- 1Anhui Province Key Laboratory of Local Livestock and Poultry Genetic Resource Conservation and Bio-breeding, Anhui Agricultural University, Hefei, China
- 2College of Animal Science and Technology, Anhui Agricultural University, Hefei, China
Telomerase determines cell lifespan by controlling chromosome stability and cell viability, m6A epigenetic modification plays an important role in the regulation of telomerase activity. Using CRISPR epigenome editing to analyze specific m6A modification sites in telomerase will provide an important tool for analyzing the molecular mechanism of m6A modification regulating telomerase activity. In this review, we clarified the relevant applications of CRISPR system, paid special attention to the regulation of m6A modification in stem cells and cancer cells based on CRISPR system, emphasized the regulation of m6A modification on telomerase activity, pointed out that m6A modification sites regulate telomerase activity, and discussed strategies based on telomerase activity and disease treatment, which are helpful to promote the research of anti-aging and tumor related diseases.
1 Introduction
The research of telomere and telomerase is of great significance to the aging of organism. Telomerase is an eukaryotic ribonucleoprotein (RNP) composed of RNA-protein complex (Blackburn and Collins, 2011). It extends the 3‘end of linear chromosome by synthesizing the telomere repeat TTAGGG to maintain telomere length and chromosome stability (Liu et al., 2022). Telomerase activity is closely related to tumorigenesis (Shay, 2016; Trybek et al., 2020), cell proliferation and cell aging (Chakravarti et al., 2021). N6-methyladenosine (m6A) RNA modification is an important epigenetic modification mode in post-transcriptional regulation (Schmidt et al., 1975; Roundtree et al., 2017a; Tang et al., 2020), which involves almost all aspects of RNA metabolism and affects various physiological and pathological processes by regulating mRNA cytoplasmic transport, splicing, stability, structure and translation (Dominissini et al., 2012). The composition of telomerase fully shows that it has a close relationship with m6A modification. The study of the mechanism of m6A modification regulating telomerase activity and maintaining telomere length will promote human anti-aging to provide new ideas.
With the application and improvement of CRISPR (clustered regularly interspaced short palindromic repeats)/Cas system (Jinek et al., 2012; Cong et al., 2013), the modified fusion protein dm6ACRISPR system can achieve precise and efficient m6A site-specific modification in RNA transcripts (Liu et al., 2019; Wilson et al., 2020), this will help to further explore the mechanism of m6A modification. In clinical cancer research, it was found that there was a site mutation in the promoter region of telomerase reverse transcriptase (TERT) gene. Use the base editing function of CRISPR system to reduce the transcription and protein expression of TERT, and induce the aging and proliferation stagnation of cancer cells, which verifies the feasibility of activated TERT promoter mutation as a cancer-specific therapeutic target (Killela et al., 2013; Li et al., 2020). Therefore, the CRISPR system technology, combined with the m6A modification of RNA and the regulation of telomerase activity, is used to regulate the aging and proliferation of cells in the body and achieve the treatment of various diseases.
In this review, we reviewed and discussed the latest research progress, and found that the CRISPR system was used to carry out m6A site-specific modification of RNA, regulate telomerase activity and affect telomere length by regulating telomerase assembly and other processes, which provided a direction for the study of epigenetic modification to regulate cell aging mechanism, and provided a prospect for the future research on cell proliferation and aging.
2 CRISPR system introduction
CRISPR/Cas9 system widely exists in prokaryotes and provides acquired immunity against the invasion of foreign viruses and plasmids (Ghaemi et al., 2021). The developed CRISPR/Cas system can accurately edit DNA or RNA targets at specific sites and has been widely used in gene editing (Hsu et al., 2014; Manghwar et al., 2019; Zhang et al., 2021). According to the composition of Cas effector proteins, CRISPR system is divided into Class I and Class II (Figure 1). These systems use site-specific guide RNA to guide Cas protein and accurately edit site-specific sequences (Wang et al., 2022; Yu et al., 2022). At present, the most widely used CRISPR systems are Class II Type II Cas9, Type V Cas12 and Type VI Cas13 (Figure 1).
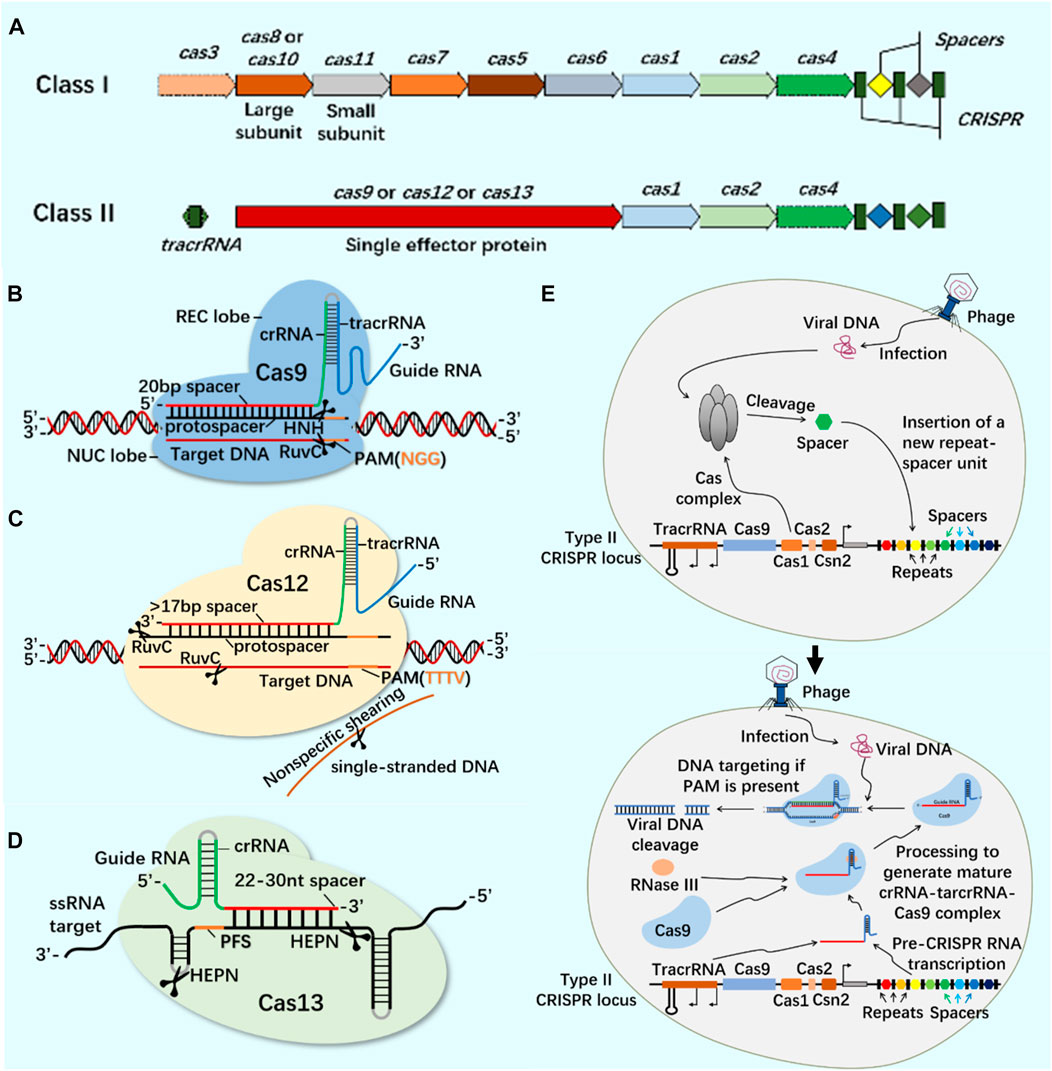
FIGURE 1. CRISPR/Cas system classification, typical structure, important members of Class II CRISPR/Cas system and principle of CRISPR/Cas system. (A) The structure of class I and class II CRISPR/Cas system loci. The class I of CRISPR/Cas system is composed of multiple Cas proteins to form a crRNA complex, which plays a role in the binding and processing of targets; The class II of CRISPR/Cas system is composed of a single multi-domain crRNA-binding protein, and its function is similar to the effect complex in the class I of CRISPR system. (B) CRISPR/Cas9 system is mainly composed of Cas9 protein and single-stranded guide RNA (sgRNA). Cas9 protein has the function of cutting DNA double-stranded, and sgRNA plays a guiding role. In the presence of the adjacent motif (PAM) of the prototype spacer, Cas9 protein can reach different target sites through base complementary pairing under the guidance of sgRNA, and achieve DNA double strand break (DSB) by cutting the target gene through the two nuclease domains of RuvC and HNH. (C) CRISPR/Cas12 system is mainly composed of Cas12 protein and single-stranded guide RNA (sgRNA). Cas12 protein has side-cutting activity and can cut double and single strands of DNA. It mainly plays the role of inducing DSBs through a single RuvC-like nuclease domain. (D) CRISPR/Cas13 system is mainly composed of Cas13 protein and single-stranded guide RNA (sgRNA). Cas13 protein is a single protein composed of multiple domains. It has the function of recognizing crRNA, cutting RNA, and even cutting pre-crRNA. It has the phenomenon of side-cutting activity similar to CRISPR/Cas12 system. It cuts RNA through two HEPN domains. (E) CRISPR/Cas system is an acquired immune system from bacteria and archaea. Taking the principle of CRISPR/Cas9 system as an example: the acquisition of highly variable spacer of CRISPR - the expression of CRISPR loci (transcription and post-transcriptional maturation) - the development of CRISPR/Cas9 system activity.
The CRISPR system is mainly used to modify specific target genes in the genome of organisms. The main editors include DNA cytosine base editor (CBE), adenine base editor (ABE) and primer editor (PE) (Kantor et al., 2020). PE is a multi-functional and high-precision genome editor (Anzalone et al., 2019), which is composed of two parts: the leader editor protein and primer editing guided RNA (pegRNA). Using the CRISPR Cas protein targeted DNA to make nicks and the DNA synthesis ability of reverse transcriptase, the sequence encoded by pegRNA can be accurately and efficiently copied into the targeted DNA sequence to achieve accurate editing, including replacement, insertion and deletion (Anzalone et al., 2019; Kantor et al., 2020; Kim et al., 2020). The advantage of PE is that it does not cause DNA double strand breakage, only cutting one strand of DNA, thereby avoiding potential risks such as chromosome loss and rearrangement caused by double strand DNA breakage. Researchers can further improve the accuracy and specificity of PE by optimizing lead editing proteins, pegRNA, and AAV genomic elements, such as introducing engineered Cas9 mutants, especially eSpCas9 and Sniper Cas9 mutants, into PE (Kim et al., 2020). The PE editing efficiency prediction models DeepPrime, DeepPrime FT, and the off target prediction model DeepPrime Off make the design and screening of pegRNAs more convenient and efficient, providing strong guarantees for the future widespread application of PE systems (Yu et al., 2023). Using PE to repair sickle cell anemia (SCD) mutations in hematopoietic stem cells or progenitor cells of patients, the repaired cells are treated for hereditary blood diseases through transplantation (Everette et al., 2023). The gene editing system developed based on CRISPR technology has brought prospects for the research and treatment of genetic diseases.
