016/j.bmcl.2018.01.026
Lee, H. Y., Jung, H., Jang, I. H., Suh, P. G., and Ryu, S. H. (2008). Cdk5 phosphorylates PLD2 to mediate EGF-dependent insulin secretion. Cell Signal 20 (10), 1787–1794. doi:10.1016/j.cellsig.2008.06.009
Li, M., Guo, D., Isales, C. M., Eizirik, D. L., Atkinson, M., She, J. X., et al. (2005). SUMO wrestling with type 1 diabetes. J. Mol. Med. Berl. 83 (7), 504–513. doi:10.1007/s00109-005-0645-5
Li, M., Shao, F., Qian, Q., Yu, W., Zhang, Z., Chen, B., et al. (2021). A putative long noncoding RNA-encoded micropeptide maintains cellular homeostasis in pancreatic beta cells. Mol. Ther. Nucleic Acids 26, 307–320. doi:10.1016/j.omtn.2021.06.027
Lim, A., Park, S. H., Sohn, J. W., Jeon, J. H., Park, J. H., Song, D. K., et al. (2009). Glucose deprivation regulates KATP channel trafficking via AMP-activated protein kinase in pancreatic beta-cells. Diabetes 58 (12), 2813–2819. doi:10.2337/db09-0600
Liu, G., Park, S. H., Imbesi, M., Nathan, W. J., Zou, X., Zhu, Y., et al. (2017). Loss of NAD-dependent protein deacetylase sirtuin-2 alters mitochondrial protein acetylation and dysregulates mitophagy. Antioxid. Redox Signal 26 (15), 849–863. doi:10.1089/ars.2016.6662
Lizcano, J. M., Goransson, O., Toth, R., Deak, M., Morrice, N. A., Boudeau, J., et al. (2004). LKB1 is a master kinase that activates 13 kinases of the AMPK subfamily, including MARK/PAR-1. EMBO J. 23 (4), 833–843. doi:10.1038/sj.emboj.7600110
Llanos, P., Contreras-Ferrat, A., Barrientos, G., Valencia, M., Mears, D., and Hidalgo, C. (2015). Glucose-dependent insulin secretion in pancreatic beta-cell islets from male rats requires Ca2+ release via ROS-stimulated ryanodine receptors. PLoS One 10 (6), e0129238. doi:10.1371/journal.pone.0129238
Lockridge, A., Jo, S., Gustafson, E., Damberg, N., Mohan, R., Olson, M., et al. (2020). Islet O-GlcNAcylation is required for lipid potentiation of insulin secretion through SERCA2. Cell Rep. 31 (5), 107609. doi:10.1016/j.celrep.2020.107609
Lopez, J. J., Jardin, I., Sanchez-Collado, J., Salido, G. M., Smani, T., and Rosado, J. A. (2020). TRPC channels in the SOCE scenario. Cells 9 (1), 126. doi:10.3390/cells9010126
Lopez-Avalos, M. D., Duvivier-Kali, V. F., Xu, G., Bonner-Weir, S., Sharma, A., and Weir, G. C. (2006). Evidence for a role of the ubiquitin-proteasome pathway in pancreatic islets. Diabetes 55 (5), 1223–1231. doi:10.2337/db05-0450
Luu, L., Dai, F. F., Prentice, K. J., Huang, X., Hardy, A. B., Hansen, J. B., et al. (2013). The loss of Sirt1 in mouse pancreatic beta cells impairs insulin secretion by disrupting glucose sensing. Diabetologia 56 (9), 2010–2020. doi:10.1007/s00125-013-2946-5
MacDonald, P. E., Ha, X. F., Wang, J., Smukler, S. R., Sun, A. M., Gaisano, H. Y., et al. (2001). Members of the Kv1 and Kv2 voltage-dependent K(+) channel families regulate insulin secretion. Mol. Endocrinol. 15 (8), 1423–1435. doi:10.1210/mend.15.8.0685
MacDonald, P. E., Sewing, S., Wang, J., Joseph, J. W., Smukler, S. R., Sakellaropoulos, G., et al. (2002). Inhibition of Kv2.1 voltage-dependent K+ channels in pancreatic beta-cells enhances glucose-dependent insulin secretion. J. Biol. Chem. 277 (47), 44938–44945. doi:10.1074/jbc.M205532200
