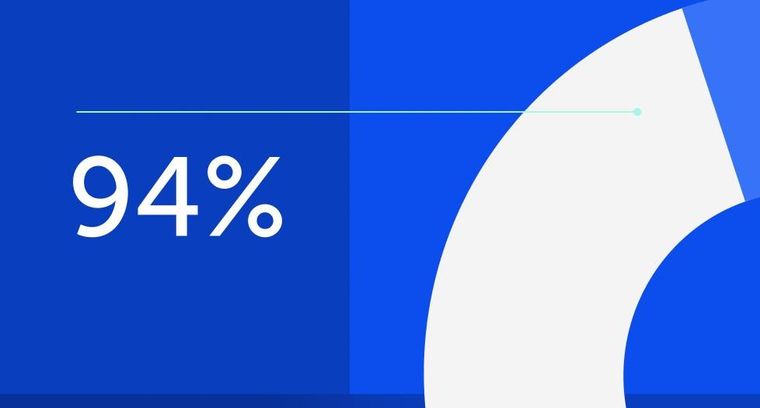
94% of researchers rate our articles as excellent or good
Learn more about the work of our research integrity team to safeguard the quality of each article we publish.
Find out more
MINI REVIEW article
Front. Clin. Diabetes Healthc., 02 June 2023
Sec. Diabetes Multiorgan Complications
Volume 4 - 2023 | https://doi.org/10.3389/fcdhc.2023.1171091
WFS1 spectrum disorder (WFS1-SD) is a rare monogenic neurodegenerative disorder whose cardinal symptoms are childhood-onset diabetes mellitus, optic atrophy, deafness, diabetes insipidus, and neurological signs ranging from mild to severe. The prognosis is poor as most patients die prematurely with severe neurological disabilities such as bulbar dysfunction and organic brain syndrome. Mutation of the WFS1 gene is recognized as the prime mover of the disease and responsible for a dysregulated ER stress signaling, which leads to neuron and pancreatic β-cell death. There is no currently cure and no treatment that definitively arrests the progression of the disease. GLP-1 receptor agonists appear to be an efficient way to reduce elevated ER stress in vitro and in vivo, and increasing findings suggest they could be effective in delaying the progression of WFS1-SD. Here, we summarize the characteristics of GLP-1 receptor agonists and preclinical and clinical data obtained by testing them in WFS1-SD as a feasible strategy for managing this disease.
Wolfram syndrome type 1 spectrum disorder (WFS1-SD, OMIM: 222300, 614296) is a rare multi-systemic monogenic disease that comprises classic WFS1 spectrum disorder and nonclassic WFS1 spectrum disorder. Classic WFS1-SD is an autosomal recessive progressive neurodegenerative disorder characterized by the onset of diabetes mellitus and optic atrophy before the age of 16 years. Additional manifestations may include variable hearing impairment/deafness, diabetes insipidus, neurologic abnormalities, neurogenic bladder, and psychiatric abnormalities. Nonclassic WFS1-SD is less common than classic WFS1-SD and is autosomal dominant. Phenotypes that appear to be milder than classic WFS1-SD include optic atrophy and hearing impairment; neonatal diabetes, profound congenital deafness, and cataracts; isolated diabetes mellitus; isolated congenital cataracts; and isolated congenital, slowly progressive, and low-frequency (<2000 Hz) sensorineural hearing loss (1). In both forms, the prognosis is poor with a median survival between 30 and 40 years. Death occurs usually as a consequence of severe neurological disabilities, mainly from respiratory failure caused by brain stem atrophy (2).
The causative gene of WFS1-SD is WFS1, contrarily from the less common Wolfram syndrome type 2 (WS2) which is caused by autosomal recessive mutations of the CISD2 (CDGSH iron-sulfur domain-containing protein 2) gene. The two diseases also differ in symptoms, being WS2 characterized by bleeding, upper intestinal ulcer, defective platelet aggregation, and absence of diabetes insipidus and psychiatric disorders (3, 4).