3 CRISPR system and RNA editing
At present, CRISPR/Cas9 and CRISPR/Cas13 systems have become tools for the research and application of DNA and RNA epigenetic modification (Figure 2; Figure 3) (Zhan et al., 2019; Kordyś et al., 2022). Nucleic acid endonuclease deficient Cas9 (dCas9)/Cas13 (dCas13) still has the activity of binding enzyme, which can combine with effector protein to regulate the expression of DNA or RNA, becoming an effective method to study gene function and regulation mechanism (Yang et al., 2019; Liu et al., 2020).
Working principle of CRISPR/dCas9 system and related tools based on CRISPR/dCas9 system development (gene editing, live cell imaging, base editing, methylation modification, histone modification and transcription regulation). Ac, acetylation; Me, Methylation; Dme, Demethylation.
3.1 RNA editing tools: based on CRISPR/dCas13 system
The modification of CRISPR system for gene editing at the DNA level is irreversible, especially the ethical issues involved in the safety of human germ cell and embryonic cell editing cannot be ignored (Leibowitz et al., 2021; Höijer et al., 2022). p53 gene is a tumor suppressor gene that participates in the regulation of cell growth, differentiation, apoptosis and other processes (Fischer et al., 2016). After Cas9 protein was introduced into cells to realize CRISPR/Cas9 mediated genome editing, p53 pathway was upregulated and DNA repair level was increased. Cas9 protein induces p53 pathway activation and p53 mediated DNA damage response (Enache et al., 2020). These findings are of great significance for the correct application of CRISPR/Cas9 mediated genome editing (Enache et al., 2020; Sinha et al., 2021). Therefore, the use of CRISPR/Cas9 technology in human pluripotent stem cells (hPSCs) cell replacement therapy should be carefully carried out and the p53 function of hPSCs cells should be monitored (Ihry et al., 2018). The modification of Cas13 protein at the RNA level successfully avoids irreversible permanent changes to the genome, and is an important tool for studying the most abundant m6A modification on RNA. At the same time, it plays an important role in studying the structure and function of telomerase composed of RNA and protein.
In terms of RNA editing, the CRISPR system has been deeply modified and applied to mRNA epigenetic modification research. The m1A modification detection method based on the CRISPR/Cas13a system has been successfully used to identify m1A in 28S rRNA (Chen et al., 2019). The catalytic inactivation of RfxCas13d (dCasRx) is fused with the m1A demethylase ALKBH3, and the dCasRx ALKBH3 fusion protein can mediate effective demethylation of m1A modified RNA, known as Reengined m1A modification valid eraser (“REVER”), providing a tool for further elucidating the relationship between m1A modification of specific transcripts and their phenotypic results (Xie et al., 2021). m1A regulates the level of glycolysis in tumor cells by regulating the expression of ATP5D in the mitochondrial ATP synthase F1 domain. The dm1ACRISPR system can upregulate the expression of ATP5D through targeted removal of ATP5D m1A modification, resulting in an increase in the level of glycolysis of tumor cells (Wu et al., 2022). This is similar to using the CRISPR system to study m6A modification, where endogenous editing studies can be conducted by identifying the targets of epigenetic modifications on mRNA such as m1A and m5C. Because the CRISPR/RfxCas13d (CasRx) related transcriptome epigenetic modification editor has the characteristics of small size and high editing efficiency (Konermann et al., 2018; Zhang et al., 2018), which is suitable for packaging into lentivirus vector for gene function research. At present, CasRx has been successfully used to knock down specific mRNA transcripts in zebrafish embryos (Kushawah et al., 2020), and to mediate RNA targeted treatment of age-related macular degeneration in model mice (Zhou et al., 2020).
4 CRISPR system and m6A modification
As an important biological function of RNA modification, m6A modification widely exists in almost all types of RNA molecules in cells (Yang et al., 2018; Hu et al., 2022). In the regulation of m6A modification, combining the modified protein specific domain with the inactivated CRISPR protein can produce a new precise editing tool for RNA methylation modification (Figure 4) (Li et al., 2020; Wilson et al., 2020; Kordyś et al., 2022). Liu et al. designed m6A modified eraser by combining CRISPR/Cas9 with demethylase ALKBH5 or FTO to realize RNA site-specific demethylation (Liu et al., 2019). Considering the important regulatory role of m6A modification on RNA in the nucleus, based on the RNA-targeted endonuclease system CRISPR/Cas13, an editor for targeted RNA methylation (TRM) was constructed, which became a new accurate editing tool for m6A modification. The editor can achieve efficient and accurate editing of m6A modification of RNA in nucleus and cytoplasm through nuclear export-signal (NES) and nuclear localization signal (NLS) (Wilson et al., 2020). The dm6ACRISPR editing tool can realize m6A modification of RNA sites, providing a more powerful weapon for in-depth research on the function of m6A modification (Table 1).
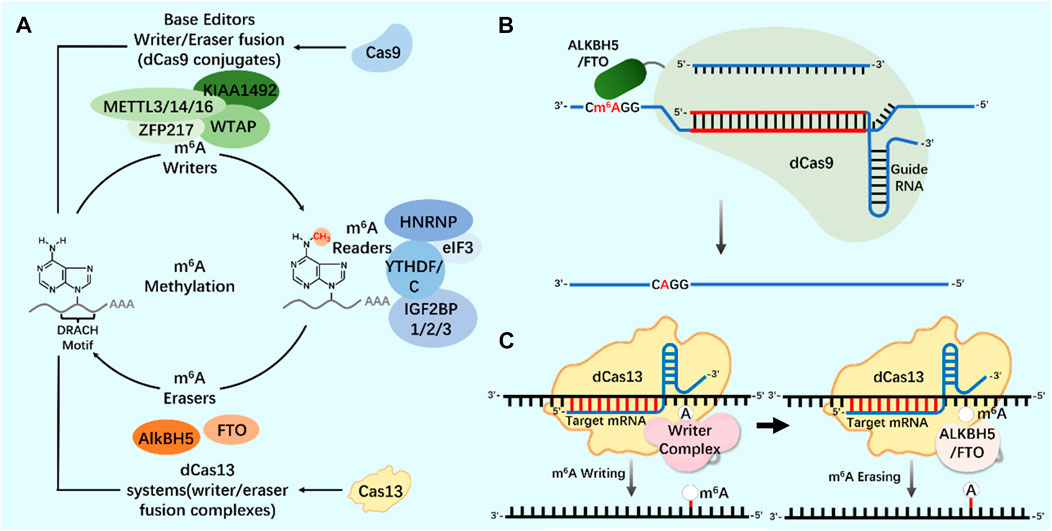
FIGURE 4. CRISPR system and m6A modification (A) N6-methyladenosine (m6A) regulation mechanism. Writers and erasers strictly regulate the presence of m6A on transcripts by targeting the m6A motif (DRACH). m6A is recognized by readers and starts the steps of regulating mRNA stability and translation. The modification system can be extended to include Cas9 (base editor, writer/eraser fusion) and Cas13 (methylation system). (B) Application of CRISPR/dCas9-ALKBH5/FTO tool in m6A modification. (C) Application of CRISPR/dCas13-Writer Complex tool in m6A modification; Application of CRISPR/dCas13-ALKBH5/FTO tool in m6A modification.
5 CRISPR system and telomerase
Telomerase, as an enzymatic RNP complex, plays a role of reverse transcriptase in the process of telomere elongation, and is significantly associated with cell aging and tumorigenesis (Sun et al., 2019). In cancer cells (Bajaj et al., 2020; Negrini et al., 2020; Wu et al., 2020), hematopoietic stem cells (Celtikci et al., 2021) and germ cells (Dogan and Forsyth, 2021; Lupatov and Yarygin, 2022), telomerase showed high activity (Demanelis et al., 2020). Cancer is closely related to a series of changes in intracellular genome and epigenome (Ushijima et al., 2021). Telomerase is silent in most normal somatic cells, but activated in 90% of cancer cells, making it an excellent target for cancer treatment. In the treatment of cancer, all kinds of telomerase activity inhibitors have failed due to their side effects. Coats plus (CP) is a rare autosomal recessive disease caused by CTC1 mutation, which is important for maintaining telomere length. CTC1L1142H mutation caused telomere damage. The point mutation of CTC1 using CRISPR/Cas9 technology confirmed that the interaction between CTC1 and STN1 is necessary to inhibit telomerase activity (Gu et al., 2018). Combining the biological functions of CRISPR/Cas9 and telomerase, the development of telomerase activating gene expression (Tage) has gradually become a new cancer gene therapy method. The Tage system consists of three components: the effector gene expression vector carrying 3‘telomerase recognition rod end, the dCas9-VP64 expression vector and the sgRNA artificial transcription factor expression vector targeting the telomere repeat sequence. Using AAV as a gene vector, the Tage system can effectively kill cancer cells and safely realize its application in the body (Dai et al., 2019). In cancer research using CRISPR system, CRISPR activation screening of targeted gRNA was carried out, gRNA libraries targeting different genes were established, targeted genes in cancer cells were systematically and accurately knocked out, and cancer gene therapy was achieved (Joung et al., 2022; Katti et al., 2022; Ye et al., 2022).