Maj, M., Hoermann, G., Rasul, S., Base, W., Wagner, L., and Attems, J. (2016). The microtubule-associated protein tau and its relevance for pancreatic beta cells. J. Diabetes Res. 2016, 1964634. doi:10.1155/2016/1964634
Malm, H. A., Mollet, I. G., Berggreen, C., Orho-Melander, M., Esguerra, J. L., Goransson, O., et al. (2016). Transcriptional regulation of the miR-212/miR-132 cluster in insulin-secreting beta-cells by cAMP-regulated transcriptional co-activator 1 and salt-inducible kinases. Mol. Cell Endocrinol. 424, 23–33. doi:10.1016/j.mce.2016.01.010
Morales-Tarre, O., Alonso-Bastida, R., Arcos-Encarnacion, B., Perez-Martinez, L., and Encarnacion-Guevara, S. (2021). Protein lysine acetylation and its role in different human pathologies: A proteomic approach. Expert Rev. Proteomics 18 (11), 949–975. doi:10.1080/14789450.2021.2007766
Mounier, C., and Posner, B. I. (2006). Transcriptional regulation by insulin: From the receptor to the gene. Can. J. Physiol. Pharmacol. 84 (7), 713–724. doi:10.1139/y05-152
Moynihan, K. A., Grimm, A. A., Plueger, M. M., Bernal-Mizrachi, E., Ford, E., Cras-Meneur, C., et al. (2005). Increased dosage of mammalian Sir2 in pancreatic beta cells enhances glucose-stimulated insulin secretion in mice. Cell Metab. 2 (2), 105–117. doi:10.1016/j.cmet.2005.07.001
Nguyen, P. T., Zottig, X., Sebastiao, M., and Bourgault, S. (2017). Role of site-specific asparagine deamidation in islet amyloid polypeptide amyloidogenesis: Key contributions of residues 14 and 21. Biochemistry 56 (29), 3808–3817. doi:10.1021/acs.biochem.7b00209
Nie, J., Lilley, B. N., Pan, Y. A., Faruque, O., Liu, X., Zhang, W., et al. (2013). SAD-A potentiates glucose-stimulated insulin secretion as a mediator of glucagon-like peptide 1 response in pancreatic beta cells. Mol. Cell Biol. 33 (13), 2527–2534. doi:10.1128/MCB.00285-13
Nie, J., Sun, C., Chang, Z. J., Musi, N., and Shi, Y. G. (2018). SAD-A promotes glucose-stimulated insulin secretion through phosphorylation and inhibition of GDIα in male islet β cells. Endocrinology 159 (8), 3036–3047. doi:10.1210/en.2017-03243
Nie, J., Sun, C., Faruque, O., Ye, G., Li, J., Liang, Q., et al. (2012). Synapses of amphids defective (SAD-A) kinase promotes glucose-stimulated insulin secretion through activation of p21-activated kinase (PAK1) in pancreatic beta-Cells. J. Biol. Chem. 287 (31), 26435–26444. doi:10.1074/jbc.M112.378372
Niki, I., Okazaki, K., Saitoh, M., Niki, A., Niki, H., Tamagawa, T., et al. (1993). Presence and possible involvement of Ca/calmodulin-dependent protein kinases in insulin release from the rat pancreatic beta cell. Biochem. Biophys. Res. Commun. 191 (1), 255–261. doi:10.1006/bbrc.1993.1210
Nolan, C. J., Madiraju, M. S., Delghingaro-Augusto, V., Peyot, M. L., and Prentki, M. (2006). Fatty acid signaling in the beta-cell and insulin secretion. Diabetes 55 (Suppl. 2), S16–S23. doi:10.2337/db06-s003