WFS1 codes for wolframin (WFS1), a transmembrane protein highly expressed in brain tissue, pancreatic β-cells, heart, lung, and placenta (5), and localized in the membrane of the endoplasmic reticulum (ER), where it plays a crucial role in maintaining ER homeostasis (4, 6, 7). ER is a cellular organelle responsible for the storage of Ca2+ ions, and for the correct folding and post-translational modification of several proteins (secretory proteins, cell surface receptors, integral membrane proteins, neurotransmitters, and hormones) (8). WFS1 loss of function causes an increase in the cytosolic concentration of Ca2+ ions, resulting in the establishment of chronic ER stress and the inappropriate activation of the unfolded protein response (UPR) signaling pathway (9). These events drive the cell to irreversible damage, which leads to apoptosis mainly in neuronal and pancreatic β-cells, where WFS1 expression is higher (7). Furthermore, it has been shown that WFS1 has a role in the transport of Ca2+ from the ER to the mitochondria and can therefore affect mitochondrial function (10).
Over 200 distinct mutations have been identified so far in WFS1-SD patients and new variants continue to be reported over time (11–13). Several attempts have been made to identify a genotype-phenotype correlation. However, the main difficulty in this regard relies on the existence of such a large number of variants of the WFS1 gene and the small size of patient cohorts because of the rarity of the disease (14–17).
Presently, no cure is available and until now patients with WFS1-SD have only profited from substitutive therapies for diabetes mellitus or diabetes insipidus (18). However, the identification of pathological molecular mechanisms has inspired new approaches, which mainly aim to restore Ca2+ homeostasis and contain ER stress to slow down the progression of the disease (4). Among the drugs tested in vitro and preclinical models, Valproate (NCT03717909) and Dantrolene (NCT02829268) were selected to undergo clinical trial investigation as possible therapeutic options for WFS1-SD (18). Increasing evidence from preclinical tests and off-label use suggests Glucagon-like peptide 1 receptor agonists (GLP-1RAs) as another possible therapeutic option.
Glucagon-like peptide 1 (GLP-1) is a hormone produced from L-cells of the small intestine by alternative processing of the proglucagon in response to nutrient ingestion (19, 20).
GLP-1 exerts its action through the GLP-1 receptor (GLP-1R), a member of G protein-coupled receptors (GPCRs). In particular, GLP-1 binds and activates a class B GPCRs coupled to Gs protein, leading to the activation of the enzyme adenyl cyclase and the consequent production of cyclic adenosine monophosphate (cAMP). Subsequently, several signal transduction pathways are initiated, generally involving protein kinase A (PKA) and exchange protein directly activated by cAMP (EPAC). Noteworthy, among the GLP-1 receptor-mediated signaling events, there is an increase in intracellular Ca2+ levels (19, 21).
GLP-1 is an incretin hormone and its main action is to improve glycemic control by stimulating glucose-dependent insulin secretion and promoting insulin synthesis (19, 20, 22). Furthermore, it inhibits glucagon release from α-cells (23) and preserves β-cell mass through the stimulation of their proliferation and inhibition of apoptosis (24–27). Moreover, GLP-1 has additional metabolic effects, namely delaying gastric emptying thereby inducing satiety, inducing central appetite suppression, and increasing natriuresis and diuresis (28–30). GLP-1 signaling in the brain transmits metabolic information to the neurons responsible for feeding behavior (31) but is also implicated in cognitive functions, such as learning and memory (32). It was likewise described a cardioprotective role for GLP-1, being capable to decrease blood pressure, improving microvascular function, and reducing inflammation (33). Notably, GLP-1 has a well-established central role since GLP-1Rs are expressed in the postrema area, hippocampus, accumbent nucleus, solitary tract nucleus, thalamus, afferent vagal system, and some other regions of the brain (34). GLP‐1 