Telomerase activity usually depends on the expression level of TERT, which is the catalytic subunit of RNP complex (Barthel et al., 2017; Wu et al., 2021). The recruitment of telomerase to telomere occurs in the S phase of the cell cycle. By using CRISPR genome editing system and CRISPR-aided nano microscope technology to track telomerase in the nucleus, it is proved that telomerase uses three-dimensional diffusion to search for telomeres, and the recruitment of telomerase to telomere is driven by the dynamic interaction between the rapidly diffusing telomerase protein TERT and telomere protein TPP1 (Schmidt et al., 2016). In the study of human telomerase RNA (hTR) biogenic post-transcriptional modification, the use of CRISPR system consumes trimethyl guanosine synthetase 1 (TGS1). The reduction of trimethylation will increase the coupling of hTR with cap-binding complex (CBC) and Sec1/Munc-18 (Sm) chaperone protein, The accumulation of mature hTR in the nucleus and cytoplasm increases, and the increased hTR is assembled with TERT protein to produce increased active telomerase complex and increased telomerase activity, thus realizing the telomere elongation of cultured human cells. This study provides a new treatment scheme for telomerase dysfunction in telomeric syndrome (Figure 5) (Chen et al., 2020).
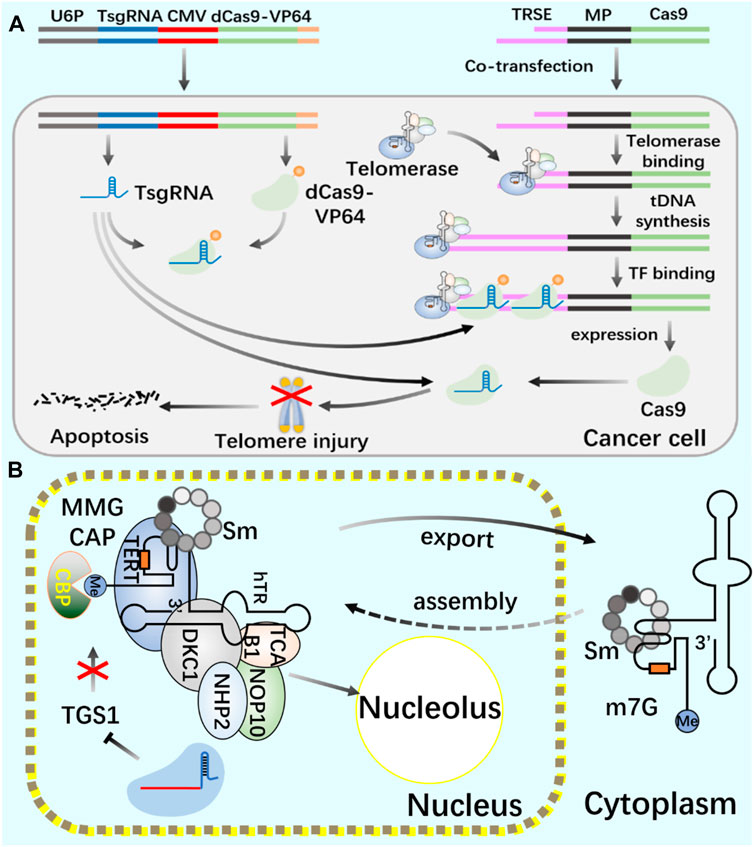
FIGURE 5. Application of CRISPR/Cas9 system in regulating telomerase and telomere (A) Use CRISPR/Cas9 and CRISPR/dCas9 systems to cut telomeres through telomerase to produce DNA damage and induce cancer cell death. (B) Use CRISPR/Cas9 system to introduce TGS1 gene frameshift mutation to realize the deletion of TGS1 hypermethylation enzyme and promote the increase of telomerase RNA and telomere elongation.
In order to further study the activation of telomerase and its activity regulation mechanism, in view of the low editing efficiency of CRISPR/Cas9 at the TERT gene locus, the genome editing method of “pop in/pop out” is used to realize precise modification of endogenous TERT gene sites in cells. This method provides a powerful tool for studying the biological function of telomerase using CRISPR/Cas9 (Kühn and Chu, 2015; Xi et al., 2015). Thus, the emergence of CRISPR system will provide an important tool for human research on telomerase and the regulation mechanism of cell aging.
6 dm6ACRISPR system and telomerase
As a repeat DNA sequence at the end of chromosome, telomere shortening is considered as a biological marker of cell aging (Al-Turki and Griffith, 2023). At each cell division, 50–100 pairs of base pairs will be lost in the chromosome end sequence, resulting in cell aging and even death (Blasco, 2005; Rossiello et al., 2022). Telomerase contains specialized TERT and telomerase RNA (TER), and has its own template and catalytic core required by TERT (Cash and Feigon, 2017; Jiang et al., 2018; Wang et al., 2019). In most human cancers, the increase of telomerase level makes cancer cells have the ability to proliferate indefinitely (Roake and Artandi, 2020). According to the characteristics of telomerase structure, composition and epigenetic modification (Figure 6; Figure 7), the telomere repeat sequence at the end of chromosome is extended to maintain the stability of genome, and the gradual loss of telomere caused by genome replication is offset. These are important for studying cell proliferation and delaying cell aging (He et al., 2021; Liu et al., 2022; Sekne et al., 2022).
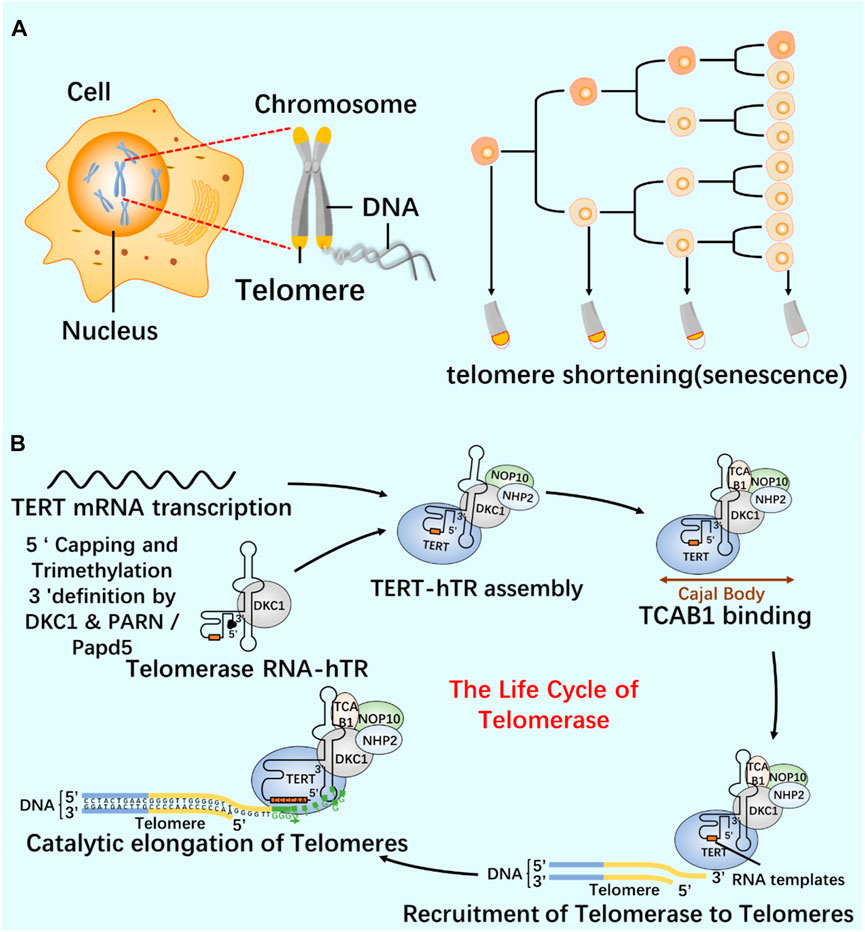
FIGURE 7. Relationship between telomeres and telomerase (A) Cell proliferation and telomere length reduction. Telomere is a repetitive DNA structure at the top of the chromosome. When the cell division DNA replicates, the telomere will protect the integrity of the chromosome. The activity of telomerase in normal cells was inhibited, and the telomere gradually shortened and disappeared with the continuous cell division. Chromosomes are finally completely exposed, cells cannot proliferate, DNA molecules degrade, and life ends. (B) The life cycle of telomerase and its regulation mechanism. Telomerase-protein RNA complex uses the non-coding RNA subunit hTR as a template, and the reverse transcriptase TERT catalyzes the telomere elongation. The life cycle of telomerase includes post-transcriptional modification (PTM) and maturation of hTR, intracellular localization, and effective assembly with TERT until the formation of a whole enzyme that can prolong telomeres.
The abnormal modification of RNA methylation is closely related to a series of cancer occurrence, and studying the relationship between m6A modification and tumor occurrence is of great significance for the treatment of cancer. In liver cancer research, it was found that methyltransferase METTL14 has a dual effect of promoting cancer cell proliferation and differentiation and inhibiting cancer cell metastasis (Ma et al., 2017; Chen et al., 2018); Overexpression of METTL5 promotes the growth, proliferation, migration, and invasion of liver cancer, knockdown of METTL5 promotes cell apoptosis, and inhibits the growth, proliferation, migration, and invasion of liver cancer (Peng et al., 2022). METTL3 has carcinogenic function in human liver cancer, and downregulation of METTL3 can weaken the tumorigenicity and lung metastasis of liver cancer (Chen and Wong, 2020). In glioblastoma, METTL3 can promote the maintenance and radiation resistance of glioblastoma stem cells and inhibit their self-renewal and proliferation (Cui et al., 2017; Visvanathan et al., 2018). Inhibition of FTO expression can hinder the growth, differentiation and self-renewal of glioblastoma stem cells (Cui et al., 2017). ALKBH5 can promote stem cell self-renewal and proliferation (Zhang et al., 2017). Overexpression of ALKBH5 was found in breast cancer research to enhance the enrichment of breast cancer stem cells (BCSC) (Zhang et al., 2016). In lung cancer and bladder cancer, METTL3 knockout can reduce the growth, survival and invasiveness of lung cancer cells, as well as the proliferation, invasion, in vitro survival and in vivo tumorigenicity of bladder cancer cells (Lin et al., 2016; Han et al., 2019). The m6A modification is closely related to the occurrence of cancer, and the m6A editing tool based on the CRISPR system will help to analyze the correlation mechanism between m6A modification and cancer occurrence.
At present, the dm6ACRISPR editing tool is constructed by combining the catalytically inactivated Cas protein with the m6A modification related protein (Li et al., 2020). This laid a foundation for studying the relationship between epigenetic modification and telomerase function and exploring the mechanism of m6A modification on telomerase activity regulation.