Oetjen, E., Diedrich, T., Eggers, A., Eckert, B., and Knepel, W. (1994). Distinct properties of the cAMP-responsive element of the rat insulin I gene. J. Biol. Chem. 269 (43), 27036–27044. doi:10.1016/s0021-9258(18)47122-0
Omar-Hmeadi, M., and Idevall-Hagren, O. (2021). Insulin granule biogenesis and exocytosis. Cell Mol. Life Sci. 78 (5), 1957–1970. doi:10.1007/s00018-020-03688-4
Ozcan, S., Andrali, S. S., and Cantrell, J. E. (2010). Modulation of transcription factor function by O-GlcNAc modification. Biochim. Biophys. Acta 1799 (5-6), 353–364. doi:10.1016/j.bbagrm.2010.02.005
Penn, E. J., Brocklehurst, K. W., Sopwith, A. M., Hales, C. N., and Hutton, J. C. (1982). Ca2+--Calmodulin dependent myosin light-chain phosphorylating activity in insulin-secreting tissues. FEBS Lett. 139 (1), 4–8. doi:10.1016/0014-5793(82)80474-2
Peterson, S. B., and Hart, G. W. (2016). New insights: A role for O-GlcNAcylation in diabetic complications. Crit. Rev. Biochem. Mol. Biol. 51 (3), 150–161. doi:10.3109/10409238.2015.1135102
Prakriya, M., and Lewis, R. S. (2015). Store-operated calcium channels. Physiol. Rev. 95 (4), 1383–1436. doi:10.1152/physrev.00020.2014
Price, S. R., Bailey, J. L., Wang, X., Jurkovitz, C., England, B. K., Ding, X., et al. (1996). Muscle wasting in insulinopenic rats results from activation of the ATP-dependent, ubiquitin-proteasome proteolytic pathway by a mechanism including gene transcription. J. Clin. Invest. 98 (8), 1703–1708. doi:10.1172/JCI118968
Rajan, S., Torres, J., Thompson, M. S., and Philipson, L. H. (2012). SUMO downregulates GLP-1-stimulated cAMP generation and insulin secretion. Am. J. Physiol. Endocrinol. Metab. 302 (6), E714–E723. doi:10.1152/ajpendo.00486.2011
Roder, P. V., Wu, B., Liu, Y., and Han, W. (2016). Pancreatic regulation of glucose homeostasis. Exp. Mol. Med. 48 (3), e219. doi:10.1038/emm.2016.6
Sabourin, J., Le Gal, L., Saurwein, L., Haefliger, J. A., Raddatz, E., and Allagnat, F. (2015). Store-operated Ca2+ entry mediated by Orai1 and TRPC1 participates to insulin secretion in rat beta-cells. J. Biol. Chem. 290 (51), 30530–30539. doi:10.1074/jbc.M115.682583
Sacco, F., Humphrey, S. J., Cox, J., Mischnik, M., Schulte, A., Klabunde, T., et al. (2016). Glucose-regulated and drug-perturbed phosphoproteome reveals molecular mechanisms controlling insulin secretion. Nat. Commun. 7, 13250. doi:10.1038/ncomms13250
Sakamaki, J., Fu, A., Reeks, C., Baird, S., Depatie, C., Al Azzabi, M., et al. (2014). Role of the SIK2-p35-PJA2 complex in pancreatic beta-cell functional compensation. Nat. Cell Biol. 16 (3), 234–244. doi:10.1038/ncb2919
Sampley, M. L., and Ozcan, S. (2012). Regulation of insulin gene transcription by multiple histone acetyltransferases. DNA Cell Biol. 31 (1), 8–14. doi:10.1089/dna.2011.1336
Sarkar, S. A., Gunter, J., Bouchard, R., Reusch, J. E., Wiseman, A., Gill, R. G., et al. (2007). Dominant negative mutant forms of the cAMP response element binding protein induce apoptosis and decrease the anti-apoptotic action of growth factors in human islets. Diabetologia 50 (8), 1649–1659. doi:10.1007/s00125-007-0707-z
