- 1Department of Endocrinology, Endocrinology Research Center, The Xiangya Hospital of Central South University, Changsha, China
- 2National Clinical Research Center for Geriatric Disorders, Xiangya Hospital, Changsha, China
- 3Key Laboratory of Organ Injury, Aging and Regenerative Medicine of Hunan Province, Changsha, China
The mammalian skeleton is a metabolically active organ that continuously undergoes bone remodeling, a process of tightly coupled bone resorption and formation throughout life. Recent studies have expanded our knowledge about the interactions between cells within bone marrow in bone remodeling. Macrophages resident in bone (BMMs) can regulate bone metabolism via secreting numbers of cytokines and exosomes. This review summarizes the current understanding of factors, exosomes, and hormones that involved in the communications between BMMs and other bone cells including mensenchymal stem cells, osteoblasts, osteocytes, and so on. We also discuss the role of BMMs and potential therapeutic approaches targeting BMMs in bone remodeling related diseases such as osteoporosis, osteoarthritis, rheumatoid arthritis, and osteosarcoma.
Introduction
Macrophages are diverse, multifunctional, and plastic cells that regulate tissue homeostasis under physiological conditions and in various pathophysiological processes according to the surrounding environment.
Macrophages can be divided into circulating and resident macrophages. During embryonic development, macrophages are also the first emerged cells of the nascent immune system and they would infiltrate various developing organs to differentiate into tissue-resident macrophages, such as bone-resident macrophages (Wynn et al., 2013). Current knowledge of bone-resident macrophages (Michalski and McCauley, 2017) is limited. Osteal macrophages are a subset of bone-resident macrophages and are f4/80 positive and trap negative. Close to the bone surface, osteal macrophages are adjacent to osteoblasts, regulate bone formation, and are closely related to the osteogenic differentiation of mesenchymal stem cells. One characteristic of this group of myeloid cells is that although they share a common precursor with osteoclasts, they have different markers on their surfaces from osteoclasts. Communications between macrophages and other bone cells play an important role in bone tissue homeostasis and new bone formation. In this review, we focus primarily on the effects of macrophages on other bone cells. Extracellular vesicles are a group of cell-derived heterogeneous membranous structures that facilitate cell-cell communications. Hence, we discussed the potential contribution of the new-found microRNAs and alarmins contained in macrophage-derived extracellular vesicles in maintaining bone homeostasis in the context of bone stromal regulation. In the end, we further explained the role of macrophages in bone remodeling-related bone diseases and described the relationship between macrophages and bone tumors such as osteosarcoma.
This review focuses on the presence of macrophages in endosseous tissue, revealing the important role of macrophages in bone physiology and pathology.
Macrophages
The Occurrence and Function of Macrophages
Since Elie Metchnikoff first translated macrophages into Greek “big eaters,” macrophages are primarily known for their phagocytosis in inflammation and immunity (Gordon, 2008). Macrophages are differentiated immune cells with heterogeneity and plasticity. They are activated under different environmental signals and participate in diverse functions (Das et al., 2014, 2015; Jafarnezhad-Ansariha et al., 2018). Macrophage is often referred to as polarization. Different subtypes of macrophages have been derived from the simple M1/M2 classification, according to the environment, transcription factors, and cytokines secreted by macrophages. According to the expression of marker in macrophage surface, M1/M2 are currently also called “M1-like” and “M2-like” (Biswas and Mantovani, 2010), but in this review, we still only use M1 and M2 to represent.
M1 or classical activation of macrophages is an important inflammatory responser. Polarized M1 can produce high levels of reactive oxygen species (ROS), nitric oxide (NO), and pro-inflammatory cytokines such as interleukin IL-1, IL-2, IL-6, IL-12, TNF-α, and IFN-γ, which are involved in enhancing the host's defense response (Mosser, 2003; Genin et al., 2015). However, excessive stimulation of M1 macrophages can lead to tissue damage and autoimmune diseases (Mosser and Edwards, 2008). M2 macrophages mainly present in the subsiding phase of inflammation and are responsible for the production of anti-inflammatory cytokines and the clearance of apoptotic cells. Exposure to anti-inflammatory cytokines (IL-4, IL-10, and IL-13) or IL-1 receptor ligands or immune complexes and toll-like receptors (TLRs) can lead to M2 macrophage polarization (Mantovani et al., 2004; Guihard et al., 2012; Woo et al., 2015). M2 can produce anti-inflammatory cytokines such as chemokines ligands 18 (CCL-18), CCL-22, IL-10, and a small amount of IL-12 family members (Mosser and Edwards, 2008; Guo et al., 2019). In addition, M2 macrophages can produce a large number of osteogenic growth factors such as BMP-2 bone morphogenetic protein-2, a subclass of the TGF-β family and a potent promoter to osteogenic differentiation of MSCs (Champagne et al., 2002; Li et al., 2018), TGF-β (Assoian et al., 1987), osteopontin (Takahashi et al., 2004), and 1,25-dihydroxy-vitamin D3 (Kreutz et al., 1993). M2 can be further subdivided into M2a, M2c, and M2d by different signal activation, cell surface markers, and their functions (Figure 1) (Mosser and Edwards, 2008; Jetten et al., 2014; Murray et al., 2014; Roszer, 2015; Ogle et al., 2016; Arora et al., 2018).
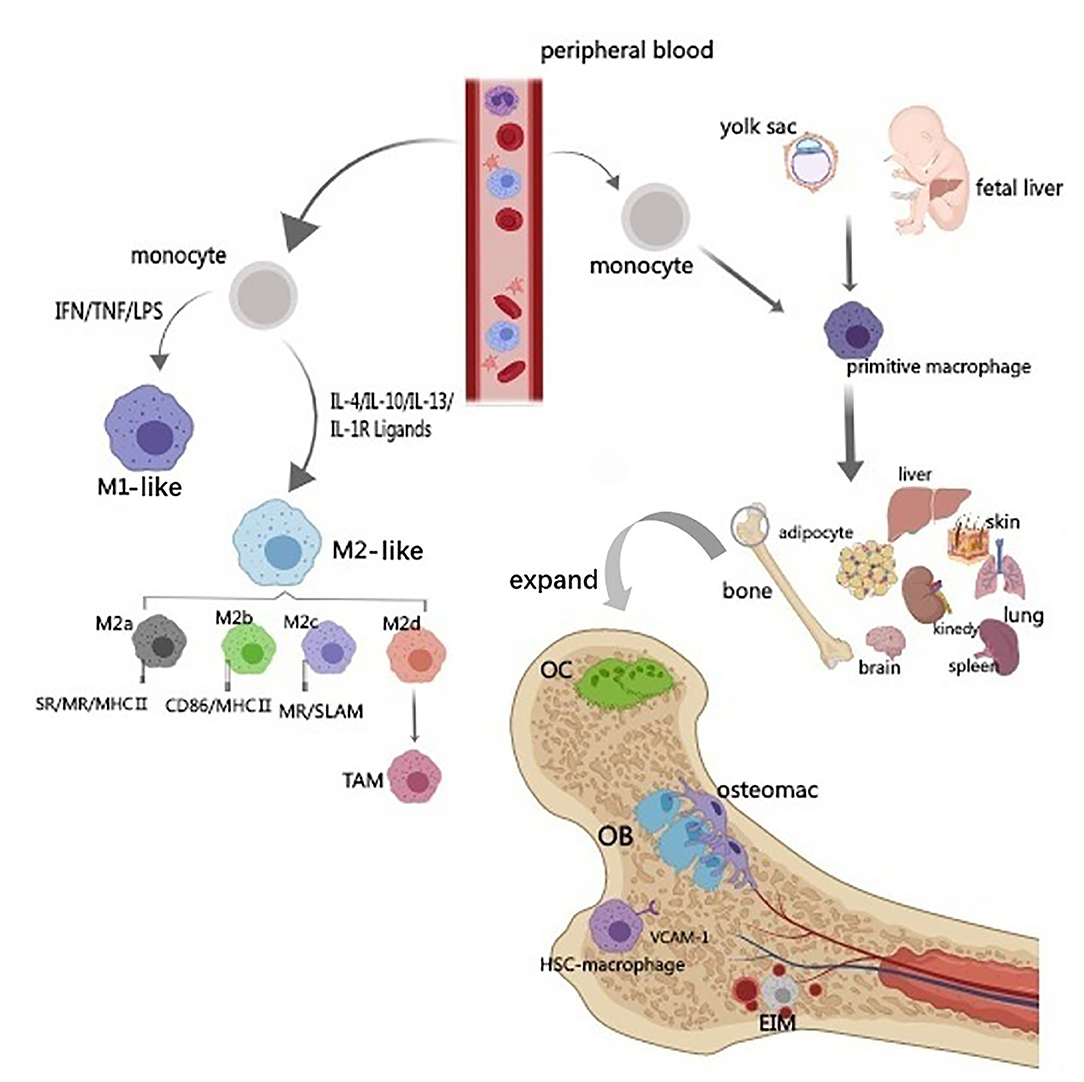
Figure 1. Origin and classification of macrophages. The diagram illustrates the source and classification of macrophages. Circulating macrophages are classified into M1 and M2 types according to their activators and functions. M2-type can be further subdivided into M2a, M2c, and M2d by different signal activation, cell surface markers and their functions. Based on the origin, tissue-resident macrophages can be divided into two subsets. One derives from the yolk sac and another population originates from hematopoietic progenitors and circulating monocytes. Bone macrophage includes bone marrow macrophage (erythroblastic island macrophage, hematopoietic stem cell niche macrophage), osteoclast and osteal macrophages (osteomacs).
Moreover, macrophages can also be divided into circulating and tissue-resident types. Tissue-resident macrophages are a diverse family of cells found in most organs (such as liver kupffer cells and alveolar macrophages in the lungs). Based on the origin, tissue-resident macrophages can be divided into two subsets. One derives from the yolk sac and another population originates from hematopoietic progenitors and circulating monocytes (Figure 1) (Heideveld and van den Akker, 2017). Tissue-resident macrophages express a large number of cell surface receptors, growth factors, proinflammatory and anti-inflammatory cytokines, and many other cell products (Jamalpoor et al., 2018). Most damage-related factors are first sensed by the resident macrophages, which become tense and recruit more macrophages when activated. Resident and recruited macrophages respond to their local environment and activate specific transcriptional programs that drive macrophages to a range of different phenotypes, from pro-inflammatory M1 macrophages to anti-inflammatory M2 macrophages (Xue et al., 2014; Juhas et al., 2018). Tissue damage caused by external (damage, chemicals, infection) and internal triggers (DNA damage, immune response) or by lack (nutrients, oxygen) and excess (sugar, cholesterol) factors may induce macrophage activation, sequentially lead to a disruption of the delicate balance between bone formation and resorption.
One Subset of Bone-Resident Macrophages—Osteal Macrophages
Bone macrophage includes bone marrow macrophage (erythroblastic island macrophage, hematopoietic stem cell niche macrophage), osteoclast and osteal macrophages which also named osteomacs. Osteal macrophages were found in both periosteum and endosseous tissue, which support osteoblastic function and maintain bone homeostasis (Winkler et al., 2010; Cho et al., 2014b; Raggatt et al., 2014; Miron et al., 2016; Michalski and McCauley, 2017). Osteal macrophages account for about one sixth of the total cells in bone tissue (Chang et al., 2008), they present in resting bone tissue and increase in active bone anabolism sites. Osteal macrophages are a unique subset of bone-resident macrophages, close to the bone surface, f4/80 positive and trap-negative (Geissmann et al., 2010), adjacent to bone-forming cells (osteoblasts), dormant cells, and osteoclasts. The osteoblasts on the inner surface of cortical bone were mostly covered by f4/80+, CD68+, mac-3+, and trap-macrophages and be regulated by osteomacs (Chang et al., 2008; Batoon et al., 2019).
CSF-1 (colony stimulating factor 1) and various molecular markers are required for the proliferation and differentiation of mononuclear phagocyte progenitor cells to monocytes, osteal macrophages, and osteoclasts. Previous studies have shown that osteomacs on and in the periosteum are highly expressed with the mature macrophage marker CD169, which can be distinguished from osteoclasts (Mohsenzadegan et al., 2015; Batoon et al., 2019). So far, there is no unique marker between osteal cells and other bone macrophage subsets, but it is known osteal cells that in endosteum do not express ER-HR3 antigen which can be distinguished (Wu et al., 2016; Kaur et al., 2017).