Telomerase structure. Telomerase is a ribonucleoprotein complex, which is composed of scaffold non-coding human telomerase RNA (hTR), telomerase reverse transcriptase (TERT) and related cofactors. Telomerase is composed of two RNA-linked structures. One is the H/ACA domain of hTR, which is composed of two groups of dyskerin complex (dyskerin, NHP2, NOP10 and GAR1) and TCAB1. The other contains the catalytic core, where hTR and TERT surround the telomere substrate. The two are connected through the CR4/5 domain of hTR.
6.1 Regulation of telomerase activity by m6A modification
RNA epigenetic modifications commonly include 5-methylcytidine (m5C) (Bohnsack et al., 2019), N6-methyladenosine (m6A) (Oerum et al., 2021), N7-methylguanosine (m7G) (Malbec et al., 2019), N1-methyladenosine (m1A) (Zhou et al., 2019), inosine (I) (Srinivasan et al., 2021), and pseudo uracil (Ψ) And dihydrouracil (D) (Haruehanroengra et al., 2020). m6A modification is closely related to many kinds of carcinogenesis, and altered m6A modification is widely involved in the progression of various tumorigenesis (Gu et al., 2020; Li et al., 2022). Deeply study m6A modification by regulating telomerase activity to maintain telomere homeostasis and genome stability is of great significance to clarify the role of m6A modification in cell aging and carcinogenesis (Table 2). Through Pan-Cancer Analysis of Whole Genomes (PCAWG) analysis of m6A modification of telomerase components, it was found that in most cancers, the expression level of telomerase components was positively correlated with methylase METTL3, negatively correlated with methylase METTL14, negatively correlated with demethylase FTO, negatively correlated with reading proteins YTHDC1, YTHDC2, YTHDF3 and FMR1, and positively correlated with reading proteins HNRNPC, HNRNP2B1, YTHDF1 and RBMX (Wang et al., 2023). These showed that there was a close relationship between telomerase component activity and m6A regulatory factors. With the help of the established CRISPR/dCas13 system to accurately edit the m6A modification platform, it is proved that the METTL3-HMBOX1 axis regulates telomere recruitment and telomere length related to telomerase in cancer cells, and leads to DNA damage reaction (Figure 8) (Lee et al., 2021). METTL3 promotes the stabilization of p53 protein and the expression of target genes in response to DNA damage and carcinogenic signals through catalytic activity dependent and independent mechanisms (Zhao et al., 2020; Raj et al., 2022). In addition, METTL3-m6A-p53 axis may be a potential target for the treatment of hepatocellular carcinoma (HCC) (Ke et al., 2022). Therefore, we can use CRISPR system to modify specific target genes with m6A, and regulate telomerase activity by regulating p53 signal pathway to maintain telomere homeostasis (Figure 9).
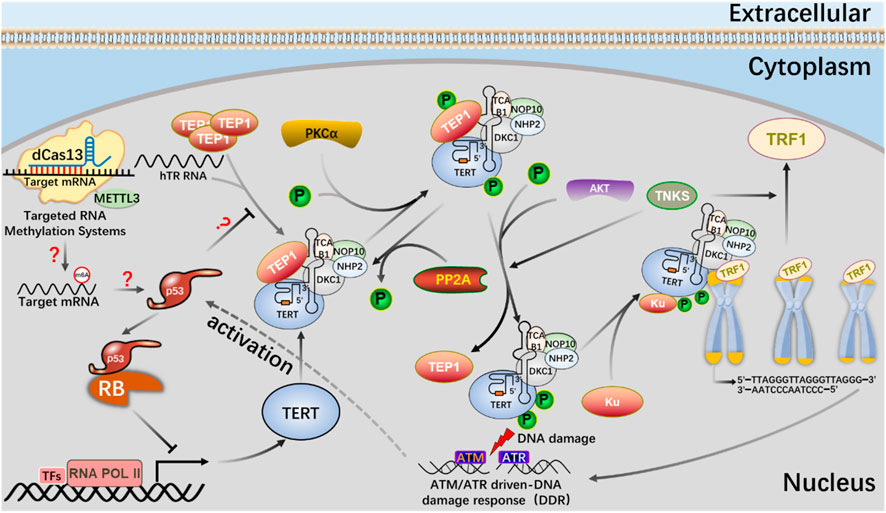
FIGURE 9. The mechanism of the m6A editing tool of CRISPR system to regulate telomerase activity and maintain telomere length in the p53 signal pathway.
dCas13b-METTL3, a m6A editing tool based on CRISPR system, proves that METTL3-catalyzed HMBOX1 methylation is involved in regulating telomerase recruitment, resulting in telomere loss in cancer cells, and m6A is involved in carcinogenesis.
The m6A editing tool of CRISPR system modifies mRNA with m6A, affects telomerase activity through p53 signal pathway, participates in phosphorylation of PKC and AKT or dephosphorylation of PP2A, telomere shortening leads to DNA damage, and activates p53 signal pathway.
Through its reading protein, m6A modification is widely involved in biological processes such as pre-mRNA splicing, RNA output, mRNA translation and RNA degradation, and regulates the stability of targeted mRNA (He and He, 2021). In the study of m6A reading protein, it was found that proteins containing YTH domain (YTHDF1 and YTHDC1) used YTH domain to recognize m6A modification, YTHDF1 and YTHDF3 worked together to affect the translation of m6A containing mRNA, YTHDF2 accelerated the decay of mRNA, and YTHDC1 affected the nuclear processing of its target, further regulating the function and fate of m6A labeled mRNA (Roundtree et al., 2017b; Hsu et al., 2017; Shi et al., 2017).
Knockout of YTHDF1 by CRISPR/Cas9 system will destroy the interaction between YT521-B homologous domain of YTHDF1 and AGO2 (argonaute 2), leading to the transformation of AGO2 droplets into gel/solids deposited in the cytoplasm (Li et al., 2022). In the nucleus, AGO2 interacts with 23 nt sRNA produced by TTS of telomerase RNA component telomerase RNA component (TERC) (position 425–447), which is called terc-sRNA. TERT and TERC constitute the core telomerase that maintains telomere length. As an RNA-binding protein, AGO2 has been found to promote telomerase activity and stimulate the association between TERT and TERC (Figure 10). AGO2 depletion leads to shorter telomeres and lower cell proliferation rate in vitro and in vivo (Laudadio et al., 2019). By regulating the recognition protein YTHDF1, it can regulate the consumption of AGO2 in the cytoplasm, affect the content of AGO2 in the nucleus, and lead to the change of telomerase activity in cells, which may lay the foundation for new therapeutic targets of tumor and telomeric diseases.
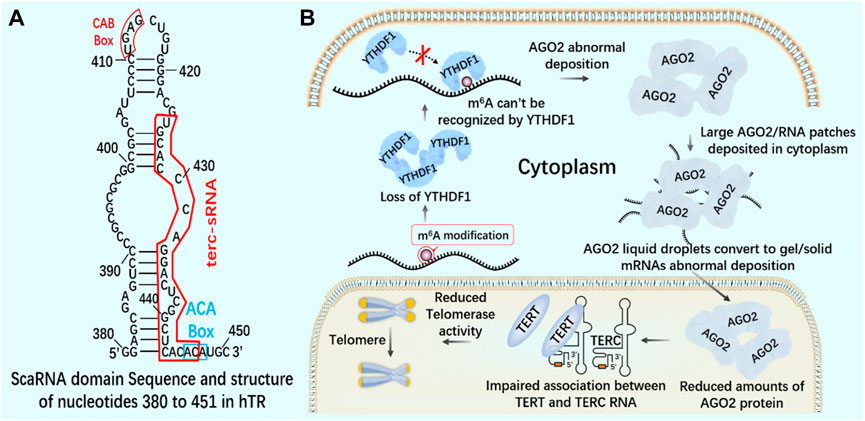
FIGURE 10. m6A reading protein and telomerase (A) The interaction between AGO2 and 23 nt sRNA produced by TTS of telomerase RNA component TERC (position 425–447) - terc-sRNA. (B) YTHDF1 interacts with AGO2 through YTH domain. YTHDF1 downregulates and destroys the interaction between YTHDF1 and AGO2. AGO2 deposits abnormally in the cytoplasm. AGO2 depletion destroys the association between TERT and TERC RNA, reduces telomerase activity, and leads to telomere shortening.
6.2 Site-directed modification of telomerase by dm6ACRISPR system
After CRISPR/Cas9 system, CRISPR/Cas13 system of type VI belongs to a known type that specifically binds and cleaves exogenous RNA (Abudayyeh et al., 2016; Shmakov et al., 2017; Smargon et al., 2017). CRISPR/Cas13 system can resist pathogenic RNA virus or regulate gene expression, and promote the knockout of mRNA, circular RNA and non-coding RNA (Wessels et al., 2020; Li et al., 2021). In addition, CRISPR/Cas13 system has been used for RNA modification in vivo, including editable regulation of selective splicing, A-to-I and C-to-U editing and m6A modification (O'Connell, 2019; Kordyś et al., 2022). Using CRISPR/Cas13 system, m6A can be added to specific RNA sites in a targeted way to achieve precise m6A modification at specific RNA sites. Since the methylation and demethylation process of m6A mainly occurs in the nucleus, two nuclear localization signal (NLS) peptides are added to dCasRx-METTL3 and dCasRx-ALKBH5 editors to realize the nuclear localization of the editing complex, which are called NLS-dCasRx-NLS-METTL3 and NLS-dCasRx-NLS-ALKBH5 (Xia et al., 2021). m6A methyltransferase METTL3 can increase the methylation modification level of telomerase related gene Cbf5 mRNA, promote its transcription and translation, and enhance telomerase activity (Jiang et al., 2021). As a nuclear protein reverse transcriptase, telomerase is composed of RNA template and catalytic protein (Wang et al., 2019). There is a 5-nt GGACU sequence with m6A common motif matching in the H/ACA scaRNA structure of hTR (Han et al., 2020), adenosine in the motif (A435) is located in the double stranded region of the RNA, suggesting that its secondary structure may be affected by m6A modification (Liu et al., 2015). The double stranded structure of the H/ACA scaRNA domain of hTR has been shown to be important for the assembly of telomerase complexes (Zhang et al., 2011). Overexpression of demethylase ALKBH5 leads to a decrease in the assembly efficiency of TCAB1 and DKC1 on telomerase, resulting in a decrease in telomerase activity. This may be mediated by modifying hTR to regulate telomerase assembly and function (Han et al., 2020).