Schwartz, M. W., Seeley, R. J., Tschop, M. H., Woods, S. C., Morton, G. J., Myers, M. G., et al. (2013). Cooperation between brain and islet in glucose homeostasis and diabetes. Nature 503 (7474), 59–66. doi:10.1038/nature12709
Seino, S. (1999). ATP-Sensitive potassium channels: A model of heteromultimeric potassium channel/receptor assemblies. Annu. Rev. Physiol. 61, 337–362. doi:10.1146/annurev.physiol.61.1.337
Shao, C., and Cobb, M. H. (2009). Sumoylation regulates the transcriptional activity of MafA in pancreatic beta cells. J. Biol. Chem. 284 (5), 3117–3124. doi:10.1074/jbc.M806286200
Shin, S., Le Lay, J., Everett, L. J., Gupta, R., Rafiq, K., and Kaestner, K. H. (2014). CREB mediates the insulinotropic and anti-apoptotic effects of GLP-1 signaling in adult mouse beta-cells. Mol. Metab. 3 (8), 803–812. doi:10.1016/j.molmet.2014.08.001
Sireesh, D., Bhakkiyalakshmi, E., Ramkumar, K. M., Rathinakumar, S., Jennifer, P. S., Rajaguru, P., et al. (2014). Targeting SUMOylation cascade for diabetes management. Curr. Drug Targets 15 (12), 1094–1106. doi:10.2174/1389450115666140915124747
Soesanto, Y., Luo, B., Parker, G., Jones, D., Cooksey, R. C., and McClain, D. A. (2011). Pleiotropic and age-dependent effects of decreased protein modification by O-linked N-acetylglucosamine on pancreatic beta-cell function and vascularization. J. Biol. Chem. 286 (29), 26118–26126. doi:10.1074/jbc.M111.249508
Song, R., Peng, W., Zhang, Y., Lv, F., Wu, H. K., Guo, J., et al. (2013). Central role of E3 ubiquitin ligase MG53 in insulin resistance and metabolic disorders. Nature 494 (7437), 375–379. doi:10.1038/nature11834
Sorrenson, B., Cognard, E., Lee, K. L., Dissanayake, W. C., Fu, Y., Han, W., et al. (2016). A critical role for beta-catenin in modulating levels of insulin secretion from beta-cells by regulating actin cytoskeleton and insulin vesicle localization. J. Biol. Chem. 291 (50), 25888–25900. doi:10.1074/jbc.M116.758516
Sorrenson, B., Dissanayake, W. C., Hu, F., Lee, K. L., and Shepherd, P. R. (2021). A role for PAK1 mediated phosphorylation of beta-catenin Ser552 in the regulation of insulin secretion. Biochem. J. 478 (8), 1605–1615. doi:10.1042/BCJ20200862
Sussel, L., Kalamaras, J., Hartigan-O'Connor, D. J., Meneses, J. J., Pedersen, R. A., Rubenstein, J. L., et al. (1998). Mice lacking the homeodomain transcription factor Nkx2.2 have diabetes due to arrested differentiation of pancreatic beta cells. Development 125 (12), 2213–2221. doi:10.1242/dev.125.12.2213
Sweeney, H. L., and Holzbaur, E. L. F. (2018). Motor proteins. Cold Spring Harb. Perspect. Biol. 10 (5), a021931. doi:10.1101/cshperspect.a021931
Takasawa, S., Kuroki, M., Nata, K., Noguchi, N., Ikeda, T., Yamauchi, A., et al. (2010). A novel ryanodine receptor expressed in pancreatic islets by alternative splicing from type 2 ryanodine receptor gene. Biochem. Biophys. Res. Commun. 397 (2), 140–145. doi:10.1016/j.bbrc.2010.05.051
Tan, J. L., Ravid, S., and Spudich, J. A. (1992). Control of nonmuscle myosins by phosphorylation. Annu. Rev. Biochem. 61, 721–759. doi:10.1146/annurev.bi.61.070192.003445