can be released from the intestine and pass through blood–brain barrier and affect the brain, but also GLP-1-producing neurons are present in several areas of the brain stem and hypothalamus (35). It is currently of great interest that GLP-1 has neuroprotective effects and can decrease inflammation and apoptosis (36–39). Several in vivo and in vitro studies using preclinical models of neurodegenerative diseases show that GLP-1R activation reduces the production of pro-inflammatory cytokines and immune cell infiltration in tissues (38, 40–42). Furthermore, it was determined that to induce the neuroprotective effects, the GLP-1R must be activated in the brain (38). Considering the numerous beneficial effects of GLP-1, this pleiotropic hormone is an attractive candidate for the treatment of obesity, diabetes, and neurodegenerative disorders. However, GLP-1 cannot be used in its native form because it has a very short half-life (1-2 min), being rapidly degraded by the enzyme dipeptidyl-peptidase-4 (DPP-4) and undergoing renal elimination (19). Therefore, biochemically modified forms of GLP-1, namely GLP-1 receptor agonists (GLP-1RAs), were developed, capable to activate the GLP-1R but having improved bioavailability and extended half-life compared to the native hormone (43). GLP-1RAs were first developed for treating type 2 diabetes and obesity and are now considered an established class of hypoglycemic agents with a very low risk of hypoglycemia (44). Nevertheless, consistent evidence from in vitro studies and preclinical models suggests that GLP-1RAs may have broader pharmacological potential. By activating GLP-1R, they lead to cAMP production and consequently to an increased expression and/or activity of receptor tyrosine kinases PI3K/AKT, epidermal growth factor receptor (EGFR), and hypoxia-inducible factor 1-alpha (HIF-1α) (45). Thanks to these mechanisms, GLP-1RAs promote pancreatic β-cell neogenesis, stimulate cell growth and increase insulin synthesis in the β-cells. It was demonstrated that GLP-1RAs reduce ER stress with different mechanisms. In a mouse model with an excess of ER stress in pancreatic β-cells, the mice treatment with the GLP1-RA exendin-4 reduced the expression levels of the ER stress- related molecules immunoglobulin-binding protein (Bip) and C/EBP-homologous protein (CHOP) (46). Similarly, other authors showed that exendin-4 protects β-cells against ER stress by inducing the anti-apoptotic protein JunB and also Bip (47). In addition, they demonstrated that the GLP1-RA inactivated caspase 12 and upregulated Bcl-2 and X-chromosome–linked inhibitor of apoptosis protein, leading to the inhibition of mitochondrial apoptosis. In addition, GLP-1RAs reduce ER stress (46, 47) and lead to favorable metabolic reprogramming and redox homeostasis (19, 48). Several data in the literature demonstrate that GLP-1R activation can also positively affect autophagy (49–53), whose defects have been shown to play a pathogenic role in both type 1 and type 2 diabetes, and in neurodegenerative diseases (54–57). In addition, consistent evidence from in vitro studies and preclinical models indicates that GLP-1RAs exert anti-inflammatory effects by modulating the immune system (39). Bendotti et al. reported in detail that GLP-1RAs can modulate the immune system both in mice and humans, independently of the weight loss or glycemic state of the subject. In particular, GLP-1RAs modify the macrophage phenotype toward an anti-inflammatory phenotype and therefore suppress the macrophage secretion of different inflammatory cytokines. Moreover, as a consequence of GLP-1RAs treatment, the inhibition of migration of CD4+ lymphocytes, the decrease in immune cell recruitment, the reduction of monocytes migration and infiltration, and the decrease in the expression of several pro-inflammatory cytokines were determined (39). Hence, thanks to their pleiotropic properties, GLP-1RAs are emerging as suitable for the treatment of diseases associated with chronic inflammation, including type 1 and 2 diabetes, neurodegenerative diseases, atherosclerosis, diabetic nephropathy, asthma, psoriasis, nonalcoholic steatohepatitis (58, 59).