If telomerase activity is regulated by m6A modification, we consider attempting to achieve precise regulation using the nuclear localization CRISPR system combined with dCasRx and NLS. Assuming that the NLS-dCasRx-NLS-METTL3 system overexpressing METTL3 promotes Cbf5 transcription and translation (Figure 11), enhancing telomerase activity, and using NLS-dCasRx-NLS-ALKBH5 overexpressing ALKBH5 to remove m6A modification on hTR, Studying the regulation of TCAB1 and DKC1 assembly on telomerase by m6A modification (Figure 12) provides new insights into the potential application of CRISPR based m6A modification in telomerase regulation.
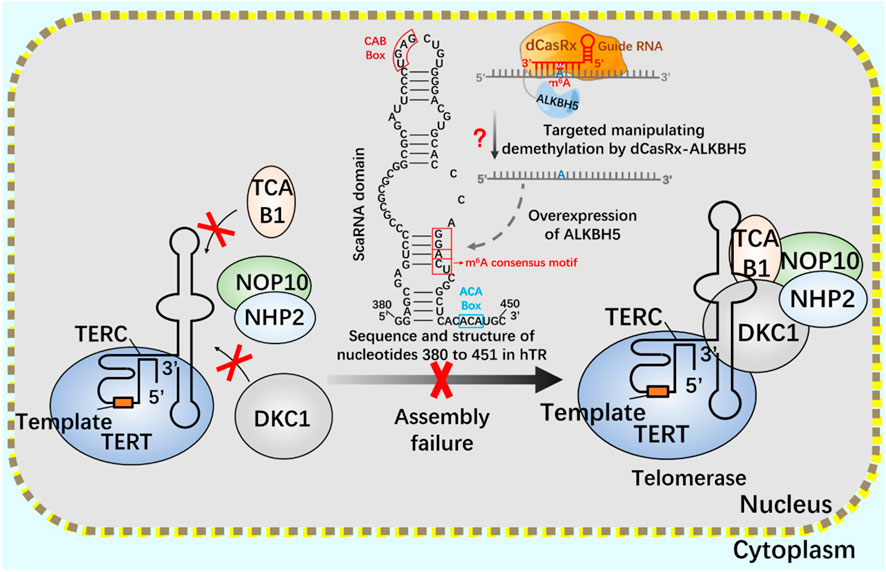
FIGURE 12. Regulation of telomerase assembly by m6A modification of NLS-dCasRx-NLS- ALKBH5 system m6A gene editing tool NLS-dCasRx-NLS-ALKBH5 locates dCasRx-ALKBH5 in the nucleus to achieve specific demethylation. Use the gene editing tool dCasRX-ALKBH5 to modify the m6A demethylation of telomerase hTR, regulate the assembly of telomerase components TCAB1 and DKC1, and reduce cell telomerase activity.
m6A gene editing tool NLS-dCasRx-NLS-METTL3 locates dCasRx-METTL3 in the nucleus to achieve specific methylation. Using the gene editing tool dCasRX-METTL3, the methylation modification level of Cbf5 mRNA was increased, the transcription and translation level of Cbf5 was enhanced, and Cbf5, as a component of telomere synthetase, increased telomere synthetase activity and regulated telomerase activity.
7 Conclusions and future prospects
CRISPR gene editing system, as the most revolutionary breakthrough in the field of biotechnology, is an unprecedented tool to cure human genetic diseases (Gillmore et al., 2021; Fox et al., 2022). m6A modification plays an important role in almost all-important biological processes (Liu et al., 2022; Boulias and Greer, 2022). Telomerase is highly active in stem cells, immune cells and germ cells to maintain telomere length (Jiang et al., 2018; Wan et al., 2021). Using CRISPR system to study the regulation mechanism of m6A modification on telomerase activity is of great significance for exploring the mechanism of cell proliferation and aging.
In this review, we systematically describe the latest application of CRISPR system in m6A modification and the regulation of telomerase activity, providing ideas for understanding the basic mechanism of regulating cell aging. When considering that m6A is the most common, frequent and conservative internal modification, and that telomerase activity is inhibited in normal cells, but remains high in most cancer cells, it is reasonable to propose that further exploring the mechanism of m6A modification on telomerase activity regulation will help to identify and develop gene therapy that can fight aging and treat cancer. It is now clear that the expression and activity of these proteins are essential for the correct regulation of the cell’s non-stop replication process. Strong evidence has emerged about the various functions of these proteins and the corresponding functions of targeted RNA in stem cells, immune cells, germ cells and sperm. So as we continue to decipher the epigenetic modification of m6A and the biology of cell proliferation and aging, we will have an important and in-depth understanding of the molecular mechanism of physiological and pathological cell aging.
Author contributions
MY, MW, YX, and ZC drafted the manuscript. YL, ZZ, and HG designed and revised the manuscript. All authors contributed to the article and approved the submitted version.
Funding
This research was partially supported by National Natural Science Foundation of China (project code: 32272875), Anhui Natural Science Foundation (project code: 2208085MC75) and Anhui Meat Sheep Joint Breeding Project (project code: 340000211260001000431).
Conflict of interest
The authors declare that the research was conducted in the absence of any commercial or financial relationships that could be construed as a potential conflict of interest.
Publisher’s note
All claims expressed in this article are solely those of the authors and do not necessarily represent those of their affiliated organizations, or those of the publisher, the editors and the reviewers. Any product that may be evaluated in this article, or claim that may be made by its manufacturer, is not guaranteed or endorsed by the publisher.
References
Abudayyeh, O. O., Gootenberg, J. S., Konermann, S., Joung, J., Slaymaker, I. M., Cox, D. B., et al. (2016). C2c2 is a single-component programmable RNA-guided RNA-targeting CRISPR effector. Science 353 (6299), aaf5573. doi:10.1126/science.aaf5573
Al-Turki, T. M., and Griffith, J. D. (2023). Mammalian telomeric RNA (TERRA) can be translated to produce valine-arginine and glycine-leucine dipeptide repeat proteins. Proc. Natl. Acad. Sci. U. S. A. 120 (9), e2221529120. doi:10.1073/pnas.2221529120
Anzalone, A. V., Randolph, P. B., Davis, J. R., Sousa, A. A., Koblan, L. W., Levy, J. M., et al. (2019). Search-and-replace genome editing without double-strand breaks or donor DNA. Nature 576 (7785), 149–157. doi:10.1038/s41586-019-1711-4
Bajaj, S., Kumar, M. S., Peters, G. J., and Mayur, Y. C. (2020). Targeting telomerase for its advent in cancer therapeutics. Med. Res. Rev. 40 (5), 1871–1919. doi:10.1002/med.21674
Barthel, F. P., Wei, W., Tang, M., Martinez-Ledesma, E., Hu, X., Amin, S. B., et al. (2017). Systematic analysis of telomere length and somatic alterations in 31 cancer types. Nat. Genet. 49 (3), 349–357. doi:10.1038/ng.3781
Blackburn, E. H., and Collins, K. (2011). Telomerase: An RNP enzyme synthesizes DNA. Cold Spring Harb. Perspect. Biol. 3 (5), 003558. doi:10.1101/cshperspect.a003558
Blasco, M. A. (2005). Telomeres and human disease: Ageing, cancer and beyond. Nat. Rev. Genet. 6 (8), 611–622. doi:10.1038/nrg1656
Bohnsack, K. E., Höbartner, C., and Bohnsack, M. T. (2019). Eukaryotic 5-methylcytosine (m⁵C) RNA methyltransferases: Mechanisms, cellular functions, and links to disease. Genes. (Basel) 10 (2), 102. doi:10.3390/genes10020102
Boulias, K., and Greer, E. L. (2022). Biological roles of adenine methylation in RNA. Nat. Rev. Genet. 24, 143–160. doi:10.1038/s41576-022-00534-0
Cash, D. D., and Feigon, J. (2017). Structure and folding of the Tetrahymena telomerase RNA pseudoknot. Nucleic Acids Res. 45 (1), 482–495. doi:10.1093/nar/gkw1153
Celtikci, B., Erkmen, G. K., and Dikmen, Z. G. (2021). Regulation and effect of telomerase and telomeric length in stem cells. Curr. Stem Cell. Res. Ther. 16 (7), 809–823. doi:10.2174/1574888X15666200422104423
Chakravarti, D., LaBella, K. A., and DePinho, R. A. (2021). Telomeres: History, health, and hallmarks of aging. Cell. 184 (2), 306–322. doi:10.1016/j.cell.2020.12.028
Chen, L., Roake, C. M., Galati, A., Bavasso, F., Micheli, E., Saggio, I., et al. (2020). Loss of human TGS1 hypermethylase promotes increased telomerase RNA and telomere elongation. Cell. Rep. 30 (5), 1358–1372. doi:10.1016/j.celrep.2020.01.004
Chen, M., Wei, L., Law, C. T., Tsang, F. H., Shen, J., Cheng, C. L., et al. (2018). RNA N6-methyladenosine methyltransferase-like 3 promotes liver cancer progression through YTHDF2-dependent posttranscriptional silencing of SOCS2. Hepatology 67 (6), 2254–2270. doi:10.1002/hep.29683
Chen, M., and Wong, C. M. (2020). The emerging roles of N6-methyladenosine (m6A) deregulation in liver carcinogenesis. Mol. Cancer 19 (1), 44. doi:10.1186/s12943-020-01172-y
Chen, X., Zhao, Q., Zhao, Y. L., Chai, G. S., Cheng, W., Zhao, Z., et al. (2021). Targeted RNA N(6) -methyladenosine demethylation controls cell fate transition in human pluripotent stem cells. Adv. Sci. (Weinh) 8 (11), 2003902. doi:10.1002/advs.202003902
Chen, Y., Yang, S., Peng, S., Li, W., Wu, F., Yao, Q., et al. (2019). N1-Methyladenosine detection with CRISPR-Cas13a/C2c2. Chem. Sci. 10 (10), 2975–2979. doi:10.1039/c8sc03408g
Cong, L., Ran, F. A., Cox, D., Lin, S., Barretto, R., Habib, N., et al. (2013). Multiplex genome engineering using CRISPR/Cas systems. Science 339 (6121), 819–823. doi:10.1126/science.1231143