Communications Between Macrophages and Bone Cells
Bone is a kind of mineralized connective tissue, which plays the role of movement, support, and protection of soft tissues, storage of calcium and phosphorus, and preservation of bone marrow (Robling et al., 2006; Datta et al., 2008). Bone tissue is composed of roughly two parts: the dense layer and the spongy layer. The dense layer consists primarily of bone cells that make up the outermost layer of bone and functions primarily to support structural stability of the body and movement. The spongy layer is a trabecular, highly vascularized network of bone that houses the red and white marrow and is a hotbed of hematopoietic blood (Le et al., 2017). Despite bone is inert in appearance, it is a highly dynamic organ, constantly absorbed by osteoclasts and regenerated by osteoblasts, by which old bone is replaced by new bone. The equilibrium state of bone resorption and formation is regulated by local and systemic factors including cells, hormones, cytokines, etc. Here we focus on the relationship between bone derived macrophages and bone remodeling related cells.
Macrophage and BMSCs
Champagne is the first one to propose the mechanism by which macrophages contribute to osteogenic differentiation of bone marrow mesenchymal stem cells (BMSCs). In their study, the conditioned medium of the inactive J774A.1 mouse macrophage cell line was used for experiments, and it was found that the activity of alkaline phosphatase in human bone marrow mesenchymal stem cells was enhanced by the mediation of BMP-2 (Figure 2) (Champagne et al., 2002; Jamalpoor et al., 2018). A subsequent study co-cultured inactive human monocytes and human bone marrow mesenchymal stem cells (hbMSCs) and found that monocytes promoted MSC proliferation and increased expression of osteocalcin and osteopontin (Pirraco et al., 2013). Osteomacs regulate maintenance and proliferation of Nestin-positive MSC. These MSC express a variety of HSC retention factors and it is thought that macrophages talk to MSC via unknown secreted factors, excluding IL-1, IL-10, TNF-α, and insulin like growth factor 1 (IGF-1) (Heideveld and van den Akker, 2017). Nicolaidou et al. found that the number of macrophages in culture was positively correlated with bone formation when they co-culture human peripheral blood mononuclear cells (PBMCs) with hbMSCs (Nicolaidou et al., 2012). This process requires direct cell-cell contact to produce a soluble factor that induces STAT3 phosphorylation, known as oncostatin M (OSM). OSM is bound by two receptor complexes, consisting of a gp130 subunit and a leukemia suppressor receptor (LIFR). OSM gene deletion altered bone healing in the tibial injury model in mice. In vitro studies have described the stimulating effect of OSM produced by macrophages on the mineralization activity and differentiation of osteoblasts (Sims and Quinn, 2014). This process also depends on prostaglandin E2 (PGE2) and cox-2 (COX2) (Nicolaidou et al., 2012). Cell-cell contact between MSCs and macrophages produces PGE2 and induces OSM production through EP2/4 receptor on macrophages. Then OSM activates STAT3 phosphorylation through OSM and LIF receptors (Figure 2) (Nicolaidou et al., 2012; Horwood, 2016). Guihard also found the OSM signaling pathway. However, when hbMSCs were cultured in the conditioned medium of mononuclear cells treated with IL-4 or IL-10, there was no enhanced osteogenesis found and no OSM secretion detected (Guihard et al., 2012). Found in the study of inducing bone formation through bone Ti implants, the osteogenic differentiation of BMSCs could be changed by shifting the macrophage phenotype. Excessive polarization in the M1 direction leads to prolonged inflammation, while excessive polarization in the M2 direction leads to enhanced osteogenesis around Ti implants greatly (Wang et al., 2018). In contrast to the above studies, the effects of M0, M1, and M2 mouse bone marrow macrophages on osteogenic differentiation of mouse bone marrow mesenchymal stem cells have been studied. It was noted that all the macrophage subtypes could promote bone formation, and the M1-type macrophages had the greatest effect on bone formation (Lu et al., 2017). Studies have found that M1 macrophages increase the early and middle osteogenesis of MSCs, but do not increase matrix mineralization, while M2 macrophages co-culture can lead to increasing matrix mineralization. Moreover, it was found that the production of OSM increased in M1 culture and the production of BMP-2 increased in M2 co-culture, suggesting that different factors may be the driving factors for MSC differentiation in M1 and M2 cultures (Zhang et al., 2017).
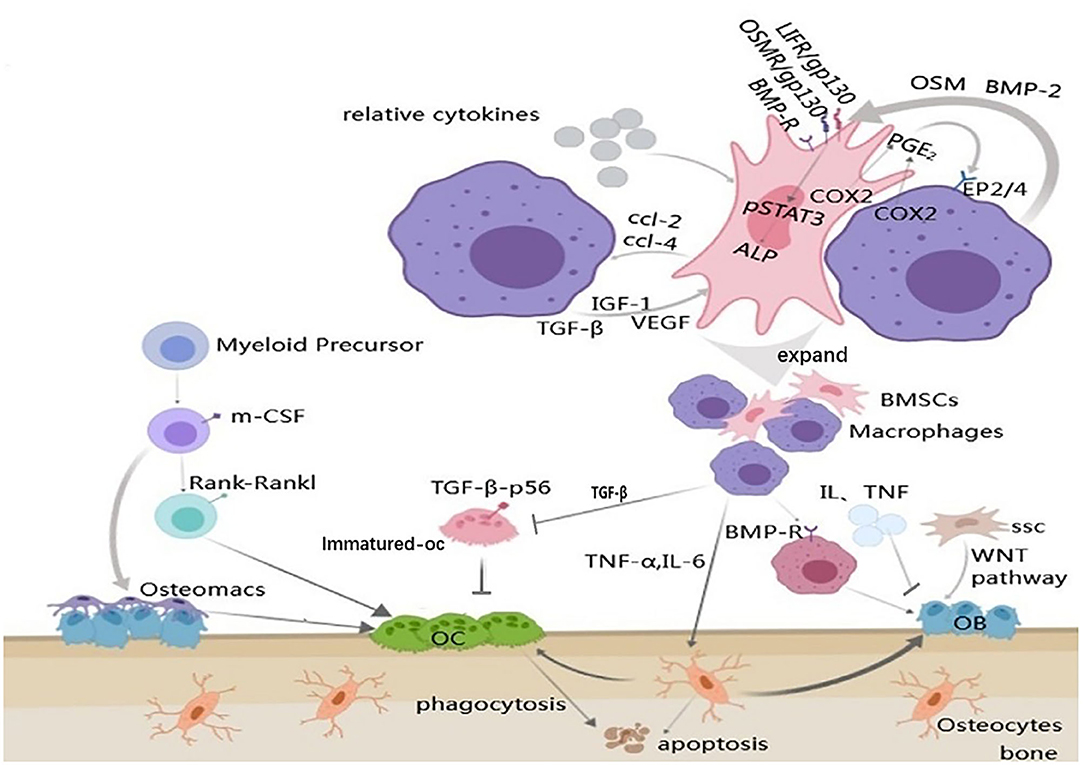
Figure 2. Communications between macrophages and bone remodeling cells. Arrow represents “stimulatory modification;” the gray triangle means “enlarged display;” the arrow with a line means “suppress.” Cell-cell communication between MSCs and macrophages results in the production of PGE2 and through the EP2/4 receptors to produce OSM. OSM acts on the MSCs via the gp130 receptors to activate STAT3 phosphorylation. Macrophage can also release BMP-2 to active the osteoblast differentiation genes, such as ALP, via the BMP-R in MSCs. MSCs can produce CCL-2 and CCL-4 to regulate macrophages and in reverse, this process is under the control of the macrophages; The differentiation of osteoblasts is regulated by variety of aspects including macrophages, osteocytes, inflammatory mediators and MSCs; Osteoclasts and osteomacs can derive from the same myeloid precursor, but it's unknown if the osteomac is a kind of the osteoclast precursors. Besides, macrophages mediate the growth of osteoclasts through various cytokines; Osteocytes apoptosis is under the regulation of macrophages.
In the co-culture model, MSCs significantly inhibited the production of LPS-induced pro-inflammatory cytokines (TNF-α, IL-1β, and IL-6) through iNOS and COX2-dependent pathways, and increased the secretion of IL-10 in macrophages by enhancing the production of PGE2 (Maggini et al., 2010). Experiments have proved that co-culture of MSCs and macrophages can significantly inhibit M1-macrophage polarization and induce M2 polarization (Kim and Hematti, 2009; Cho et al., 2014a). Human and mouse bone marrow mesenchymal stem cells secrete a large number of chemokines, including CCL-2 and CCL-4 (Seebach et al., 2014), which are the main chemokines for monocytes and macrophages (Mantovani et al., 2004). Combining INF-γ with another pro-inflammatory cytokine (TNF-β, IL-1β) activates bone marrow mesenchymal stem cells in damaged or inflammatory tissue, leading to increased secretion of various chemokines (Figure 2) (Ren et al., 2008). This MSC-mediated macrophage recruitment and macrophage phenotypic regulation may promote tissue regeneration (Bernardo and Fibbe, 2013).
Macrophage and Osteoblasts
The purest form of bone formation is intramembrane ossification, in which the bone matrix is formed and deposited directly by osteoblasts. In vivo, osteal cells occur in multiple stages of intramembranous bone healing and form a unique canopy structure on mature osteoblasts. Osteal cells are in direct contact with stromal production and mineralized osteoblasts. Moreover, osteomacs promoted osteoblast differentiation, through the OSM-mediated tyrosine phosphorylation and interaction between the STAT3 and Yes-associated protein 1 (YAP1) (Wang et al., 2020). Depletion of osteomacs significantly inhibited the formation of new bone, however, specific enlargement of osteomacs resulted in a significant increase in new mineralized substrates (Raggatt et al., 2014). In MAFIA mice (a macrophage Fas-induced apoptosis mouse model), the bone transformation rate is significantly reduced (Cho et al., 2014b). TNF-α released by activated macrophages can stimulate osteoblast chemotactic effect in vitro, and can inhibit osteoblast differentiation in rheumatoid arthritis patients (Sun et al., 2018). IL-6 inhibits osteoblast differentiation and disrupts the balance of normal bone turnover (Harmer et al., 2018). Osteoblasts are derived from a subtype of skeleton stem cells (SSC) which belongs to bone marrow mesenchymal stem cells (Fierro et al., 2017). The WNT pathway is a major pathway for the transformation of SSC into osteoblasts. Macrophage-derived BMP-2 plays a critical role in inducing ossification by inducing alkaline phosphatase production through the signaling cascade of Wnt and Wnt/LRP5 in osteoblasts (Rawadi et al., 2003; Jamalpoor et al., 2018). Treatment of macrophages with BMP-2 antibody can prevent osteogenesis (Champagne et al., 2002). The conditioned medium collected from the BMP-2-stimulated macrophages also accelerated the osteogenic differentiation of the BMSC (Wei et al., 2018). In addition, BMP-2 affects the migration, recruitment and the differentiation of macrophages (Pardali et al., 2018). In addition to WNT, the macrophage derived-BMP can bind to the BMP receptor, which causes the dimerization of BMP-R and the phosphorylation of Smad proteins. Then, the phosphorylated molecule activates Runx2 to up-regulate OB activity and differentiation (Figure 2) (Kawabata et al., 1998). In a study of xenografts about deproteinized bovine bone matrix (DBBM), it was found that IL-10 released by macrophages can promote the osteogenic response of osteoblasts induced by macrophages (Shi et al., 2018).