Thurmond, D. C., Gonelle-Gispert, C., Furukawa, M., Halban, P. A., and Pessin, J. E. (2003). Glucose-stimulated insulin secretion is coupled to the interaction of actin with the t-SNARE (target membrane soluble N-ethylmaleimide-sensitive factor attachment protein receptor protein) complex. Mol. Endocrinol. 17 (4), 732–742. doi:10.1210/me.2002-0333
Tian, G., Sagetorp, J., Xu, Y., Shuai, H., Degerman, E., and Tengholm, A. (2012a). Role of phosphodiesterases in the shaping of sub-plasma-membrane cAMP oscillations and pulsatile insulin secretion. J. Cell Sci. 125 (Pt 21), 5084–5095. doi:10.1242/jcs.107201
Tian, G., Sandler, S., Gylfe, E., and Tengholm, A. (2011). Glucose- and hormone-induced cAMP oscillations in α- and β-cells within intact pancreatic islets. Diabetes 60 (5), 1535–1543. doi:10.2337/db10-1087
Tian, G., Sol, E. R., Xu, Y., Shuai, H., and Tengholm, A. (2015). Impaired cAMP generation contributes to defective glucose-stimulated insulin secretion after long-term exposure to palmitate. Diabetes 64 (3), 904–915. doi:10.2337/db14-1036
Tian, G., Tepikin, A. V., Tengholm, A., and Gylfe, E. (2012b). cAMP induces stromal interaction molecule 1 (STIM1) puncta but neither Orai1 protein clustering nor store-operated Ca2+ entry (SOCE) in islet cells. J. Biol. Chem. 287 (13), 9862–9872. doi:10.1074/jbc.M111.292854
Uruno, A., Furusawa, Y., Yagishita, Y., Fukutomi, T., Muramatsu, H., Negishi, T., et al. (2013). The Keap1-Nrf2 system prevents onset of diabetes mellitus. Mol. Cell Biol. 33 (15), 2996–3010. doi:10.1128/MCB.00225-13
Vaquero, A., Scher, M. B., Lee, D. H., Sutton, A., Cheng, H. L., Alt, F. W., et al. (2006). SirT2 is a histone deacetylase with preference for histone H4 Lys 16 during mitosis. Genes Dev. 20 (10), 1256–1261. doi:10.1101/gad.1412706
Varadi, A., Tsuboi, T., Johnson-Cadwell, L. I., Allan, V. J., and Rutter, G. A. (2003). Kinesin I and cytoplasmic dynein orchestrate glucose-stimulated insulin-containing vesicle movements in clonal MIN6 beta-cells. Biochem. Biophys. Res. Commun. 311 (2), 272–282. doi:10.1016/j.bbrc.2003.09.208
Varadi, A., Tsuboi, T., and Rutter, G. A. (2005). Myosin Va transports dense core secretory vesicles in pancreatic MIN6 beta-cells. Mol. Biol. Cell 16 (6), 2670–2680. doi:10.1091/mbc.e04-11-1001
Vergari, E., Plummer, G., Dai, X., and MacDonald, P. E. (2012). DeSUMOylation controls insulin exocytosis in response to metabolic signals. Biomolecules 2 (2), 269–281. doi:10.3390/biom2020269
Walsh, C. T., Garneau-Tsodikova, S., and Gatto, G. J. (2005). Protein posttranslational modifications: The chemistry of proteome diversifications. Angew. Chem. Int. Ed. Engl. 44 (45), 7342–7372. doi:10.1002/anie.200501023
Walsh, G., and Jefferis, R. (2006). Post-translational modifications in the context of therapeutic proteins. Nat. Biotechnol. 24 (10), 1241–1252. doi:10.1038/nbt1252
Wang, B., Lin, H., Li, X., Lu, W., Kim, J. B., Xu, A., et al. (2020). The adaptor protein APPL2 controls glucose-stimulated insulin secretion via F-actin remodeling in pancreatic beta-cells. Proc. Natl. Acad. Sci. U. S. A. 117 (45), 28307–28315. doi:10.1073/pnas.2016997117