Cui, Q., Shi, H., Ye, P., Li, L., Qu, Q., Sun, G., et al. (2017). m(6 A RNA methylation regulates the self-renewal and tumorigenesis of glioblastoma stem cells. Cell. Rep. 18 (11), 2622–2634. doi:10.1016/j.celrep.2017.02.059
Dai, W., Xu, X., Wang, D., Wu, J., and Wang, J. (2019). Cancer therapy with a CRISPR-assisted telomerase-activating gene expression system. Oncogene 38 (21), 4110–4124. doi:10.1038/s41388-019-0707-8
Demanelis, K., Jasmine, F., Chen, L. S., Chernoff, M., Tong, L., Delgado, D., et al. (2020). Determinants of telomere length across human tissues. Science 369 (6509), 6876. doi:10.1126/science.aaz6876
Dogan, F., and Forsyth, N. R. (2021). Epigenetic features in regulation of telomeres and telomerase in stem cells. Emerg. Top. Life Sci. 5 (4), 497–505. doi:10.1042/ETLS20200344
Dominissini, D., Moshitch-Moshkovitz, S., Schwartz, S., Salmon-Divon, M., Ungar, L., Osenberg, S., et al. (2012). Topology of the human and mouse m6A RNA methylomes revealed by m6A-seq. Nature 485 (7397), 201–206. doi:10.1038/nature11112
Enache, O. M., Rendo, V., Abdusamad, M., Lam, D., Davison, D., Pal, S., et al. (2020). Cas9 activates the p53 pathway and selects for p53-inactivating mutations. Nat. Genet. 52 (7), 662–668. doi:10.1038/s41588-020-0623-4
Everette, K. A., Newby, G. A., Levine, R. M., Mayberry, K., Jang, Y., Mayuranathan, T., et al. (2023). Ex vivo prime editing of patient haematopoietic stem cells rescues sickle-cell disease phenotypes after engraftment in mice. Nat. Biomed. Eng. 7, 616–628. doi:10.1038/s41551-023-01026-0
Fischer, N. W., Prodeus, A., Malkin, D., and Gariépy, J. (2016). p53 oligomerization status modulates cell fate decisions between growth, arrest and apoptosis. Cell. Cycle 15 (23), 3210–3219. doi:10.1080/15384101.2016.1241917
Fox, T. A., Houghton, B. C., Petersone, L., Waters, E., Edner, N. M., McKenna, A., et al. (2022). Therapeutic gene editing of T cells to correct CTLA-4 insufficiency. Sci. Transl. Med. 14 (668), eabn5811. doi:10.1126/scitranslmed.abn5811
Ghaemi, A., Bagheri, E., Abnous, K., Taghdisi, S. M., Ramezani, M., and Alibolandi, M. (2021). CRISPR-cas9 genome editing delivery systems for targeted cancer therapy. Life Sci. 267, 118969. doi:10.1016/j.lfs.2020.118969
Gillmore, J. D., Gane, E., Taubel, J., Kao, J., Fontana, M., Maitland, M. L., et al. (2021). CRISPR-Cas9 in vivo gene editing for transthyretin amyloidosis. N. Engl. J. Med. 385 (6), 493–502. doi:10.1056/NEJMoa2107454
Gu, C., Shi, X., Dai, C., Shen, F., Rocco, G., Chen, J., et al. (2020). RNA m(6)A modification in cancers: Molecular mechanisms and potential clinical applications. Innov. (Camb) 1 (3), 100066. doi:10.1016/j.xinn.2020.100066
Gu, P., Jia, S., Takasugi, T., Smith, E., Nandakumar, J., Hendrickson, E., et al. (2018). CTC1-STN1 coordinates G- and C-strand synthesis to regulate telomere length. Aging Cell. 17 (4), e12783. doi:10.1111/acel.12783
Han, J., Wang, J. Z., Yang, X., Yu, H., Zhou, R., Lu, H. C., et al. (2019). METTL3 promote tumor proliferation of bladder cancer by accelerating pri-miR221/222 maturation in m6A-dependent manner. Mol. Cancer 18 (1), 110. doi:10.1186/s12943-019-1036-9
Han, S., Zhao, B. S., Myers, S. A., Carr, S. A., He, C., and Ting, A. Y. (2020). RNA-protein interaction mapping via MS2- or Cas13-based APEX targeting. Proc. Natl. Acad. Sci. U. S. A. 117 (36), 22068–22079. doi:10.1073/pnas.2006617117
Haruehanroengra, P., Zheng, Y. Y., Zhou, Y., Huang, Y., and Sheng, J. (2020). RNA modifications and cancer. RNA Biol. 17 (11), 1560–1575. doi:10.1080/15476286.2020.1722449
He, P. C., and He, C. (2021). m(6) A RNA methylation: from mechanisms to therapeutic potential. Embo J. 40 (3), e105977. doi:10.15252/embj.2020105977
He, Y., Wang, Y., Liu, B., Helmling, C., Sušac, L., Cheng, R., et al. (2021). Structures of telomerase at several steps of telomere repeat synthesis. Nature 593 (7859), 454–459. doi:10.1038/s41586-021-03529-9
Höijer, I., Emmanouilidou, A., Östlund, R., van Schendel, R., Bozorgpana, S., Tijsterman, M., et al. (2022). CRISPR-Cas9 induces large structural variants at on-target and off-target sites in vivo that segregate across generations. Nat. Commun. 13 (1), 627. doi:10.1038/s41467-022-28244-5
Hsu, P. D., Lander, E. S., and Zhang, F. (2014). Development and applications of CRISPR-Cas9 for genome engineering. Cell. 157 (6), 1262–1278. doi:10.1016/j.cell.2014.05.010
Hsu, P. J., Zhu, Y., Ma, H., Guo, Y., Shi, X., Liu, Y., et al. (2017). Ythdc2 is an N(6)-methyladenosine binding protein that regulates mammalian spermatogenesis. Cell. Res. 27 (9), 1115–1127. doi:10.1038/cr.2017.99
Hu, Y., Gong, C., Li, Z., Liu, J., Chen, Y., Huang, Y., et al. (2022). Demethylase ALKBH5 suppresses invasion of gastric cancer via PKMYT1 m6A modification. Mol. Cancer 21 (1), 34. doi:10.1186/s12943-022-01522-y
Ihry, R. J., Worringer, K. A., Salick, M. R., Frias, E., Ho, D., Theriault, K., et al. (2018). p53 inhibits CRISPR-Cas9 engineering in human pluripotent stem cells. Nat. Med. 24 (7), 939–946. doi:10.1038/s41591-018-0050-6
Jiang, J., Wang, Y., Sušac, L., Chan, H., Basu, R., Zhou, Z. H., et al. (2018). Structure of telomerase with telomeric DNA. Cell. 173 (5), 1179–1190. doi:10.1016/j.cell.2018.04.038
Jiang, X., Xing, L., Chen, Y., Qin, R., Song, S., Lu, Y., et al. (2021). CircMEG3 inhibits telomerase activity by reducing Cbf5 in human liver cancer stem cells. Mol. Ther. Nucleic Acids 23, 310–323. doi:10.1016/j.omtn.2020.11.009
Jinek, M., Chylinski, K., Fonfara, I., Hauer, M., Doudna, J. A., and Charpentier, E. (2012). A programmable dual-RNA-guided DNA endonuclease in adaptive bacterial immunity. Science 337 (6096), 816–821. doi:10.1126/science.1225829
Joung, J., Kirchgatterer, P. C., Singh, A., Cho, J. H., Nety, S. P., Larson, R. C., et al. (2022). CRISPR activation screen identifies BCL-2 proteins and B3GNT2 as drivers of cancer resistance to T cell-mediated cytotoxicity. Nat. Commun. 13 (1), 1606. doi:10.1038/s41467-022-29205-8
Kantor, A., McClements, M. E., and MacLaren, R. E. (2020). CRISPR-Cas9 DNA base-editing and prime-editing. Int. J. Mol. Sci. 21 (17), 6240. doi:10.3390/ijms21176240
Katti, A., Diaz, B. J., Caragine, C. M., Sanjana, N. E., and Dow, L. E. (2022). CRISPR in cancer biology and therapy. Nat. Rev. Cancer 22 (5), 259–279. doi:10.1038/s41568-022-00441-w
Ke, W., Zhang, L., Zhao, X., and Lu, Z. (2022). p53 m(6 A modulation sensitizes hepatocellular carcinoma to apatinib through apoptosis. Apoptosis 27 (5-6), 426–440. doi:10.1007/s10495-022-01728-x
Killela, P. J., Reitman, Z. J., Jiao, Y., Bettegowda, C., Agrawal, N., Diaz, L. A., et al. (2013). TERT promoter mutations occur frequently in gliomas and a subset of tumors derived from cells with low rates of self-renewal. Proc. Natl. Acad. Sci. U. S. A. 110 (15), 6021–6026. doi:10.1073/pnas.1303607110
Kim, D. Y., Moon, S. B., Ko, J. H., Kim, Y. S., and Kim, D. (2020). Unbiased investigation of specificities of prime editing systems in human cells. Nucleic Acids Res. 48 (18), 10576–10589. doi:10.1093/nar/gkaa764
Konermann, S., Lotfy, P., Brideau, N. J., Oki, J., Shokhirev, M. N., and Hsu, P. D. (2018). Transcriptome engineering with RNA-targeting type VI-D CRISPR effectors. Cell. 173 (3), 665–676. doi:10.1016/j.cell.2018.02.033
Kordyś, M., Sen, R., and Warkocki, Z. (2022). Applications of the versatile CRISPR-Cas13 RNA targeting system. Wiley Interdiscip. Rev. RNA 13 (3), 1694. doi:10.1002/wrna.1694
Kühn, R., and Chu, V. T. (2015). Pop in, pop out: A novel gene-targeting strategy for use with CRISPR-cas9. Genome Biol. 16, 244. doi:10.1186/s13059-015-0810-2
Kushawah, G., Hernandez-Huertas, L., Abugattas-Nuñez Del Prado, J., Martinez-Morales, J. R., DeVore, M. L., Hassan, H., et al. (2020). CRISPR-Cas13d induces efficient mRNA knockdown in animal embryos. Dev. Cell. 54 (6), 805–817. doi:10.1016/j.devcel.2020.07.013
Laudadio, I., Orso, F., Azzalin, G., Calabrò, C., Berardinelli, F., Coluzzi, E., et al. (2019). AGO2 promotes telomerase activity and interaction between the telomerase components TERT and TERC. EMBO Rep. 20 (2), 45969. doi:10.15252/embr.201845969