Macrophage and Osteoclasts
Osteal macrophages and osteoclasts are derived from myeloid progenitor cell precursors and can be stimulated by many of the same cytokines to function. But it must be emphasized that the osteomacs are not osteoclasts, because osteoclasts do not contain F4/80 Ag at all. Osteoclasts activated by pro-inflammatory stimuli may produce pre-osteoclast cytokines, including IL-6 and IL-1, which can promote the differentiation and/or function of osteoclasts. Therefore, osteoporosis may provide some candidate cellular mechanisms to explain why chronic inflammation and systemic infection often lead to osteopenia/osteoporosis (Chang et al., 2008). The generation of macrophage-derived osteoclasts can be activated by M-CSF and RANKL, and the blocking of the RANKL signaling pathway may prevent the progression of osteoporosis in mice (Jin et al., 2019). Macrophages can produce TGF-β1, which is essential for bone metabolism. NF-kB, composed of subunits such as p65, is a downstream transcription factor of the RANKL-RANKL signaling pathway (Park et al., 2017). Nuclear factor of activated T cells cytoplasmic 1 (NFATc1) is a master regulator of osteoclast differentiation (Okamoto and Takayanagi, 2011). Some studies have shown that TGF-β1 directly down-regulates NFATc1 activity by blocking the p65 in the receptor activator and then inhibits the generation and bone resorption of osteoclasts (Figure 2) (Tokunaga et al., 2020). Recently, the role of the NEMO protein (a core component of the NF-kB signaling pathway) in mouse bone marrow macrophages was investigated that if the lysine-270 (NEMO-Lys270) in NEMO protein was mutated to Ala, the NF-kB signal in bone marrow macrophages would lose control, leading to the accelerated production of osteoclasts (Adapala et al., 2020). What's more, studies have found that the overexpression of cr6 interaction factor-1 (Crif1) in mouse BMSCs can increase the secretion of RANKL through the cAMP/PKA pathway, and then combine with RANK on macrophages to promote the formation of osteoclasts in vitro (Xiang et al., 2020).
Macrophage and Osteocytes
Osteocytes account for 90–95% of all bone cells and are the most abundant and long-lived cells (Franz-Odendaal et al., 2006). Activated macrophages can induce the production of pro-inflammatory cytokines such as IL-1, IL-6, and TNF-α, which may play a significant role in inflammatory bone loss. Activated fibroblast growth factor-23 secreted by osteocytes after activation induced by IL-1 and TNF-α may cause hypophosphatemia during sepsis. TNF-α attracts osteoclasts by inducing osteocyte apoptosis (Tan et al., 2006). IL-1 may cause the decrease of bone cell activity through the NF-kB/RANKL signaling pathway, and soluble IL-6 can increase osteocyte-mediated osteoclastic differentiation by activating JAK2 and RANKL during normal bone growth and bone remodeling (Wu et al., 2017; Yang and Yang, 2019). It was reported that purified osteocytes can express a much higher amount of RANKL, which bind with mouse bone marrow macrophage and support osteoclast differentiation (Elango et al., 2018). Inflammation is thought to be highly correlated with bone cell apoptosis. If macrophages in part of the femoral head are polarized to the M1 phenotype and up-regulate a large number of inflammatory mediators, they can promote bone cell apoptosis and accelerate femoral head necrosis (Figure 2) (Jin et al., 2020).
In summary, bone formation relies on the interaction of a variety of cells which reminded above and other cytokines including HIF-1α (Karshovska et al., 2020)and CSF-1 (Alexander et al., 2011; Raggatt et al., 2014)to maintain the dynamic balance of the skeletal environment.
Macrophage-Derived Exosomes (Vesicles) Regulate Bone Metabolism
Intercellular communication is a key biological process that enables cells to coordinate their responses spatially and temporally to physiological changes. A fresh member of the intercellular communication system is the extracellular vesicle (EVs) (Théry, 2011) which is a small membrane derived phospholipid bilayer with a diameter between 30 and 2,000 nm. A vital class of EVs is exosomes (released by exocytosis, 30–150 nm diameter) (Colombo et al., 2014), has gained closely attention.
Recent studies have shown that macrophage-derived EVs play an important role in maintaining stability of the bone environment and bone remodeling (Table 1). In the study of BMP-2/macrophage-derived exosomes implantation of titanium nanotube, BMP2/macrophage-derived exosomes dramatically increased the expression of osteogenesis-related genes (ALP, osteopontin, Runx2, BMP-2, and BMP-7). Furthermore, exosome-encapsulated nanotubes activated autophagy of hBMSCs and altered the secretion of cytokines associated with bone remodeling. All of these indicate the pro-osteogenic role of the BMP-2/macrophage-derived exosomes (Wei et al., 2019). And studies have found that after tendon injury, the development of the fibrotic healing response impairs the function of the tendon and restricts the movement of the tendon. MicroRNAs are a same category of small, single-stranded non-coding RNAs discovered in diverse organisms, which can regulate the expression of mRNAs (Yang et al., 2017). Studies have found that exosome miR-21-5p secreted by bone marrow macrophages activates and promotes tendon cell fibrosis fiber formation by inhibiting the expression of Smad7 (Cui et al., 2019). After induction into M2 macrophages, murine bone marrow derived macrophages can secrete miR-21, interfere with the normal signal of the PTEN/PI3K/AKT signaling pathway, and thus regulate the biological behavior of a variety of tumors, including osteosarcoma (Figure 3) (Zheng et al., 2017; Yang et al., 2018). This finding serves as a potential target for the prevention and treatment of tendon adhesion and bone tumors. Bone marrow macrophage-derived miR-155 can be induced to release by the activators of pro-inflammatory M1 phenotype macrophages (LPS, IFN-α) and promote the secretion of inflammatory cytokines such as TNF-α, IL-12, thereby aggravating the inflammatory response in RA (Stanczyk et al., 2008), diabetes (Ying et al., 2017), and heart disease (Figure 3) (Heymans et al., 2013). The latest studies found that M2-type macrophages derived from mouse bone marrow macrophages enriched miR-5106. M2D-Exo containing miR-5106 can promote osteogenic differentiation of bone marrow mesenchymal stem cells and accelerate fracture healing in vivo by inhibiting the expression of osteogenic related genes SIK2 and SIK3 (Figure 3) (Xiong et al., 2020). Unexpectedly, compared to exosomes from M0 and M2, exosomes from M1 have a stronger stimulating effect on the proliferation, osteogenesis, and adipogenic differentiation of BMSCS and all three types of exosomes had an adverse effect on the chondrogenic differentiation of BMMSCs (Xia et al., 2020). The above findings indicate that the exact mechanisms of the macrophage-derived EVs still need to be further investigated and could be used as an effective therapeutic strategy for tissue regeneration.
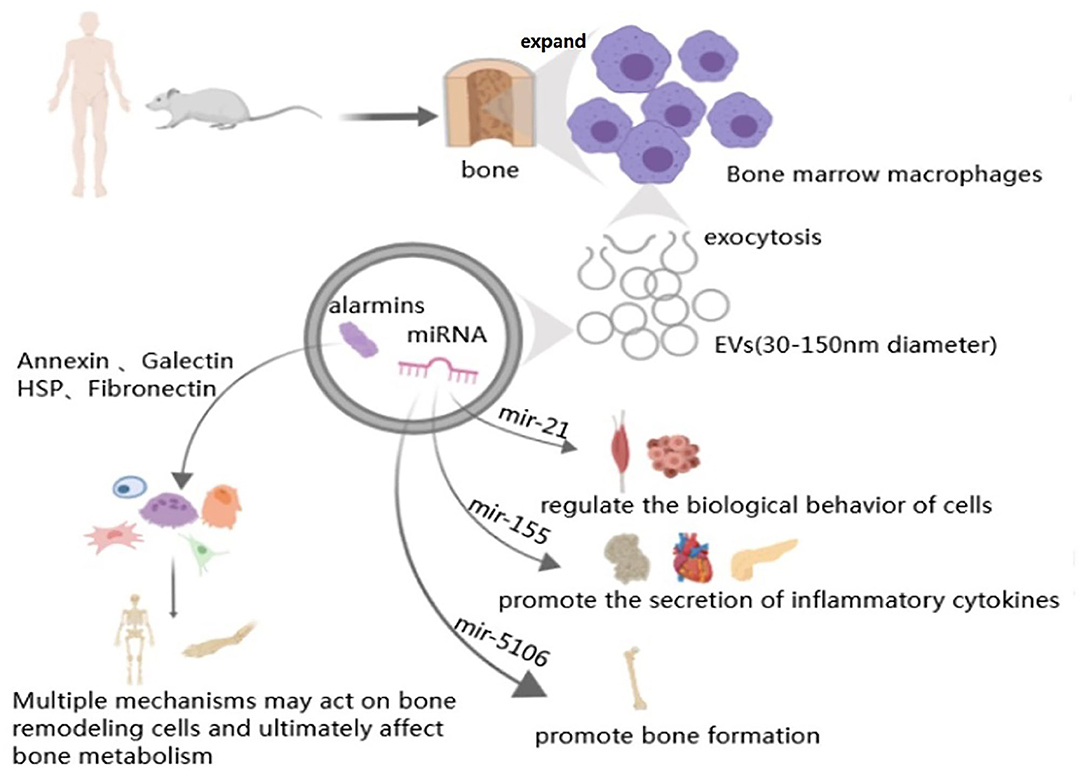
Figure 3. Macrophage-derived exosomes in bone metabolism. Arrow represents “stimulatory modification;” the gray triangle means “enlarged display.” The structure of macrophage derived exosomes is phospholipid bilayer with a diameter between 30 and 150 nm. Macrophage derived exosomes contain miRNAs (such as mir-21, mir-155, and mir-5106) and alarmins (such as Annexin, Galectin, HSP, and Fibronectin), all of them can through diverses signal pathways or release relative cytokines to participate in variety organs metabolism, including bone remodeling.
Proteomic studies have shown that macrophage-derived EVs contain a large number of alarmins (one of endogenous molecules) (Figure 3). Annexins can stimulate bone resorption (Li et al., 2005), improve the phagocytic efficiency of macrophage (Stukes et al., 2016), which is related to Multiple myeloma (MM) (D'Souza et al., 2012) and play an auxiliary role of macrophages in infected tissues (Silva et al., 2019; McArthur et al., 2020; Sanches et al., 2020). Galectins mainly affect the differentiation of bone marrow stromal cells (Andersen et al., 2003), osteoblast, osteoclast (Shimura et al., 2005; Nakajima et al., 2014; Simon et al., 2017; Iacobini et al., 2018) and the osteogenesis of mesenchymal stem cells (Weilner et al., 2016). What's more, HSP-60 (Koh et al., 2009), HSP-70 (Notsu et al., 2016; Nakamura et al., 2019) and fibronectin are also primarily concerned with osteoblasts (Moursi et al., 1996) and osteoclasts (Gramoun et al., 2010) to come into play (Table 1).
Macrophages play a critical role in bone remodeling as a source of vehicle-carried alarmins. In conclusion, future studies should be more detailed to determine the contribution of the macrophage-derived exosomes.
The body's regulatory agents are complex and poorly understood, and future efforts would be made to unravel them (Figure 4).
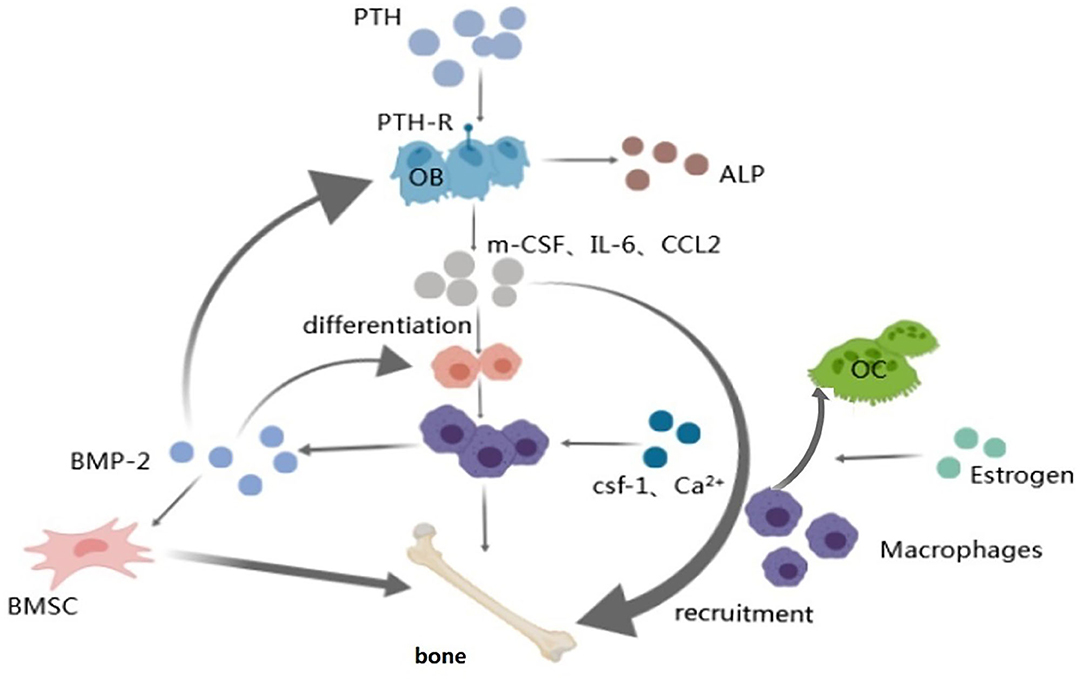
Figure 4. Macrophage and regulatory hormones and factors. Arrow represents “stimulatory modification;” the gray triangle means “enlarged display.” Macrophages play a part in the anabolic response to intermittent PTH therapy via osteoblasts which can secrete factors (IL-6, etc.) that promote the differentiation and recruitment of macrophages; Worthy to be mentioned the work of CSF-1 and Ca2+ on macrophages; Macrophage-derived BMP-2 has effect on BMSCs, osteoblasts and macrophages via BMP-R to accelerate the osteogenic differentiation so as to make bone metabolism balance; Estrogen regulates bone homeostasis mainly through osteoblasts and osteoclasts. Estrogen mainly affects the activity of macrophages by interfering with the macrophage-mediated osteoclast formation process.