GLP-1RAs are recognized as a novel class of anti-diabetic drugs for the treatment of type 2 diabetes and have been suggested as an adjuvant treatment in type 1 diabetes as well (44, 60, 61). In particular, among the GLP-1 RAs, exenatide and liraglutide have been studied in patients with type 1 diabetes (61). GLP-1RAs induce glucose-dependent insulin secretion from pancreatic β-cells, enhance their growth and proliferation, increase their number, inhibit apoptosis, and induce insulin synthesis (62). Another focus of current studies is how non-insulin glucose-lowering medications affect weight loss. Among several anti-diabetic medications, a systemic analysis reveals that GLP1-RAs and dual GLP-1/Gastric inhibitory polypeptide (GIP) agonists such as tirzepatide are most effective at causing weight loss in patients with type 2 diabetes (63).
GLP-1R are abundantly expressed in the cardiovascular system and by binding them GLP-1RAs may have direct or indirect protective effects by improving cardiac function, increasing the cardiomyocytes activity, decreasing intravascular oxidative stress, inhibiting hepatocyte gluconeogenesis and oxidative stress, and promoting vasodilation (64, 65). Several extensive clinical studies have shown that GLP-1RAs may lower the incidence of cardiovascular events. These studies have been carefully reviewed elsewhere (83, 84), so we won’t go into greater detail about them here.
Recent research has focused on the GLP-1/GLP-1R axis’ protective role against ischemic brain injury. By improving cell survival signaling pathways, lowering ischemia-reperfusion injury, encouraging brain healing, and regulating inflammatory response and oxidative stress, the activation of GLP-1R can decrease the extent of cerebral infarction (66–68). By activating neuronal receptors (69), GLP-1RAs exert beneficial effects on memory function, motor activity, synapse morphology and synaptic function, neurogenesis, apoptosis prevention, and minimizing the chronic inflammation in the brains of various animal models, such as Alzheimer’s Disease, Parkinson’s Disease, amyotrophic lateral sclerosis, multiple sclerosis, or other neurodegenerative diseases (70, 71). However, GLP-1RAs are only studied and not yet used in therapy for neurodegenerative diseases.
Especially in its advanced phases, asthma is a relatively prevalent chronic lung illness characterized by chronic persistent airway inflammation and airway remodeling that cause incompletely reversible airway blockage. Numerous studies have demonstrated that GLP-1RAs reduce eosinophil production of IL-4, IL-8, and IL-13 and inhibit the PKA/NF-B signaling pathway in animal models of asthma (72). Therefore, for obese patients with asthma, GLP-1RAs therapy may represent a novel add-on therapy (73).
The first use of a GLP-1RA in a mouse model of WFS1-SD was reported in 2016 by Sedman et al. (74). In this study, the authors showed that exenatide was capable to lower the blood glucose level and to increase the insulin-to-glucose ratio during the glucose tolerance test, demonstrating the ability of this GLP-1RA to correct the impaired insulin secretion caused by wolframin deficiency. Similar results were obtained by Kondo et al. in 2018, which showed that exenatide could alleviate ER stress by increasing and partially restoring the amount of phosphorylated AMP-activated kinase (p-AMPK), and reducing thioredoxin interacting protein (TXNIP) production in the β-cells of the Wfs1−/− mice (75). Furthermore, for the first time, these authors described the effect of 24 weeks of treatment with liraglutide in a woman with WFS1-SD, reporting the ability of this GLP-1RA to modulate the β-cell function and to improve glycaemic control in this rare disease. A similar effect was obtained after the administration of the GLP-1RA dulaglutide in a case report of a WFS1-SD patient (76).
In the meanwhile, a research group at the University of Tartu constructed and validated a Wfs1-deficient rat. This model of WFS1-SD was described to develop a prominent diabetic phenotype and neurodegeneration of the brainstem and optic nerve very similarly to human patients (77). By using it, it was substantiated that the GLP-1RA liraglutide was effective in preventing the development of glucose intolerance, improving insulin and glucagon secretion control, and reducing ER stress in Langerhans islets in Wfs1−/− rats (78). Moreover, the treatment resulted capable to delay the onset of diabetes and protecting against the development of optic nerve atrophy and vision loss, even if it did not prevent progressive sensorineural hearing loss (79). The effectiveness of liraglutide in the Wfs1-deficient rat model of WFS1-SD was detected not only after early treatment but also when administrated after the onset of several symptoms to mimic a relatively late diagnosis of WFS1-SD in patients (80). In the same rat model, liraglutide has been shown to delay the progression of hyperglycemia and exert neuroprotective effects. In particular, it increased the number of neurons and the neuronal volume in Wfs1−/− animals’ dorsal nuclei, and decreased ER stress and neuroinflammation in the inferior olive. Finally, liraglutide protected the optic nerve axons from degeneration and counteracted retinal ganglion cell death (80). Recently it was suggested a combination treatment with 7,8-dihydroxyflavone (7,8-DHF) to further improve the liraglutide neuroprotective effect in the rat model of WFS1-SD (81).