Lee, J. H., Hong, J., Zhang, Z., de la Peña Avalos, B., Proietti, C. J., Deamicis, A. R., et al. (2021). Regulation of telomere homeostasis and genomic stability in cancer by N (6)-adenosine methylation (m(6)A). Sci. Adv. 7 (31). doi:10.1126/sciadv.abg7073
Leibowitz, M. L., Papathanasiou, S., Doerfler, P. A., Blaine, L. J., Sun, L., Yao, Y., et al. (2021). Chromothripsis as an on-target consequence of CRISPR-Cas9 genome editing. Nat. Genet. 53 (6), 895–905. doi:10.1038/s41588-021-00838-7
Li, J., Chen, K., Dong, X., Xu, Y., Sun, Q., Wang, H., et al. (2022a). YTHDF1 promotes mRNA degradation via YTHDF1-AGO2 interaction and phase separation. Cell. Prolif. 55 (1), e13157. doi:10.1111/cpr.13157
Li, J., Chen, Z., Chen, F., Xie, G., Ling, Y., Peng, Y., et al. (2020a). Targeted mRNA demethylation using an engineered dCas13b-ALKBH5 fusion protein. Nucleic Acids Res. 48 (10), 5684–5694. doi:10.1093/nar/gkaa269
Li, S., Li, X., Xue, W., Zhang, L., Yang, L. Z., Cao, S. M., et al. (2021). Screening for functional circular RNAs using the CRISPR-Cas13 system. Nat. Methods 18 (1), 51–59. doi:10.1038/s41592-020-01011-4
Li, X., Ma, S., Deng, Y., Yi, P., and Yu, J. (2022b). Targeting the RNA m(6)A modification for cancer immunotherapy. Mol. Cancer 21 (1), 76. doi:10.1186/s12943-022-01558-0
Li, X., Qian, X., Wang, B., Xia, Y., Zheng, Y., Du, L., et al. (2020b). Programmable base editing of mutated TERT promoter inhibits brain tumour growth. Nat. Cell. Biol. 22 (3), 282–288. doi:10.1038/s41556-020-0471-6
Lin, S., Choe, J., Du, P., Triboulet, R., and Gregory, R. I. (2016). The m(6)A methyltransferase METTL3 promotes translation in human cancer cells. Mol. Cell. 62 (3), 335–345. doi:10.1016/j.molcel.2016.03.021
Liu, B., He, Y., Wang, Y., Song, H., Zhou, Z. H., and Feigon, J. (2022a). Structure of active human telomerase with telomere shelterin protein TPP1. Nature 604 (7906), 578–583. doi:10.1038/s41586-022-04582-8
Liu, L., Li, H., Hu, D., Wang, Y., Shao, W., Zhong, J., et al. (2022b). Insights into N6-methyladenosine and programmed cell death in cancer. Mol. Cancer 21 (1), 32. doi:10.1186/s12943-022-01508-w
Liu, N., Dai, Q., Zheng, G., He, C., Parisien, M., and Pan, T. (2015). N(6)-methyladenosine-dependent RNA structural switches regulate RNA-protein interactions. Nature 518 (7540), 560–564. doi:10.1038/nature14234
Liu, X. M., Zhou, J., Mao, Y., Ji, Q., and Qian, S. B. (2019). Programmable RNA N(6)-methyladenosine editing by CRISPR-Cas9 conjugates. Nat. Chem. Biol. 15 (9), 865–871. doi:10.1038/s41589-019-0327-1
Liu, Z., Liao, Z., Chen, Y., Han, L., Yin, Q., and Xiao, H. (2020). Application of various delivery methods for CRISPR/dCas9. Mol. Biotechnol. 62 (8), 355–363. doi:10.1007/s12033-020-00258-8
Lupatov, A. Y., and Yarygin, K. N. (2022). Telomeres and telomerase in the control of stem cells. Biomedicines 10 (10), 2335. doi:10.3390/biomedicines10102335
Ma, J. Z., Yang, F., Zhou, C. C., Liu, F., Yuan, J. H., Wang, F., et al. (2017). METTL14 suppresses the metastatic potential of hepatocellular carcinoma by modulating N(6) -methyladenosine-dependent primary MicroRNA processing. Hepatology 65 (2), 529–543. doi:10.1002/hep.28885
Malbec, L., Zhang, T., Chen, Y. S., Zhang, Y., Sun, B. F., Shi, B. Y., et al. (2019). Dynamic methylome of internal mRNA N(7)-methylguanosine and its regulatory role in translation. Cell. Res. 29 (11), 927–941. doi:10.1038/s41422-019-0230-z
Manghwar, H., Lindsey, K., Zhang, X., and Jin, S. (2019). CRISPR/Cas system: Recent advances and future prospects for genome editing. Trends Plant Sci. 24 (12), 1102–1125. doi:10.1016/j.tplants.2019.09.006
Negrini, S., De Palma, R., and Filaci, G. (2020). Anti-cancer immunotherapies targeting telomerase. Cancers (Basel) 12 (8), 2260. doi:10.3390/cancers12082260
O'Connell, M. R. (2019). Molecular mechanisms of RNA targeting by cas13-containing type VI CRISPR-cas systems. J. Mol. Biol. 431 (1), 66–87. doi:10.1016/j.jmb.2018.06.029
Oerum, S., Meynier, V., Catala, M., and Tisné, C. (2021). A comprehensive review of m6A/m6Am RNA methyltransferase structures. Nucleic Acids Res. 49 (13), 7239–7255. doi:10.1093/nar/gkab378
Peng, H., Chen, B., Wei, W., Guo, S., Han, H., Yang, C., et al. (2022). N(6)-methyladenosine (m(6)A) in 18S rRNA promotes fatty acid metabolism and oncogenic transformation. Nat. Metab. 4 (8), 1041–1054. doi:10.1038/s42255-022-00622-9
Raj, N., Wang, M., Seoane, J. A., Zhao, R. L., Kaiser, A. M., Moonie, N. A., et al. (2022). The Mettl3 epitranscriptomic writer amplifies p53 stress responses. Mol. Cell. 82 (13), 2370–2384. doi:10.1016/j.molcel.2022.04.010
Roake, C. M., and Artandi, S. E. (2020). Regulation of human telomerase in homeostasis and disease. Nat. Rev. Mol. Cell. Biol. 21 (7), 384–397. doi:10.1038/s41580-020-0234-z
Rossiello, F., Jurk, D., Passos, J. F., and d'Adda di Fagagna, F. (2022). Telomere dysfunction in ageing and age-related diseases. Nat. Cell. Biol. 24 (2), 135–147. doi:10.1038/s41556-022-00842-x
Roundtree, I. A., Evans, M. E., Pan, T., and He, C. (2017a). Dynamic RNA modifications in gene expression regulation. Cell. 169 (7), 1187–1200. doi:10.1016/j.cell.2017.05.045
Roundtree, I. A., Luo, G. Z., Zhang, Z., Wang, X., Zhou, T., Cui, Y., et al. (2017b). YTHDC1 mediates nuclear export of N(6)-methyladenosine methylated mRNAs. Elife 6, 31311. doi:10.7554/eLife.31311
Schmidt, J. C., Zaug, A. J., and Cech, T. R. (2016). Live cell imaging reveals the dynamics of telomerase recruitment to telomeres. Cell. 166 (5), 1188–1197. doi:10.1016/j.cell.2016.07.033
Schmidt, W., Arnold, H. H., and Kersten, H. (1975). Biosynthetic pathway of ribothymidine in B. subtilis and M. lysodeikticus involving different coenzymes for transfer RNA and ribosomal RNA. Nucleic Acids Res. 2 (7), 1043–1051. doi:10.1093/nar/2.7.1043
Sekne, Z., Ghanim, G. E., van Roon, A. M., and Nguyen, T. H. D. (2022). Structural basis of human telomerase recruitment by TPP1-POT1. Science 375 (6585), 1173–1176. doi:10.1126/science.abn6840
Shay, J. W. (2016). Role of telomeres and telomerase in aging and cancer. Cancer Discov. 6 (6), 584–593. doi:10.1158/2159-8290.CD-16-0062
Shi, H., Wang, X., Lu, Z., Zhao, B. S., Ma, H., Hsu, P. J., et al. (2017). YTHDF3 facilitates translation and decay of N(6)-methyladenosine-modified RNA. Cell. Res. 27 (3), 315–328. doi:10.1038/cr.2017.15
Shi, H. Z., Xiong, J. S., Gan, L., Zhang, Y., Zhang, C. C., Kong, Y. Q., et al. (2022). N6-methyladenosine reader YTHDF3 regulates melanoma metastasis via its 'executor'LOXL3. Clin. Transl. Med. 12 (11), 1075. doi:10.1002/ctm2.1075
Shmakov, S., Smargon, A., Scott, D., Cox, D., Pyzocha, N., Yan, W., et al. (2017). Diversity and evolution of class 2 CRISPR-Cas systems. Nat. Rev. Microbiol. 15 (3), 169–182. doi:10.1038/nrmicro.2016.184
Sinha, S., Barbosa, K., Cheng, K., Leiserson, M. D. M., Jain, P., Deshpande, A., et al. (2021). A systematic genome-wide mapping of oncogenic mutation selection during CRISPR-Cas9 genome editing. Nat. Commun. 12 (1), 6512. doi:10.1038/s41467-021-26788-6
Smargon, A. A., Cox, D. B. T., Pyzocha, N. K., Zheng, K., Slaymaker, I. M., Gootenberg, J. S., et al. (2017). Cas13b is a type VI-B CRISPR-associated RNA-guided RNase differentially regulated by accessory proteins Csx27 and Csx28. Mol. Cell. 65 (4), 618–630. doi:10.1016/j.molcel.2016.12.023
Srinivasan, S., Torres, A. G., and Ribas de Pouplana, L. (2021). Inosine in biology and disease. Genes. (Basel) 12 (4), 600. doi:10.3390/genes12040600
Sun, L., Chiang, J. Y., Choi, J. Y., Xiong, Z. M., Mao, X., Collins, F. S., et al. (2019). Transient induction of telomerase expression mediates senescence and reduces tumorigenesis in primary fibroblasts. Proc. Natl. Acad. Sci. U. S. A. 116 (38), 18983–18993. doi:10.1073/pnas.1907199116
Tang, C., Xie, Y., Yu, T., Liu, N., Wang, Z., Woolsey, R. J., et al. (2020). m(6 A-dependent biogenesis of circular RNAs in male germ cells. Cell. Res. 30 (3), 211–228. doi:10.1038/s41422-020-0279-8
Trybek, T., Kowalik, A., Góźdź, S., and Kowalska, A. (2020). Telomeres and telomerase in oncogenesis. Oncol. Lett. 20 (2), 1015–1027. doi:10.3892/ol.2020.11659