Macrophage and Bone Remodeling-Related Bone Diseases
Tissue homeostasis requires a tight tissue system of various cell types to maintain. If the balance is disrupted, the progression of abnormal disease states may continue. Macrophages are involved in the development of many diseases with various phenotypes, but the direct link between macrophage function and bone-related diseases has not been thoroughly studied.
Osteoporosis
Osteoporosis is a common disease characterized by the destruction of bone mass and microstructure, resulting in brittle fractures. The characterize of age-related osteoporosis is reduced bone formation and increased marrow fat accumulation (Li et al., 2015). People with osteoporosis are under a 40% lifetime risk of fractures, most often in the spine, hip or wrist. As the aging of the population, postmenopausal osteoporosis, in particular, will further increase (Rachner et al., 2011). In addition, the 12-month mortality rate for osteoporotic hip and spinal fractures is up to 20%, and the incidence of fracture complications such as pneumonia or thromboembolic disease increases due to chronic fixation (Center et al., 1999).
Aging is usually accompanied by an irreversible recession in physiological functions. Age-associated metabolic dysfunction includes, but is not limited to, increased fat mass accumulation and insulin sensitivity deterioration (Huang et al., 2020). Aging is usually accompanied by a decrease in M2-type macrophages and an increase in proinflammatory factors, which promote the M1 transformation of the macrophage phenotype to aggravate the symptom. A study of transplanted bone marrow from 4-week-old mice into 12-month-old mice found improved fracture healing in older mice, which was attributed to younger inflammatory cells participating in the repair process (Xing et al., 2010). Cytokines secreted by macrophages related to osteoclasts including IL-1, IL-6, IL-18, IL-23, IL-27, and TNF-α may promote osteoclast differentiation and activation in an inflammatory state (Figure 5) (Yang and Yang, 2019). As mentioned, macrophages regulate osteoclasts and osteoblasts mainly through inflammatory cytokines to mediate the occurrence of osteoporosis.
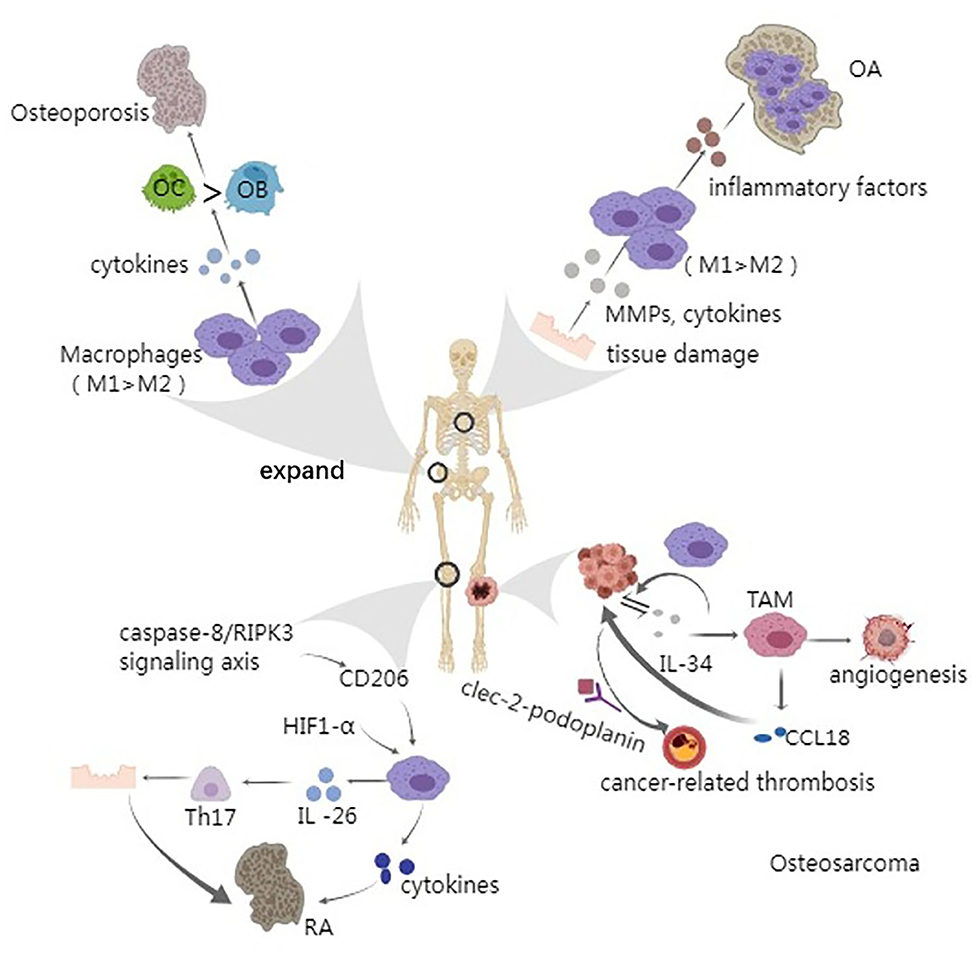
Figure 5. Macrophage and bone remodeling related diseases. Arrow represents “stimulatory modification;” the gray triangle means “enlarged display.” Osteoporosis is an age-related bone disease to some extent. The release and accumulation of proinflammatory cytokines can active osteoclasts and thus greatly accelerate the process of osteoporosis; The patients of osteoarthritis have a vital inducer that is tissue damage which can release various cytokines, leading to the imbalance between M1 and M2 macrophages. Usually, the quantity of M1 prevails, leading to the aggravation of OA; Caspase-8/RIPK3 signaling pathway and HIF1-α have been found to play a role in regulating macrophages, through CD206 and glycolysis, respectively. Besides, macrophages can secret IL-26, an inducer of Th17 cells which can cause autoimmune tissue damage; Osteosarcoma always occurs in the metaphyseal region of the long bone and the growth and metastasis of tumor cells have a closely relationship with IL-34 and CCL18. Moreover, tumor cell-derived IL-34 stimulates the angiogenesis of TAM. Cancer-related thrombosis could be made by the interaction of clec-2-podoplanin and the ectopic expression of podoplanin in patient's macrophages is the inducer, leading to the severe complications.
In addition to inflammatory response, latest studies have identified new regulatory mechanisms of macrophages in osteoporosis. They employed mice BMMs modified for over expression or inhibition of miR-128 levels to determine its effect on osteoclast differentiation. In vitro experiments showed that miR-128 may play a regulatory role in BMMs through miR-128/SIRT1/NF-kB signaling axis, and over expression or inhibition of miR-128 can significantly increase or decrease the occurrence of macrophage-derived osteoclasts, respectively (Shen et al., 2020). Further studies found that miR-506-3p can selectively inhibit NFATc1 in RANKL-induced activated rat BMMs, further reduce the release of bone resorption enzymes, thus relieving osteolysis (Dinesh et al., 2020). What's more, both the smo-GLI1/2 axis (Hh ligands permitting the activation of Hh signal transducer smoothened (SMO) and transmitting intracellular signaling through transcription factors of the GLI family)in the Hedgehog (Hh) signaling pathway (Kohara et al., 2020) and delphinidin (Imangali et al., 2020) can mediate the regulation of osteoclasts by macrophages.
The new studies provide an important basis for the treatment of senile osteoporosis by preventing osteoclast formation which derived from macrophages, but the exact mechanism of macrophages in osteoporosis has yet to be investigated.
Osteoarthritis
Osteoarthritis (Murray et al., 2014), the most common form of joint disease, is characterized by cartilage destruction, synovial fibrosis, osteophyte (osteophyte at the edge of the joint) formation and pain, and sometimes swelling of certain joints such as the knee, hip, hand, and spine (Hügle and Geurts, 2017). Depending on the world health organization (WHO), the prevalence of OA worldwide exceeds 150 million (Harrell et al., 2019). The exact mechanism of OA appears to be the result of complex interactions among mechanical, cellular, and immune factors. Synovitis has turned out to be found in the early and late stages of most patients with OA (Sellam and Berenbaum, 2010), and the accumulation of macrophages in the synovium is a feature of synovitis (Sun et al., 2016).
The high proportion of M2 macrophages in the synovial tissues has certain clinical diagnostic significance for OA (Chen et al., 2020), and the pro-inflammatory M1 macrophages are also increased significantly (Sun et al., 2017). Some studies have confirmed that macrophages play an important role in the occurrence of OA through inflammatory factors, cytokines and proteins, whether it is inflammatory or mechanical injury (Bondeson et al., 2010). Since M1 macrophages are considered to be pro-inflammatory while M2 macrophages are anti-inflammatory, the degree of imbalance between M1 and M2 macrophages in OA is related to the severity of OA (Xue et al., 2019). In OA, potential mediators (MMPs, cytokines, and growth factors due to cartilage injury) leak into synovial fluid through damaged cartilage and activate synovial macrophages. Subsequently, the proinflammatory cytokines, catabolism, and anabolic factors are released, which induce osteophyte formation (Figure 5) (Liu-Bryan, 2013). OA was induced by intra articular injection of collagenase (collagenase-induced osteoarthritis (CIOA) and surgical medial meniscus instability (DMM) and the result showed that compared with M2 macrophages, M1 polarization of synovial macrophages was increased in the CIOA and DMM circu;amodels (Zhang et al., 2018). All above studies indicate that M1 macrophages are potential therapeutic targets for OA therapy.
Rheumatoid Arthritis
Rheumatoid arthritis (RA) is a chronic immune-mediated inflammatory disease that affects nearly 1% of the world's population. It mainly affects synovial tissue, cartilage, and bone, leading to delayed joint damage, pain, swelling, and stiffness (McInnes and Schett, 2011). RA patients are at a higher risk of serious infection, respiratory disease, osteoporosis, cardiovascular disease, cancer, and death than the general population.
The exact pathogenesis of RA is unknown, but the activated macrophages play an important role in the pathogenesis. Caspase-8 is a promoter of apoptosis and an inhibitor of cell necrosis. Studies have found that the caspase-8/RIPK3 signaling axis is involved in maintaining stability of macrophages in synovial tissues, thereby limiting arthritis. The lack of caspase-8 in mouse synovium macrophage reduces the expression of CD206, resulting the inadequate of caspase-8-deficient macrophages to endocytosis of cellular debris caused by joint inflammation, thereby failing to control subsequent inflammation (Figure 5) (Dominguez et al., 2017). IL-34 is a pleiotropic cytokine and it's expression is associated with inflammatory diseases involving excessive proliferation of monocytes/macrophages (Ushach and Zlotnik, 2016). The plasma concentration of IL-34 in synovial fluid and serum is low (Nandi et al., 2012; Tian et al., 2013), but in rheumatoid arthritis, the content of IL-34 is dramatically increased, hence it's level is a useful biomarker for predicting the progression of rheumatoid arthritis (Ding et al., 2015; Liu et al., 2018; Ge et al., 2019). IL-34 and CSF-1R act in combination on mononuclear cell line (THP-1) can through a series of reactions to promote the production of helper T (Th17) cells, which is the main driver of autoimmune tissue damage, especially RA (Kuwabara et al., 2017). Additional data indicated that mononuclear/macrophage-derived IL−26 stimulation is also an important inducer of Th17 cells in RA (Figure 5) (Kragstrup et al., 2018). In addition, scientists have found that increasing the amount of anti-inflammatory macrophages by decreasing FLIP (flice-like inhibitory protein, highly expressed in RA synovial macrophages) in macrophages may be an effective treatment for suppressing inflammation (Huang et al., 2017). What's more, it is now becoming clear that metabolic pathways are the characteristic of rheumatoid arthritis and they may be the potential therapeutic targets (Sanchez-Lopez et al., 2019). Glycolysis is the preferred source of ATP under anoxic conditions (Epstein et al., 2017). Inflammatory joints are usually in a severe hypoxia environment, which results in the synovial fluid hypoxia inducing factor 1 α (HIF1-α) generation increases. The HIF1-α can inhibit the progression of glycolysis to stimulate the production of IL-1 and other pro-inflammatory cytokines by macrophages (Figure 5) (Tannahill et al., 2013), thus to accelerate the incidence of RA, increase oxidative damage and cartilage erosion (Hua and Dias, 2016).