Wei, F. Y., Nagashima, K., Ohshima, T., Saheki, Y., Lu, Y. F., Matsushita, M., et al. (2005). Cdk5-dependent regulation of glucose-stimulated insulin secretion. Nat. Med. 11 (10), 1104–1108. doi:10.1038/nm1299
Wende, A. R. (2015). Unsticking the broken diabetic heart: O-GlcNAcylation and calcium sensitivity. Diabetes 64 (10), 3339–3341. doi:10.2337/dbi15-0001
Wijesekara, N., Goncalves, R. A., Ahrens, R., De Felice, F. G., and Fraser, P. E. (2018). Tau ablation in mice leads to pancreatic beta cell dysfunction and glucose intolerance. FASEB J. 32 (6), 3166–3173. doi:10.1096/fj.201701352
Wilson, J. R., Biden, T. J., and Ludowyke, R. I. (1999). Increases in phosphorylation of the myosin II heavy chain, but not regulatory light chains, correlate with insulin secretion in rat pancreatic islets and RINm5F cells. Diabetes 48 (12), 2383–2389. doi:10.2337/diabetes.48.12.2383
Wu, B., Wei, S., Petersen, N., Ali, Y., Wang, X., Bacaj, T., et al. (2015). Synaptotagmin-7 phosphorylation mediates GLP-1-dependent potentiation of insulin secretion from beta-cells. Proc. Natl. Acad. Sci. U. S. A. 112 (32), 9996–10001. doi:10.1073/pnas.1513004112
Wu, T., Zhang, S., Xu, J., Zhang, Y., Sun, T., Shao, Y., et al. (2020). HRD1, an important player in pancreatic beta-cell failure and therapeutic target for type 2 diabetic mice. Diabetes 69 (5), 940–953. doi:10.2337/db19-1060
Wu, X., Xu, M., Geng, M., Chen, S., Little, P. J., Xu, S., et al. (2023). Targeting protein modifications in metabolic diseases: Molecular mechanisms and targeted therapies. Signal Transduct. Target Ther. 8 (1), 220. doi:10.1038/s41392-023-01439-y
Xiong, X., Wang, G., Tao, R., Wu, P., Kono, T., Li, K., et al. (2016). Sirtuin 6 regulates glucose-stimulated insulin secretion in mouse pancreatic beta cells. Diabetologia 59 (1), 151–160. doi:10.1007/s00125-015-3778-2
Xu, J., Zhang, W., Cui, W., Shi, B., and Wang, H. (2019). PKCα promotes insulin secretion via TRPC1 phosphorylation in INS-1E cells. Biosci. Biotechnol. Biochem. 83 (9), 1676–1682. doi:10.1080/09168451.2019.1617106
Yamada, T., Murata, D., Adachi, Y., Itoh, K., Kameoka, S., Igarashi, A., et al. (2018). Mitochondrial stasis reveals p62-mediated ubiquitination in parkin-independent mitophagy and mitigates nonalcoholic fatty liver disease. Cell Metab. 28 (4), 588–604.e5. doi:10.1016/j.cmet.2018.06.014
Yang, M. L., Horstman, S., Gee, R., Guyer, P., Lam, T. T., Kanyo, J., et al. (2022). Citrullination of glucokinase is linked to autoimmune diabetes. Nat. Commun. 13 (1), 1870. doi:10.1038/s41467-022-29512-0
Yang, M. L., Sodre, F. M. C., Mamula, M. J., and Overbergh, L. (2021). Citrullination and PAD enzyme Biology in type 1 diabetes - regulators of inflammation, autoimmunity, and pathology. Front. Immunol. 12, 678953. doi:10.3389/fimmu.2021.678953
Yang, X. D., Xiang, D. X., and Yang, Y. Y. (2016). Role of E3 ubiquitin ligases in insulin resistance. Diabetes Obes. Metab. 18 (8), 747–754. doi:10.1111/dom.12677
Yang, X., Ongusaha, P. P., Miles, P. D., Havstad, J. C., Zhang, F., So, W. V., et al. (2008). Phosphoinositide signalling links O-GlcNAc transferase to insulin resistance. Nature 451 (7181), 964–969. doi:10.1038/nature06668