Ushijima, T., Clark, S. J., and Tan, P. (2021). Mapping genomic and epigenomic evolution in cancer ecosystems. Science 373 (6562), 1474–1479. doi:10.1126/science.abh1645
Visvanathan, A., Patil, V., Arora, A., Hegde, A. S., Arivazhagan, A., Santosh, V., et al. (2018). Essential role of METTL3-mediated m(6)A modification in glioma stem-like cells maintenance and radioresistance. Oncogene 37 (4), 522–533. doi:10.1038/onc.2017.351
Wan, F., Ding, Y., Zhang, Y., Wu, Z., Li, S., Yang, L., et al. (2021). Zipper head mechanism of telomere synthesis by human telomerase. Cell. Res. 31 (12), 1275–1290. doi:10.1038/s41422-021-00586-7
Wang, J., Dai, M., Xing, X., Wang, X., Qin, X., Huang, T., et al. (2023). Genomic, epigenomic, and transcriptomic signatures for telomerase complex components: A pan-cancer analysis. Mol. Oncol. 17 (1), 150–172. doi:10.1002/1878-0261.13324
Wang, S., Guo, M., Zhu, Y., Lin, Z., and Huang, Z. (2022). Cryo-EM structure of the type III-E CRISPR-Cas effector gRAMP in complex with TPR-CHAT. Cell. Res. 32, 1128–1131. doi:10.1038/s41422-022-00738-3
Wang, Y., Sušac, L., and Feigon, J. (2019). Structural biology of telomerase. Cold Spring Harb. Perspect. Biol. 11 (12), 032383. doi:10.1101/cshperspect.a032383
Wei, J., Yu, X., Yang, L., Liu, X., Gao, B., Huang, B., et al. (2022). FTO mediates LINE1 m(6)A demethylation and chromatin regulation in mESCs and mouse development. Science 376 (6596), 968–973. doi:10.1126/science.abe9582
Wessels, H. H., Méndez-Mancilla, A., Guo, X., Legut, M., Daniloski, Z., and Sanjana, N. E. (2020). Massively parallel Cas13 screens reveal principles for guide RNA design. Nat. Biotechnol. 38 (6), 722–727. doi:10.1038/s41587-020-0456-9
Wilson, C., Chen, P. J., Miao, Z., and Liu, D. R. (2020). Programmable m(6)A modification of cellular RNAs with a Cas13-directed methyltransferase. Nat. Biotechnol. 38 (12), 1431–1440. doi:10.1038/s41587-020-0572-6
Wu, L., Fidan, K., Um, J. Y., and Ahn, K. S. (2020). Telomerase: Key regulator of inflammation and cancer. Pharmacol. Res. 155, 104726. doi:10.1016/j.phrs.2020.104726
Wu, X., Wu, J., Dai, J., Chen, B., Chen, Z., Wang, S., et al. (2021). Aggregation-induced emission luminogens reveal cell cycle-dependent telomerase activity in cancer cells. Natl. Sci. Rev. 8 (6), 306. doi:10.1093/nsr/nwaa306
Wu, Y., Chen, Z., Xie, G., Zhang, H., Wang, Z., Zhou, J., et al. (2022). RNA m(1)A methylation regulates glycolysis of cancer cells through modulating ATP5D. Proc. Natl. Acad. Sci. U. S. A. 119 (28), 2119038119. doi:10.1073/pnas.2119038119
Xi, L., Schmidt, J. C., Zaug, A. J., Ascarrunz, D. R., and Cech, T. R. (2015). A novel two-step genome editing strategy with CRISPR-Cas9 provides new insights into telomerase action and TERT gene expression. Genome Biol. 16, 231. doi:10.1186/s13059-015-0791-1
Xia, Z., Tang, M., Ma, J., Zhang, H., Gimple, R. C., Prager, B. C., et al. (2021). Epitranscriptomic editing of the RNA N6-methyladenosine modification by dCasRx conjugated methyltransferase and demethylase. Nucleic Acids Res. 49 (13), 7361–7374. doi:10.1093/nar/gkab517
Xie, S., Jin, H., Yang, F., Zheng, H., Chang, Y., Liao, Y., et al. (2021). Programmable RNA N(1) -methyladenosine demethylation by a cas13d-directed demethylase. Angew. Chem. Int. Ed. Engl. 60 (36), 19592–19597. doi:10.1002/anie.202105253
Xu, C., Xie, N., Su, Y., Sun, Z., Liang, Y., Zhang, N., et al. (2020). HnRNP F/H associate with hTERC and telomerase holoenzyme to modulate telomerase function and promote cell proliferation. Cell. Death Differ. 27 (6), 1998–2013. doi:10.1038/s41418-019-0483-6
Yang, L. Z., Wang, Y., Li, S. Q., Yao, R. W., Luan, P. F., Wu, H., et al. (2019). Dynamic imaging of RNA in living cells by CRISPR-cas13 systems. Mol. Cell. 76 (6), 981–997. doi:10.1016/j.molcel.2019.10.024
Yang, Y., Hsu, P. J., Chen, Y. S., and Yang, Y. G. (2018). Dynamic transcriptomic m(6)A decoration: Writers, erasers, readers and functions in RNA metabolism. Cell. Res. 28 (6), 616–624. doi:10.1038/s41422-018-0040-8
Ye, L., Park, J. J., Peng, L., Yang, Q., Chow, R. D., Dong, M. B., et al. (2022). A genome-scale gain-of-function CRISPR screen in CD8 T cells identifies proline metabolism as a means to enhance CAR-T therapy. Cell. Metab. 34 (4), 595–614. doi:10.1016/j.cmet.2022.02.009
Ying, X., Jiang, X., Zhang, H., Liu, B., Huang, Y., Zhu, X., et al. (2020). Programmable N6-methyladenosine modification of CDCP1 mRNA by RCas9-methyltransferase like 3 conjugates promotes bladder cancer development. Mol. Cancer 19 (1), 169. doi:10.1186/s12943-020-01289-0
Yu, G., Kim, H. K., Park, J., Kwak, H., Cheong, Y., Kim, D., et al. (2023). Prediction of efficiencies for diverse prime editing systems in multiple cell types. Cell. 186 (10), 2256–2272. doi:10.1016/j.cell.2023.03.034
Yu, G., Wang, X., Zhang, Y., An, Q., Wen, Y., Li, X., et al. (2022). Structure and function of a bacterial type III-E CRISPR-Cas7-11 complex. Nat. Microbiol. 7, 2078–2088. doi:10.1038/s41564-022-01256-z
Zhan, T., Rindtorff, N., Betge, J., Ebert, M. P., and Boutros, M. (2019). CRISPR/Cas9 for cancer research and therapy. Semin. Cancer Biol. 55, 106–119. doi:10.1016/j.semcancer.2018.04.001
Zhang, C., Konermann, S., Brideau, N. J., Lotfy, P., Wu, X., Novick, S. J., et al. (2018). Structural basis for the RNA-guided ribonuclease activity of CRISPR-cas13d. Cell. 175 (1), 212–223.e17. doi:10.1016/j.cell.2018.09.001
Zhang, C., Samanta, D., Lu, H., Bullen, J. W., Zhang, H., Chen, I., et al. (2016). Hypoxia induces the breast cancer stem cell phenotype by HIF-dependent and ALKBH5-mediated m⁶A-demethylation of NANOG mRNA. Proc. Natl. Acad. Sci. U. S. A. 113 (14), E2047–E2056. doi:10.1073/pnas.1602883113
Zhang, H., Qin, C., An, C., Zheng, X., Wen, S., Chen, W., et al. (2021). Application of the CRISPR/Cas9-based gene editing technique in basic research, diagnosis, and therapy of cancer. Mol. Cancer 20 (1), 126. doi:10.1186/s12943-021-01431-6
Zhang, Q., Kim, N. K., and Feigon, J. (2011). Architecture of human telomerase RNA. Proc. Natl. Acad. Sci. U. S. A. 108 (51), 20325–20332. doi:10.1073/pnas.1100279108
Zhang, S., Zhao, B. S., Zhou, A., Lin, K., Zheng, S., Lu, Z., et al. (2017). m(6 A demethylase ALKBH5 maintains tumorigenicity of glioblastoma stem-like cells by sustaining FOXM1 expression and cell proliferation program. Cancer Cell. 31 (4), 591–606.e6. doi:10.1016/j.ccell.2017.02.013
Zhao, T., Sun, D., Zhao, M., Lai, Y., Liu, Y., and Zhang, Z. (2020). N(6)-methyladenosine mediates arsenite-induced human keratinocyte transformation by suppressing p53 activation. Environ. Pollut. 259, 113908. doi:10.1016/j.envpol.2019.113908
Zhou, C., Hu, X., Tang, C., Liu, W., Wang, S., Zhou, Y., et al. (2020). CasRx-mediated RNA targeting prevents choroidal neovascularization in a mouse model of age-related macular degeneration. Natl. Sci. Rev. 7 (5), 835–837. doi:10.1093/nsr/nwaa033
Keywords: CRISPR system, m6A modification, epigenetic regulation, telomere, telomerase
Citation: Yi M, Wang M, Xu Y, Cao Z, Ling Y, Zhang Z and Cao H (2023) CRISPR-based m6A modification and its potential applications in telomerase regulation. Front. Cell Dev. Biol. 11:1200734. doi: 10.3389/fcell.2023.1200734
Received: 05 April 2023; Accepted: 27 June 2023;
Published: 14 July 2023.
Edited by:
Guang Ji, Shanghai University of Traditional Chinese Medicine, ChinaReviewed by:
Haiwei Mou, Wistar Institute, United StatesXiao-Min Liu, China Pharmaceutical University, China
Copyright © 2023 Yi, Wang, Xu, Cao, Ling, Zhang and Cao. This is an open-access article distributed under the terms of the Creative Commons Attribution License (CC BY). The use, distribution or reproduction in other forums is permitted, provided the original author(s) and the copyright owner(s) are credited and that the original publication in this journal is cited, in accordance with accepted academic practice. No use, distribution or reproduction is permitted which does not comply with these terms.
*Correspondence: Yinghui Ling, bGluZ3lpbmdodWlAYWhhdS5lZHUuY24=; Zijun Zhang, emhhbmd6aWp1bkBhaGF1LmVkdS5jbg==; Hongguo Cao, Y2FvaG9uZ2d1bzFAYWhhdS5lZHUuY24=
†These authors have contributed equally to this work