Osteosarcoma
Osteosarcoma is the most common primary malignant bone tumor. It can occur in any bone but most seen in the metaphyseal region of the long bone, and initially presents as progressive pain and/or swelling. Risk factors are various (Harrison and Schwartz, 2017) and this tumor tend to involve the lung (Kelleher and O'Sullivan, 2017).
Both cancer cells and immune cells can secrete various interleukins such as IL-34 (Franzè et al., 2020), which can increase the recruitment of M2 macrophages and promote the growth and metastasis of osteosarcoma (Ségaliny et al., 2015). There is increasing evidences that IL-34 promotes tumorigenesis through autocrine and paracrine mechanisms. In the autocrine pathway, IL-34 interacts with the M-CSF receptor on cancer cells to activate signaling pathways, stimulate the growth, and spread of cancer cells and increase their resistance to chemotherapy drugs (Figure 5) (Baghdadi et al., 2016). In the paracrine pathway, IL-34 produced by tumor cells and/or immune cells triggers M-CSF1-R signaling in tumor-associated macrophages (TAMs), thereby promoting recruitment of TAM to the tumor area, facilitating the formation of new blood vessels and the exosmosis of immunosuppressive cells (Figure 5) (Mantovani et al., 2017). CCL-18 (c-c motif chemokine ligand 18) is an element secreted by TAM. Studies have shown that CCL-18 can be the activator of the EP300/UCA1/Wnt/hy-catenin pathway and promote the proliferation and migration of OS cells (Figure 5) (Su et al., 2019). Patients with cancer have an increased risk of thromboembolism. Platelet-activated receptor c-type lectin-like receptor 2 (clec-2) is almost specifically expressed in human platelets/megakaryocytes. The endogenous ligand podoplanin is a membrane protein. Patients with osteosarcoma may have ectopic expression of podoplanin in macrophages, and the interaction of clec-2-podoplanin stimulates the formation of cancer-related thrombosis, thus aggravating the patient's condition (Figure 5) (Suzuki-Inoue, 2019). The exact mechanism of osteosarcoma is still unknown, but recent studies on macrophages may provide new methods for clinical treatment and prevention of complications in patients with osteosarcoma.
Bisphosphonates, PTH, estrogen, or selective estrogen receptor modulators (SERMs) and calcitonin have been used to treat osteoporosis. Non-steroidal anti-inflammatory drugs are commonly used in rheumatoid. Moreover, surgical treatment is considered the first choice for the treatment of OA and osteosarcoma. But long-term use of PTH/bisphosphonates can increase the risk of severe side effects (osteosarcoma, mandibular necrosis, and atypical femoral fractures). Surgical treatment is unsuitable for the patients who cannot tolerate it. Thus, it seems that new treatments for common bone diseases are especially important in terms of conservative treatment. At present, we have known that bone tissue macrophages can communicate with a variety of cells in the bone by secreting of a series of hormones, cytokines, and exosomes. They mediate bone remodeling in various aspects and provides a new direction for clinical treatments.
Conclusions and Future Directions
Current knowledge of macrophages has been outlined, including their polarization, support for bone formation, potential role in bone biology, and regulation of bone metastasis. Osteal macrophages construct the cell canopy structure at the bone reconstruction site, anabolic cytokines promote bone formation and coordinate the coupling between osteoclasts and osteoblasts. However, the study of osteal macrophages is a relatively new research field, and many important and puzzling research problems still exist. The identification of specific markers of osteal macrophages remains to be explored, and the mice with specific Cre expression in BMMs/osteal macrophages is also expected to provide greater convenience for scientific research.
The potential role of microRNAs and protein profiles in exosomes, secreted by bone-resident macrophages, remains the critical issues. The prospect of improving patient outcomes and designing new therapeutic approaches are the eventual goals by better understanding the change of macrophages in the state of aging and figuring out the effects of the change on bone remodeling related diseases. Presently, new treatments targeting macrophages mainly focus on mediating the inflammatory response while lack the specific methods targeting the communications of bone-resident macrophages and other cells. Thus, validation of the role of macrophages in human bone disease requires further investigation. Targeting bone-resident macrophages may be a powerful tool for the treatment of bone remodeling related diseases.
Author Contributions
CL conceived the presented idea and supervised the written of this work. KC wrote the manuscript with supports from CL, XL, MH, YJ, CH, WH, JH, LL, and MY. All authors discussed the results and contributed to the final manuscript.
Funding
This work was supported by the National Key R&D Program of China (Grant No. 2019YFA0111900), the National Natural Science Foundation of China (Grants 81922017, 81873669, and 81802209), the Hunan Province Natural Science Foundation of China (Grant 2018JJ3863), the Innovation Driven Project of Central South University (Grant 20170033010017), and the Talent Plan of Xiangya Hospital in Central South University (Grants 35 and 41).
Conflict of Interest
The authors declare that the research was conducted in the absence of any commercial or financial relationships that could be construed as a potential conflict of interest.
References
Adapala, N. S., Swarnkar, G., Arra, M., Shen, J., Mbalaviele, G., Ke, K., et al. (2020). Inflammatory osteolysis is regulated by site-specific ISGylation of the scaffold protein NEMO. Elife. 9:e56095. doi: 10.7554/eLife.56095
Alexander, K. A., Chang, M. K., Maylin, E. R., Kohler, T., Müller, R., Wu, A. C., et al. (2011). Osteal macrophages promote in vivo intramembranous bone healing in a mouse tibial injury model. J. Bone Miner. Res. 26, 1517–1532. doi: 10.1002/jbmr.354
Andersen, H., Jensen, O. N., Moiseeva, E. P., and Eriksen, E. F. (2003). A proteome study of secreted prostatic factors affecting osteoblastic activity: galectin-1 is involved in differentiation of human bone marrow stromal cells. J. Bone Miner. Res. 18, 195–203. doi: 10.1359/jbmr.2003.18.2.195
Arora, S., Dev, K., Agarwal, B., Das, P., and Syed, M. A. (2018). Macrophages: their role, activation and polarization in pulmonary diseases. Immunobiology 223, 383–396. doi: 10.1016/j.imbio.2017.11.001
Assoian, R. K., Fleurdelys, B. E., Stevenson, H. C., Miller, P. J., Madtes, D. K., Raines, E. W., et al. (1987). Expression and secretion of type beta transforming growth factor by activated human macrophages. Proc. Natl. Acad. Sci. U.S.A. 84, 6020–6024. doi: 10.1073/pnas.84.17.6020
Baghdadi, M., Wada, H., Nakanishi, S., Abe, H., Han, N., Putra, W. E., et al. (2016). Chemotherapy-induced IL34 enhances immunosuppression by tumor-associated macrophages and mediates survival of chemoresistant lung cancer cells. Cancer Res. 76, 6030–6042. doi: 10.1158/0008-5472.CAN-16-1170
Batoon, L., Millard, S. M., Wullschleger, M. E., Preda, C., Wu, A. C., Kaur, S., et al. (2019). CD169+ macrophages are critical for osteoblast maintenance and promote intramembranous and endochondral ossification during bone repair. Biomaterials 196, 51–66. doi: 10.1016/j.biomaterials.2017.10.033
Bernardo, M. E., and Fibbe, W. E. (2013). Mesenchymal stromal cells: sensors and switchers of inflammation. Cell Stem Cell 13, 392–402. doi: 10.1016/j.stem.2013.09.006
Biswas, S. K., and Mantovani, A. (2010). Macrophage plasticity and interaction with lymphocyte subsets: cancer as a paradigm. Nat. Immunol. 11, 889–896. doi: 10.1038/ni.1937
Bondeson, J., Blom, A. B., Wainwright, S., Hughes, C., Caterson, B., and van den Berg, W. B. (2010). The role of synovial macrophages and macrophage-produced mediators in driving inflammatory and destructive responses in osteoarthritis. Arthritis Rheum. 62, 647–657. doi: 10.1002/art.27290
Center, J. R., Nguyen, T. V., Schneider, D., Sambrook, P. N., and Eisman, J. A. (1999). Mortality after all major types of osteoporotic fracture in men and women: an observational study. Lancet 353, 878–882. doi: 10.1016/S0140-6736(98)09075-8
Champagne, C. M., Takebe, J., Offenbacher, S., and Cooper, L. F. (2002). Macrophage cell lines produce osteoinductive signals that include bone morphogenetic protein-2. Bone 30, 26–31. doi: 10.1016/S8756-3282(01)00638-X
Chang, M. K., Raggatt, L. J., Alexander, K. A., Kuliwaba, J. S., Fazzalari, N. L., Schroder, K., et al. (2008). Osteal tissue macrophages are intercalated throughout human and mouse bone lining tissues and regulate osteoblast function in vitro and in vivo. J. Immunol. 181, 1232–1244. doi: 10.4049/jimmunol.181.2.1232
Chen, Z., Ma, Y., Li, X., Deng, Z., Zheng, M., and Zheng, Q. (2020). The immune cell landscape in different anatomical structures of knee in osteoarthritis: a gene expression-based study. Biomed. Res. Int. 2020:9647072. doi: 10.1155/2020/9647072
Cho, D. I., Kim, M. R., Jeong, H. Y., Jeong, H. C., Jeong, M. H., Yoon, S. H., et al. (2014a). Mesenchymal stem cells reciprocally regulate the M1/M2 balance in mouse bone marrow-derived macrophages. Exp. Mol. Med. 46:e70. doi: 10.1038/emm.2013.135
Cho, S. W., Soki, F. N., Koh, A. J., Eber, M. R., Entezami, P., Park, S. I., et al. (2014b). Osteal macrophages support physiologic skeletal remodeling and anabolic actions of parathyroid hormone in bone. Proc. Natl. Acad. Sci. U.S.A. 111, 1545–1550. doi: 10.1073/pnas.1315153111
Colombo, M., Raposo, G., and Théry, C. (2014). Biogenesis, secretion, and intercellular interactions of exosomes and other extracellular vesicles. Annu. Rev. Cell Dev. Biol. 30, 255–289. doi: 10.1146/annurev-cellbio-101512-122326
Cui, H., He, Y., Chen, S., Zhang, D., Yu, Y., and Fan, C. (2019). Macrophage-derived miRNA-containing exosomes induce peritendinous fibrosis after tendon injury through the miR-21-5p/Smad7 pathway. Mol. Ther. Nucl. Acids 14, 114–130. doi: 10.1016/j.omtn.2018.11.006