Yang, X., and Qian, K. (2017). Protein O-GlcNAcylation: Emerging mechanisms and functions. Nat. Rev. Mol. Cell Biol. 18 (7), 452–465. doi:10.1038/nrm.2017.22
Yao, C., Zhuang, H., Cheng, W., Lin, Y., Du, P., Yang, B., et al. (2015). FADD phosphorylation impaired islet morphology and function. J. Cell Physiol. 230 (7), 1448–1456. doi:10.1002/jcp.24885
Yoshida, M., Yokoi, N., Takahashi, H., Hatano, N., Hayami, T., Ogawa, W., et al. (2022). O-GlcNAcylation of myocyte-specific enhancer factor 2D negatively regulates insulin secretion from pancreatic beta-cells. Biochem. Biophys. Res. Commun. 605, 90–96. doi:10.1016/j.bbrc.2022.03.036
Zhang, C., Moriguchi, T., Kajihara, M., Esaki, R., Harada, A., Shimohata, H., et al. (2005). MafA is a key regulator of glucose-stimulated insulin secretion. Mol. Cell Biol. 25 (12), 4969–4976. doi:10.1128/MCB.25.12.4969-4976.2005
Zhang, W., Lilja, L., Mandic, S. A., Gromada, J., Smidt, K., Janson, J., et al. (2006). Tomosyn is expressed in beta-cells and negatively regulates insulin exocytosis. Diabetes 55 (3), 574–581. doi:10.2337/diabetes.55.03.06.db05-0015
Zhang, Y., Zhou, F., Bai, M., Liu, Y., Zhang, L., Zhu, Q., et al. (2019). The pivotal role of protein acetylation in linking glucose and fatty acid metabolism to beta-cell function. Cell Death Dis. 10 (2), 66. doi:10.1038/s41419-019-1349-z
Zhou, G., Liu, S. H., Shahi, K. M., Wang, H., Duan, X., Lin, X., et al. (2012). Negative regulation of pancreatic and duodenal homeobox-1 by somatostatin receptor subtype 5. Mol. Endocrinol. 26 (7), 1225–1234. doi:10.1210/me.2012-1095
Keywords: PTMs, phosphorylation, acetylation, ubiquitination, sumoylation, O-GlcNAcylation, palmitoylation, insulin secretion
Citation: Yang C, Wei M, Zhao Y, Yang Z, Song M, Mi J, Yang X and Tian G (2023) Regulation of insulin secretion by the post-translational modifications. Front. Cell Dev. Biol. 11:1217189. doi: 10.3389/fcell.2023.1217189
Received: 05 May 2023; Accepted: 24 July 2023;
Published: 04 August 2023.
Edited by:
Venkateswarlu Kanamarlapudi, Swansea University Medical School, United KingdomReviewed by:
Hui-Ying Lim, University of Oklahoma Health Sciences Center, United StatesRohit Mahar, Hemwati Nandan Bahuguna Garhwal University, India
Suresh Kumar Mohankumar, Swansea University Medical School, United Kingdom
Copyright © 2023 Yang, Wei, Zhao, Yang, Song, Mi, Yang and Tian. This is an open-access article distributed under the terms of the Creative Commons Attribution License (CC BY). The use, distribution or reproduction in other forums is permitted, provided the original author(s) and the copyright owner(s) are credited and that the original publication in this journal is cited, in accordance with accepted academic practice. No use, distribution or reproduction is permitted which does not comply with these terms.
*Correspondence: Geng Tian, dGlhbmdlbmdAYnptYy5lZHUuY24=; Xiaoyong Yang, eGlhb3lvbmcueWFuZ0B5YWxlLmVkdQ==
†These authors have contributed equally to this work and share first authorship