Das, A., Ganesh, K., Khanna, S., Sen, C. K., and Roy, S. (2014). Engulfment of apoptotic cells by macrophages: a role of microRNA-21 in the resolution of wound inflammation. J. Immunol. 192, 1120–1129. doi: 10.4049/jimmunol.1300613
Das, A., Sinha, M., Datta, S., Abas, M., Chaffee, S., Sen, C. K., and Roy, S. (2015). Monocyte and macrophage plasticity in tissue repair and regeneration. Am. J. Pathol. 185, 2596–2606. doi: 10.1016/j.ajpath.2015.06.001
Datta, H. K., Ng, W. F., Walker, J. A., Tuck, S. P., and Varanasi, S. S. (2008). The cell biology of bone metabolism. J. Clin. Pathol. 61, 577–587. doi: 10.1136/jcp.2007.048868
Dinesh, P., Kalaiselvan, S., Sujitha, S., and Rasool, M. (2020). miR-506-3p alleviates uncontrolled osteoclastogenesis via repression of RANKL/NFATc1 signaling pathway. J. Cell. Physiol. 235, 9497–9509. doi: 10.1002/jcp.29757
Ding, R., Li, P., Song, D., Zhang, X., and Bi, L. (2015). Predictors of response to TNF-α antagonist therapy in Chinese rheumatoid arthritis. Clin. Rheumatol. 34, 1203–1210. doi: 10.1007/s10067-015-2973-3
Dominguez, S., Montgomery, A. B., Haines, G. K. 3rd, Bloomfield, C. L., and Cuda, C. M. (2017). The caspase-8/RIPK3 signaling axis in antigen presenting cells controls the inflammatory arthritic response. Arthritis Res. Ther. 19:224. doi: 10.1186/s13075-017-1436-4
D'Souza, S., Kurihara, N., Shiozawa, Y., Joseph, J., Taichman, R., Galson, D. L., et al. (2012). Annexin II interactions with the annexin II receptor enhance multiple myeloma cell adhesion and growth in the bone marrow microenvironment. Blood 119, 1888–1896. doi: 10.1182/blood-2011-11-393348
Elango, J., Sanchez, C., de Val, J., Henrotin, Y., Wang, S., Motaung, K., et al. (2018). Cross-talk between primary osteocytes and bone marrow macrophages for osteoclastogenesis upon collagen treatment. Sci. Rep. 8:5318. doi: 10.1038/s41598-018-23532-x
Epstein, T., Gatenby, R. A., and Brown, J. S. (2017). The Warburg effect as an adaptation of cancer cells to rapid fluctuations in energy demand. PLoS ONE 12:e0185085. doi: 10.1371/journal.pone.0185085
Fierro, F. A., Nolta, J. A., and Adamopoulos, I. E. (2017). Concise review: stem cells in osteoimmunology. Stem Cells 35, 1461–1467. doi: 10.1002/stem.2625
Franzè, E., Stolfi, C., Troncone, E., Scarozza, P., and Monteleone, G. (2020). Role of interleukin-34 in cancer. Cancers 12:252. doi: 10.3390/cancers12010252
Franz-Odendaal, T. A., Hall, B. K., and Witten, P. E. (2006). Buried alive: how osteoblasts become osteocytes. Dev. Dyn. 235, 176–190. doi: 10.1002/dvdy.20603
Ge, Y., Huang, M., and Yao, Y. M. (2019). Immunomodulation of interleukin-34 and its potential significance as a disease biomarker and therapeutic target. Int. J. Biol. Sci. 15, 1835–1845. doi: 10.7150/ijbs.35070
Geissmann, F., Manz, M. G., Jung, S., Sieweke, M. H., Merad, M., and Ley, K. (2010). Development of monocytes, macrophages, and dendritic cells. Science 327, 656–661. doi: 10.1126/science.1178331
Genin, M., Clement, F., Fattaccioli, A., Raes, M., and Michiels, C. (2015). M1 and M2 macrophages derived from THP-1 cells differentially modulate the response of cancer cells to etoposide. BMC Cancer 15:577. doi: 10.1186/s12885-015-1546-9
Gordon, S. (2008). Elie Metchnikoff: father of natural immunity. Eur. J. Immunol. 38, 3257–3264. doi: 10.1002/eji.200838855
Gramoun, A., Azizi, N., Sodek, J., Heersche, J. N., Nakchbandi, I., and Manolson, M. F. (2010). Fibronectin inhibits osteoclastogenesis while enhancing osteoclast activity via nitric oxide and interleukin-1β-mediated signaling pathways. J. Cell. Biochem. 111, 1020–1034. doi: 10.1002/jcb.22791
Guihard, P., Danger, Y., Brounais, B., David, E., Brion, R., Delecrin, J., et al. (2012). Induction of osteogenesis in mesenchymal stem cells by activated monocytes/macrophages depends on oncostatin M signaling. Stem Cells. 30, 762–772. doi: 10.1002/stem.1040
Guo, Y., Cao, W., and Zhu, Y. (2019). Immunoregulatory functions of the IL-12 family of cytokines in antiviral systems. Viruses 11:772. doi: 10.3390/v11090772
Harmer, D., Falank, C., and Reagan, M. R. (2018). Interleukin-6 interweaves the bone marrow microenvironment, bone loss, and multiple myeloma. Front. Endocrinol. 9:788. doi: 10.3389/fendo.2018.00788
Harrell, C. R., Markovic, B. S., Fellabaum, C., Arsenijevic, A., and Volarevic, V. (2019). Mesenchymal stem cell-based therapy of osteoarthritis: current knowledge and future perspectives. Biomed. Pharmacother. 109, 2318–2326. doi: 10.1016/j.biopha.2018.11.099
Harrison, D. J., and Schwartz, C. L. (2017). Osteogenic sarcoma: systemic chemotherapy options for localized disease. Curr. Treat. Options Oncol. 18:24. doi: 10.1007/s11864-017-0464-2
Heideveld, E., and van den Akker, E. (2017). Digesting the role of bone marrow macrophages on hematopoiesis. Immunobiology 222, 814–822. doi: 10.1016/j.imbio.2016.11.007
Heymans, S., Corsten, M. F., Verhesen, W., Carai, P., van Leeuwen, R. E., Custers, K., et al. (2013). Macrophage microRNA-155 promotes cardiac hypertrophy and failure. Circulation 128, 1420–1432. doi: 10.1161/CIRCULATIONAHA.112.001357
Horwood, N. J. (2016). Macrophage polarization and bone formation: a review. Clin. Rev. Allergy Immunol. 51, 79–86. doi: 10.1007/s12016-015-8519-2
Hua, S., and Dias, T. H. (2016). Hypoxia-inducible factor (HIF) as a target for novel therapies in rheumatoid arthritis. Front. Pharmacol. 7:184. doi: 10.3389/fphar.2016.00184
Huang, Q. Q., Birkett, R., Doyle, R. E., Haines, G. K., Perlman, H., Shi, B., et al. (2017). Association of increased F4/80(high) macrophages with suppression of serum-transfer arthritis in mice with reduced FLIP in myeloid cells. Arthritis Rheumatol. 69, 1762–1771. doi: 10.1002/art.40151
Huang, Y., Xiao, Y., Liu, Y., Guo, M., Guo, Q., Zhou, F., et al. (2020). MicroRNA-188 regulates aging-associated metabolic phenotype. Aging Cell. 19:e13077. doi: 10.1111/acel.13077
Hügle, T., and Geurts, J. (2017). What drives osteoarthritis?-synovial versus subchondral bone pathology. Rheumatology 56, 1461–1471. doi: 10.1093/rheumatology/kew389
Iacobini, C., Blasetti Fantauzzi, C., Bedini, R., Pecci, R., Bartolazzi, A., Amadio, B., et al. (2018). Galectin-3 is essential for proper bone cell differentiation and activity, bone remodeling and biomechanical competence in mice. Metab. Clin. Exp. 83, 149–158. doi: 10.1016/j.metabol.2018.02.001
Imangali, N., Phan, Q. T., Mahady, G., and Winkler, C. (2020). The dietary anthocyanin delphinidin prevents bone resorption by inhibiting Rankl-induced differentiation of osteoclasts in a medaka (Oryzias latipes) model of osteoporosis. J. Fish Biol. doi: 10.1111/jfb.14317. [Epub ahead of print].
Jafarnezhad-Ansariha, F., Yekaninejad, M. S., Jamshidi, A. R., Mansouri, R., Vojdanian, M., Mahmoudi, M., et al. (2018). The effects of β-D-mannuronic acid (M2000), as a novel NSAID, on COX1 and COX2 activities and gene expression in ankylosing spondylitis patients and the murine monocyte/macrophage, J774 cell line. Inflammopharmacology 26, 375–384. doi: 10.1007/s10787-017-0386-4
Jamalpoor, Z., Asgari, A., Lashkari, M. H., Mirshafiey, A., and Mohsenzadegan, M. (2018). Modulation of macrophage polarization for bone tissue engineering applications. Iran. J. Allergy Asthma Immunol. 17, 398–408. doi: 10.18502/ijaai.v17i5.298
Jetten, N., Verbruggen, S., Gijbels, M. J., Post, M. J., De Winther, M. P., and Donners, M. M. (2014). Anti-inflammatory M2, but not pro-inflammatory M1 macrophages promote angiogenesis in vivo. Angiogenesis 17, 109–118. doi: 10.1007/s10456-013-9381-6
Jin, H., Yao, L., Chen, K., Liu, Y., Wang, Q., Wang, Z., et al. (2019). Evodiamine inhibits RANKL-induced osteoclastogenesis and prevents ovariectomy-induced bone loss in mice. J. Cell. Mol. Med. 23, 522–534. doi: 10.1111/jcmm.13955
Jin, S., Meng, C., He, Y., Wang, X., Zhang, Q., Wang, Z., et al. (2020). Curcumin prevents osteocyte apoptosis by inhibiting M1-type macrophage polarization in mice model of glucocorticoid-associated osteonecrosis of the femoral head. J. Orthop. Res. 38, 2020–2030. doi: 10.1002/jor.24619
Juhas, M., Abutaleb, N., Wang, J. T., Ye, J., Shaikh, Z., Sriworarat, C., Qian, Y., and Bursac, N. (2018). Incorporation of macrophages into engineered skeletal muscle enables enhanced muscle regeneration. Nat. Biomed. Eng. 2, 942–954. doi: 10.1038/s41551-018-0290-2
Karshovska, E., Wei, Y., Subramanian, P., Mohibullah, R., Geißler, C., Baatsch, I., et al. (2020). HIF-1α (hypoxia-inducible factor-1α) promotes macrophage necroptosis by regulating miR-210 and miR-383. Arterioscler. Thromb. Vasc. Biol. 40, 583–596. doi: 10.1161/ATVBAHA.119.313290
Kaur, S., Raggatt, L. J., Batoon, L., Hume, D. A., Levesque, J. P., and Pettit, A. R. (2017). Role of bone marrow macrophages in controlling homeostasis and repair in bone and bone marrow niches. Semin. Cell Dev. Biol. 61, 12–21. doi: 10.1016/j.semcdb.2016.08.009
Kawabata, M., Imamura, T., and Miyazono, K. (1998). Signal transduction by bone morphogenetic proteins. Cytokine Growth Factor Rev. 9, 49–61. doi: 10.1016/S1359-6101(97)00036-1
Kelleher, F. C., and O'Sullivan, H. (2017). Monocytes, macrophages, and osteoclasts in osteosarcoma. J. Adolesc. Young Adult Oncol. 6, 396–405. doi: 10.1089/jayao.2016.0078
Kim, J., and Hematti, P. (2009). Mesenchymal stem cell-educated macrophages: a novel type of alternatively activated macrophages. Exp. Hematol. 37, 1445–1453. doi: 10.1016/j.exphem.2009.09.004
Koh, J. M., Lee, Y. S., Kim, Y. S., Park, S. H., Lee, S. H., Kim, H. H., et al. (2009). Heat shock protein 60 causes osteoclastic bone resorption via toll-like receptor-2 in estrogen deficiency. Bone 45, 650–660. doi: 10.1016/j.bone.2009.06.007
Kohara, Y., Haraguchi, R., Kitazawa, R., Imai, Y., and Kitazawa, S. (2020). Hedgehog inhibitors suppress osteoclastogenesis in in vitro cultures, and deletion of smo in macrophage/osteoclast lineage prevents age-related bone loss. Int. J. Mol. Sci. 21:2745. doi: 10.3390/ijms21082745
Kragstrup, T. W., Andersen, T., Heftdal, L. D., Hvid, M., Gerwien, J., Sivakumar, P., et al. (2018). The IL-20 cytokine family in rheumatoid arthritis and spondyloarthritis. Front. Immunol. 9:2226. doi: 10.3389/fimmu.2018.02226
Kreutz, M., Andreesen, R., Krause, S. W., Szabo, A., Ritz, E., and Reichel, H. (1993). 1,25-dihydroxyvitamin D3 production and vitamin D3 receptor expression are developmentally regulated during differentiation of human monocytes into macrophages. Blood 82, 1300–1307. doi: 10.1182/blood.V82.4.1300.1300
Kuwabara, T., Ishikawa, F., Kondo, M., and Kakiuchi, T. (2017). The role of IL-17 and related cytokines in inflammatory autoimmune diseases. Mediators Inflamm. 2017:3908061. doi: 10.1155/2017/3908061
Le, B. Q., Nurcombe, V., Cool, S. M., van Blitterswijk, C. A., de Boer, J., and LaPointe, V. L. S. (2017). The components of bone and what they can teach us about regeneration. Materials 11:14. doi: 10.3390/ma11010014
Li, C. J., Cheng, P., Liang, M. K., Chen, Y. S., Lu, Q., Wang, J. Y., et al. (2015). MicroRNA-188 regulates age-related switch between osteoblast and adipocyte differentiation. J. Clin. Invest. 125, 1509–1522. doi: 10.1172/JCI77716
Li, C. J., Xiao, Y., Yang, M., Su, T., Sun, X., Guo, Q., et al. (2018). Long noncoding RNA Bmncr regulates mesenchymal stem cell fate during skeletal aging. J. Clin. Invest. 128, 5251–5266. doi: 10.1172/JCI99044
Li, F., Chung, H., Reddy, S. V., Lu, G., Kurihara, N., Zhao, A. Z., et al. (2005). Annexin II stimulates RANKL expression through MAPK. J. Bone Miner. Res. 20, 1161–1167. doi: 10.1359/JBMR.050207
Liu, Q., Fan, J., Bai, J., Peng, L., Zhang, T., Deng, L., et al. (2018). IL-34 promotes foam cell formation by enhancing CD36 expression through p38 MAPK pathway. Sci. Rep. 8:17347. doi: 10.1038/s41598-018-35485-2
Liu-Bryan, R. (2013). Synovium and the innate inflammatory network in osteoarthritis progression. Curr. Rheumatol. Rep. 15:323. doi: 10.1007/s11926-013-0323-5
Lu, L. Y., Loi, F., Nathan, K., Lin, T. H., Pajarinen, J., Gibon, E., et al. (2017). Pro-inflammatory M1 macrophages promote osteogenesis by mesenchymal stem cells via the COX-2-prostaglandin E2 pathway. J. Orthop. Res. 35, 2378–2385. doi: 10.1002/jor.23553
Maggini, J., Mirkin, G., Bognanni, I., Holmberg, J., Piazzón, I. M., Nepomnaschy, I., et al. (2010). Mouse bone marrow-derived mesenchymal stromal cells turn activated macrophages into a regulatory-like profile. PLoS ONE 5:e9252. doi: 10.1371/journal.pone.0009252
Mantovani, A., Marchesi, F., Malesci, A., Laghi, L., and Allavena, P. (2017). Tumour-associated macrophages as treatment targets in oncology. Nat. Rev. Clin. Oncol. 14, 399–416. doi: 10.1038/nrclinonc.2016.217
Mantovani, A., Sica, A., Sozzani, S., Allavena, P., Vecchi, A., and Locati, M. (2004). The chemokine system in diverse forms of macrophage activation and polarization. Trends Immunol. 25, 677–686. doi: 10.1016/j.it.2004.09.015
McArthur, S., Juban, G., Gobbetti, T., Desgeorges, T., Theret, M., Gondin, J., et al. (2020). Annexin A1 drives macrophage skewing to accelerate muscle regeneration through AMPK activation. J. Clin. Invest. 130, 1156–1167. doi: 10.1172/JCI124635
McInnes, I. B., and Schett, G. (2011). The pathogenesis of rheumatoid arthritis. N. Engl. J. Med. 365, 2205–2219. doi: 10.1056/NEJMra1004965
Michalski, M. N., and McCauley, L. K. (2017). Macrophages and skeletal health. Pharmacol. Ther. 174, 43–54. doi: 10.1016/j.pharmthera.2017.02.017
Miron, R. J., Zohdi, H., Fujioka-Kobayashi, M., and Bosshardt, D. D. (2016). Giant cells around bone biomaterials: osteoclasts or multi-nucleated giant cells? Acta Biomater. 46, 15–28. doi: 10.1016/j.actbio.2016.09.029
Mohsenzadegan, M., Fayazi, M. R., Abdolmaleki, M., Bakhshayesh, M., Seif, F., and Mousavizadeh, K. (2015). Direct immunomodulatory influence of IFN-β on human astrocytoma cells. Immunopharmacol. Immunotoxicol. 37, 214–219. doi: 10.3109/08923973.2015.1014559
Mosser, D. M. (2003). The many faces of macrophage activation. J. Leukoc. Biol. 73, 209–212. doi: 10.1189/jlb.0602325
Mosser, D. M., and Edwards, J. P. (2008). Exploring the full spectrum of macrophage activation. Nat. Rev. Immunol. 8, 958–969. doi: 10.1038/nri2448
Moursi, A. M., Damsky, C. H., Lull, J., Zimmerman, D., Doty, S. B., Aota, S., et al. (1996). Fibronectin regulates calvarial osteoblast differentiation. J. Cell Sci. 109, 1369–1380.
Murray, P. J., Allen, J. E., Biswas, S. K., Fisher, E. A., Gilroy, D. W., Goerdt, S., et al. (2014). Macrophage activation and polarization: nomenclature and experimental guidelines. Immunity 41, 14–20. doi: 10.1016/j.immuni.2014.06.008
Nakajima, K., Kho, D. H., Yanagawa, T., Harazono, Y., Gao, X., Hogan, V., et al. (2014). Galectin-3 inhibits osteoblast differentiation through notch signaling. Neoplasia 16, 939–949. doi: 10.1016/j.neo.2014.09.005
Nakamura, M., Notsu, K., and Nakagawa, M. (2019). Heat shock protein 60 negatively regulates the biological functions of ubiquitin-like protein MNSFβ in macrophages. Mol. Cell. Biochem. 456, 29–39. doi: 10.1007/s11010-018-3487-5
Nandi, S., Gokhan, S., Dai, X. M., Wei, S., Enikolopov, G., Lin, H., et al. (2012). The CSF-1 receptor ligands IL-34 and CSF-1 exhibit distinct developmental brain expression patterns and regulate neural progenitor cell maintenance and maturation. Dev. Biol. 367, 100–113. doi: 10.1016/j.ydbio.2012.03.026
Nicolaidou, V., Wong, M. M., Redpath, A. N., Ersek, A., Baban, D. F., Williams, L. M., et al. (2012). Monocytes induce STAT3 activation in human mesenchymal stem cells to promote osteoblast formation. PLoS ONE 7:e39871. doi: 10.1371/journal.pone.0039871
Notsu, K., Nakagawa, M., and Nakamura, M. (2016). Ubiquitin-like protein MNSFβ noncovalently binds to molecular chaperone HSPA8 and regulates osteoclastogenesis. Mol. Cell. Biochem. 421, 149–156. doi: 10.1007/s11010-016-2795-x
Ogle, M. E., Segar, C. E., Sridhar, S., and Botchwey, E. A. (2016). Monocytes and macrophages in tissue repair: implications for immunoregenerative biomaterial design. Exp. Biol. Med. 241, 1084–1097. doi: 10.1177/1535370216650293
Okamoto, K., and Takayanagi, H. (2011). Regulation of bone by the adaptive immune system in arthritis. Arthritis Res. Ther. 13:219. doi: 10.1186/ar3323
Pardali, E., Makowski, L. M., Leffers, M., Borgscheiper, A., and Waltenberger, J. (2018). BMP-2 induces human mononuclear cell chemotaxis and adhesion and modulates monocyte-to-macrophage differentiation. J. Cell. Mol. Med. 22, 5429–5438. doi: 10.1111/jcmm.13814
Park, J. H., Lee, N. K., and Lee, S. Y. (2017). Current understanding of RANK signaling in osteoclast differentiation and maturation. Mol. Cells 40, 706–713. doi: 10.14348/molcells.2017.0225
Pirraco, R. P., Reis, R. L., and Marques, A. P. (2013). Effect of monocytes/macrophages on the early osteogenic differentiation of hBMSCs. J. Tissue Eng. Regen. Med. 7, 392–400. doi: 10.1002/term.535
Rachner, T. D., Khosla, S., and Hofbauer, L. C. (2011). Osteoporosis: now and the future. Lancet 377, 1276–1287. doi: 10.1016/S0140-6736(10)62349-5
Raggatt, L. J., Wullschleger, M. E., Alexander, K. A., Wu, A. C., Millard, S. M., Kaur, S., et al. (2014). Fracture healing via periosteal callus formation requires macrophages for both initiation and progression of early endochondral ossification. Am. J. Pathol. 184, 3192–3204. doi: 10.1016/j.ajpath.2014.08.017
Rawadi, G., Vayssière, B., Dunn, F., Baron, R., and Roman-Roman, S. (2003). BMP-2 controls alkaline phosphatase expression and osteoblast mineralization by a Wnt autocrine loop. J. Bone Miner. Res. 18, 1842–1853. doi: 10.1359/jbmr.2003.18.10.1842
Ren, G., Zhang, L., Zhao, X., Xu, G., Zhang, Y., Roberts, A. I., et al. (2008). Mesenchymal stem cell-mediated immunosuppression occurs via concerted action of chemokines and nitric oxide. Cell Stem Cell 2, 141–150. doi: 10.1016/j.stem.2007.11.014
Robling, A. G., Castillo, A. B., and Turner, C. H. (2006). Biomechanical and molecular regulation of bone remodeling. Annu. Rev. Biomed. Eng. 8, 455–498. doi: 10.1146/annurev.bioeng.8.061505.095721
Roszer, T. (2015). Understanding the mysterious M2 macrophage through activation markers and effector mechanisms. Mediators Inflamm. 2015:816460. doi: 10.1155/2015/816460
Sanches, J. M., Branco, L. M., Duarte, G. H. B., Oliani, S. M., Bortoluci, K. R., Moreira, V., et al. (2020). Annexin A1 regulates NLRP3 inflammasome activation and modifies lipid release profile in isolated peritoneal macrophages. Cells 9:926. doi: 10.3390/cells9040926
Sanchez-Lopez, E., Cheng, A., and Guma, M. (2019). Can metabolic pathways be therapeutic targets in rheumatoid arthritis? J. Clin. Med. 8:753. doi: 10.3390/jcm8050753
Seebach, E., Freischmidt, H., Holschbach, J., Fellenberg, J., and Richter, W. (2014). Mesenchymal stroma cells trigger early attraction of M1 macrophages and endothelial cells into fibrin hydrogels, stimulating long bone healing without long-term engraftment. Acta Biomater. 10, 4730–4741. doi: 10.1016/j.actbio.2014.07.017
Ségaliny, A. I., Mohamadi, A., Dizier, B., Lokajczyk, A., Brion, R., Lanel, R., et al. (2015). Interleukin-34 promotes tumor progression and metastatic process in osteosarcoma through induction of angiogenesis and macrophage recruitment. Int. J. Cancer 137, 73–85. doi: 10.1002/ijc.29376
Sellam, J., and Berenbaum, F. (2010). The role of synovitis in pathophysiology and clinical symptoms of osteoarthritis. Nat. Rev. Rheumatol. 6, 625–635. doi: 10.1038/nrrheum.2010.159
Shen, G., Ren, H., Shang, Q., Zhang, Z., Zhao, W., Yu, X., et al. (2020). miR-128 plays a critical role in murine osteoclastogenesis and estrogen deficiency-induced bone loss. Theranostics 10, 4334–4348. doi: 10.7150/thno.42982
Shi, M., Wang, C., Wang, Y., Tang, C., Miron, R. J., and Zhang, Y. (2018). Deproteinized bovine bone matrix induces osteoblast differentiation via macrophage polarization. J. Biomed. Mater. Res. A 106, 1236–1246. doi: 10.1002/jbm.a.36321
Shimura, T., Takenaka, Y., Fukumori, T., Tsutsumi, S., Okada, K., Hogan, V., et al. (2005). Implication of galectin-3 in Wnt signaling. Cancer Res. 65, 3535–3537. doi: 10.1158/0008-5472.CAN-05-0104
Silva, J. M. D., Silva, H., Zelenski, C., Souza, J. A. M., Hueb, M., and Damazo, A. S. (2019). Analysis of macrophage subtypes and annexin A1 expression in lesions of patients with cutaneous leishmaniasis. Rev. Soc. Bras. Med. Trop. 52:e20190361. doi: 10.1590/0037-8682-0361-2019
Simon, D., Derer, A., Andes, F. T., Lezuo, P., Bozec, A., Schett, G., et al. (2017). Galectin-3 as a novel regulator of osteoblast-osteoclast interaction and bone homeostasis. Bone 105, 35–41. doi: 10.1016/j.bone.2017.08.013
Sims, N. A., and Quinn, J. M. (2014). Osteoimmunology: oncostatin M as a pleiotropic regulator of bone formation and resorption in health and disease. Bonekey Rep. 3:527. doi: 10.1038/bonekey.2014.22
Stanczyk, J., Pedrioli, D. M., Brentano, F., Sanchez-Pernaute, O., Kolling, C., Gay, R. E., et al. (2008). Altered expression of MicroRNA in synovial fibroblasts and synovial tissue in rheumatoid arthritis. Arthritis Rheum. 58, 1001–1009. doi: 10.1002/art.23386
Stukes, S., Coelho, C., Rivera, J., Jedlicka, A. E., Hajjar, K. A., and Casadevall, I. (2016). The membrane phospholipid binding protein annexin A2 promotes phagocytosis and nonlytic exocytosis of cryptococcus neoformans and impacts survival in fungal infection. J Immunol. 197, 1252–61. doi: 10.4049/jimmunol.1501855
Su, Y., Zhou, Y., Sun, Y. J., Wang, Y. L., Yin, J. Y., Huang, Y. J., et al. (2019). Macrophage-derived CCL18 promotes osteosarcoma proliferation and migration by upregulating the expression of UCA1. J. Mol. Med. 97, 49–61. doi: 10.1007/s00109-018-1711-0
Sun, A. R., Friis, T., Sekar, S., Crawford, R., Xiao, Y., and Prasadam, I. (2016). Is synovial macrophage activation the inflammatory link between obesity and osteoarthritis? Curr. Rheumatol. Rep. 18:57. doi: 10.1007/s11926-016-0605-9
Sun, A. R., Panchal, S. K., Friis, T., Sekar, S., Crawford, R., Brown, L., et al. (2017). Obesity-associated metabolic syndrome spontaneously induces infiltration of pro-inflammatory macrophage in synovium and promotes osteoarthritis. PLoS ONE 12:e0183693. doi: 10.1371/journal.pone.0183693
Sun, W., Meednu, N., Rosenberg, A., Rangel-Moreno, J., Wang, V., Glanzman, J., et al. (2018). B cells inhibit bone formation in rheumatoid arthritis by suppressing osteoblast differentiation. Nat. Commun. 9:5127. doi: 10.1038/s41467-018-07626-8
Suzuki-Inoue, K. (2019). Platelets and cancer-associated thrombosis: focusing on the platelet activation receptor CLEC-2 and podoplanin. Blood 134, 1912–1918. doi: 10.1182/blood.2019001388
Takahashi, F., Takahashi, K., Shimizu, K., Cui, R., Tada, N., Takahashi, H., et al. (2004). Osteopontin is strongly expressed by alveolar macrophages in the lungs of acute respiratory distress syndrome. Lung 182, 173–185. doi: 10.1007/s00408-004-0309-1
Tan, S. D., Kuijpers-Jagtman, A. M., Semeins, C. M., Bronckers, A. L., Maltha, J. C., Von den Hoff, J. W., et al. (2006). Fluid shear stress inhibits TNFalpha-induced osteocyte apoptosis. J. Dent. Res. 85, 905–909. doi: 10.1177/154405910608501006
Tannahill, G. M., Curtis, A. M., Adamik, J., Palsson-McDermott, E. M., McGettrick, A. F., Goel, G., et al. (2013). Succinate is an inflammatory signal that induces IL-1β through HIF-1α. Nature 496, 238–242. doi: 10.1038/nature11986
Théry, C. (2011). Exosomes: secreted vesicles and intercellular communications. F1000 Biol. Rep. 3:15. doi: 10.3410/B3-15
Tian, Y., Shen, H., Xia, L., and Lu, J. (2013). Elevated serum and synovial fluid levels of interleukin-34 in rheumatoid arthritis: possible association with disease progression via interleukin-17 production. J. Interferon Cytokine Res. 33, 398–401. doi: 10.1089/jir.2012.0122
Tokunaga, T., Mokuda, S., Kohno, H., Yukawa, K., Kuranobu, T., Oi, K., et al. (2020). TGFβ1 Regulates human RANKL-induced osteoclastogenesis via suppression of NFATc1 expression. Int. J. Mol. Sci. 21:800. doi: 10.3390/ijms21030800
Ushach, I., and Zlotnik, A. (2016). Biological role of granulocyte macrophage colony-stimulating factor (GM-CSF) and macrophage colony-stimulating factor (M-CSF) on cells of the myeloid lineage. J. Leukoc. Biol. 100, 481–489. doi: 10.1189/jlb.3RU0316-144R
Wang, J., Meng, F., Song, W., Jin, J., Ma, Q., Fei, D., et al. (2018). Nanostructured titanium regulates osseointegration via influencing macrophage polarization in the osteogenic environment. Int J Nanomedicine. 13, 4029–4043. doi: 10.2147/IJN.S163956
Wang, J., Zheng, Z., Huang, B., Wu, H., Zhang, X., Chen, Y., et al. (2020). Osteal Tissue macrophages are involved in endplate osteosclerosis through the OSM-STAT3/YAP1 signaling axis in modic changes. J. Immunol. 205, 968–980. doi: 10.4049/jimmunol.1901001
Wei, F., Li, M., Crawford, R., Zhou, Y., and Xiao, Y. (2019). Exosome-integrated titanium oxide nanotubes for targeted bone regeneration. Acta Biomater. 86, 480–492. doi: 10.1016/j.actbio.2019.01.006
Wei, F., Zhou, Y., Wang, J., Liu, C., and Xiao, Y. (2018). The immunomodulatory role of BMP-2 on macrophages to accelerate osteogenesis. Tissue Eng. A 24, 584–594. doi: 10.1089/ten.tea.2017.0232
Weilner, S., Keider, V., Winter, M., Harreither, E., Salzer, B., Weiss, F., et al. (2016). Vesicular Galectin-3 levels decrease with donor age and contribute to the reduced osteo-inductive potential of human plasma derived extracellular vesicles. Aging 8, 16–33. doi: 10.18632/aging.100865
Winkler, I. G., Sims, N. A., Pettit, A. R., Barbier, V., Nowlan, B., Helwani, F., et al. (2010). Bone marrow macrophages maintain hematopoietic stem cell (HSC) niches and their depletion mobilizes HSCs. Blood 116, 4815–4828. doi: 10.1182/blood-2009-11-253534
Woo, S. R., Corrales, L., and Gajewski, T. F. (2015). Innate immune recognition of cancer. Annu. Rev. Immunol. 33, 445–474. doi: 10.1146/annurev-immunol-032414-112043
Wu, A. C., He, Y., Broomfield, A., Paatan, N. J., Harrington, B. S., Tseng, H. W., et al. (2016). CD169+ macrophages mediate pathological formation of woven bone in skeletal lesions of prostate cancer. J. Pathol. 239, 218–230. doi: 10.1002/path.4718
Wu, Q., Zhou, X., Huang, D., Ji, Y., and Kang, F. (2017). IL-6 enhances osteocyte-mediated osteoclastogenesis by promoting JAK2 and RANKL activity in vitro. Cell. Physiol. Biochem. 41, 1360–1369. doi: 10.1159/000465455
Wynn, T. A., Chawla, A., and Pollard, J. W. (2013). Macrophage biology in development, homeostasis and disease. Nature 496, 445–455. doi: 10.1038/nature12034
Xia, Y., He, X. T., Xu, X. Y., Tian, B. M., An, Y., and Chen, F. M. (2020). Exosomes derived from M0, M1 and M2 macrophages exert distinct influences on the proliferation and differentiation of mesenchymal stem cells. PeerJ. 8:e8970. doi: 10.7717/peerj.8970
Xiang, L. X., Ran, Q., Chen, L., Xiang, Y., Li, F. J., Zhang, X. M., et al. (2020). CR6-interacting factor-1 contributes to osteoclastogenesis by inducing receptor activator of nuclear factor κB ligand after radiation. World J. Stem Cells 12, 222–240. doi: 10.4252/wjsc.v12.i3.222
Xing, Z., Lu, C., Hu, D., Miclau, T. 3rd, and Marcucio, R. S. (2010). Rejuvenation of the inflammatory system stimulates fracture repair in aged mice. J. Orthop. Res. 28, 1000–1006. doi: 10.1002/jor.21087
Xiong, Y., Chen, L., Yan, C., Zhou, W., Yu, T., Sun, Y., et al. (2020). M2 Macrophagy-derived exosomal miRNA-5106 induces bone mesenchymal stem cells towards osteoblastic fate by targeting salt-inducible kinase 2 and 3. J. Nanobiotechnol. 18:66. doi: 10.1186/s12951-020-00622-5
Xue, J., Schmidt, S. V., Sander, J., Draffehn, A., Krebs, W., Quester, I., et al. (2014). Transcriptome-based network analysis reveals a spectrum model of human macrophage activation. Immunity 40, 274–288. doi: 10.1016/j.immuni.2014.01.006
Xue, Y. Z. B., Niu, Y. M., Tang, B., and Wang, C. M. (2019). PCL/EUG scaffolds with tunable stiffness can regulate macrophage secretion behavior. Prog. Biophys. Mol. Biol. 148, 4–11. doi: 10.1016/j.pbiomolbio.2019.05.006
Yang, D. H., and Yang, M. Y. (2019). The role of macrophage in the pathogenesis of osteoporosis. Int. J. Mol. Sci. 20:2093. doi: 10.3390/ijms20092093
Yang, J., Zou, Y., and Jiang, D. (2018). Honokiol suppresses proliferation and induces apoptosis via regulation of the miR-21/PTEN/PI3K/AKT signaling pathway in human osteosarcoma cells. Int. J. Mol. Med. 41, 1845–1854. doi: 10.3892/ijmm.2018.3433
Yang, M., Li, C. J., Sun, X., Guo, Q., Xiao, Y., Su, T., et al. (2017). MiR-497~195 cluster regulates angiogenesis during coupling with osteogenesis by maintaining endothelial Notch and HIF-1α activity. Nat. Commun. 8:16003. doi: 10.1038/ncomms16003
Ying, W., Riopel, M., Bandyopadhyay, G., Dong, Y., Birmingham, A., Seo, J. B., et al. (2017). Adipose tissue macrophage-derived exosomal miRNAs can modulate in vivo and in vitro insulin sensitivity. Cell 171, 372–384.e12. doi: 10.1016/j.cell.2017.08.035
Zhang, H., Lin, C., Zeng, C., Wang, Z., Wang, H., Lu, J., et al. (2018). Synovial macrophage M1 polarisation exacerbates experimental osteoarthritis partially through R-spondin-2. Ann. Rheum. Dis. 77, 1524–1534. doi: 10.1136/annrheumdis-2018-213450
Zhang, Y., Böse, T., Unger, R. E., Jansen, J. A., Kirkpatrick, C. J., and van den Beucken, J. (2017). Macrophage type modulates osteogenic differentiation of adipose tissue MSCs. Cell Tissue Res. 369, 273–286. doi: 10.1007/s00441-017-2598-8
Keywords: macrophages, intracellular communication, macrophage-derived exosome, bone remodeling, bone diseases
Citation: Chen K, Jiao Y, Liu L, Huang M, He C, He W, Hou J, Yang M, Luo X and Li C (2020) Communications Between Bone Marrow Macrophages and Bone Cells in Bone Remodeling. Front. Cell Dev. Biol. 8:598263. doi: 10.3389/fcell.2020.598263
Received: 24 August 2020; Accepted: 27 November 2020;
Published: 22 December 2020.
Edited by:
Mario Antonio Bianchet, Johns Hopkins University, United StatesReviewed by:
Bin Tang, Southern University of Science and Technology, ChinaZhongyu Liu, Third Affiliated Hospital of Sun Yat-sen University, China
Junichi Kikuta, Osaka University, Japan
Copyright © 2020 Chen, Jiao, Liu, Huang, He, He, Hou, Yang, Luo and Li. This is an open-access article distributed under the terms of the Creative Commons Attribution License (CC BY). The use, distribution or reproduction in other forums is permitted, provided the original author(s) and the copyright owner(s) are credited and that the original publication in this journal is cited, in accordance with accepted academic practice. No use, distribution or reproduction is permitted which does not comply with these terms.
*Correspondence: Changjun Li, bGljaGFuZ2p1bkBjc3UuZWR1LmNu