Considering the overall results obtained in preclinical models of WFS1-SD and the case report data in a WFS1-SD patient (Table 1), IRCCS San Raffaele Hospital in Milan, Italy, started an off-label treatment of liraglutide in pediatric patients with WFS1-SD (18). Recently and for the first time, Frontino et al. reported in detail the follow-up of a small cohort of WFS1-SD patients treated with 1.8mg/day liraglutide for 8-27 months. In this study, four genetically determined WFS1-SD pediatric patients with insulin-dependent diabetes mellitus and optic atrophy were enrolled to obtain preliminary data regarding the safety, tolerability, and efficacy of daily treatment with liraglutide. The authors observed a decrease in insulin requirement, stabilization of neuro-ophthalmological and neurophysiological disease parameters, and no onset of new WFS1-SD-related symptoms at the latest follow-up (18). Accordingly, a very recent case report described the treatment of a WFS1-SD patient with 0.6 mg per day liraglutide in association with the chemical chaperone tauroursodeoxycholic acid (TUDCA) to mitigate ER stress, reduce insulin requirements, and improve glycemic control (82).
The numerous beneficial properties of GLP-1RAs and the well-recognized effect in type 2 diabetes encouraged testing GLP-1RAs in animal models of WFS1-SD to investigate if these compounds could be useful in the management of diabetes in this rare disease. All the GLP-1RAs tested for this purpose so far in WFS1-SD, namely exenatide, liraglutide, and dulaglutide (Table 1), improved the glycemic control both in rodents and in patients. This effect may depend on the fact that GLP-1 receptor-mediated signaling directly modulates the ER response, leading to the promotion of β-cell adaptation and preventing their apoptosis (46, 83). In addition, it was established an association of genetic variations in the WFS1 locus with reduced GLP-1-induced insulin secretion and a higher risk of type 2 diabetes (84). Thus, alterations of ER homeostasis deriving from WFS1 variants could be associated with impaired incretin action and, consequently, β-cell dysfunction (84). Therefore, it is possible to speculate that the activation of the GLP-1 receptor signal using GLP-1RAs could restore the incretin deficiency in WFS1-SD, alleviating insulin insufficiency and aiding glycemic control.
However, in WFS1-SD diabetes is only one of the disease manifestations, being the severe neurological disabilities responsible for the poor prognosis (4). In this regard, evidence highlighting the possible use of GLP-1RAs for the treatment of diseases associated with chronic inflammation and progressive neurodegeneration (38), raised the possibility that GLP-1RAs could counteract also the neurological manifestations in WFS1-SD by impairing neuronal inflammation. This appears a reasonable hypothesis since small peptides GLP-1RAs, including liraglutide and exendin-4, can play a central role and affect the brain because they easily cross the blood-brain barrier upon peripheral administration (19). In this regard, liraglutide protects against optic nerve atrophy and vision loss in the Wfs1 -/- rats (79–81) and WFS1-SD patients (18). These liraglutide-mediated neuroprotective effects may derive from its ability in modulating the ER stress response and eliciting ER proteostasis in brain cells (85). Another possible mechanism explaining the beneficial effect of GLP-1RAs in counteracting the progression of neurological manifestations in WFS1-SD is the ability of these molecules to modulate autophagy, whose defects have been shown to play a pathogenic role in neurodegenerative diseases (50, 52).
Recent discoveries highlighted that WFS1-SD is associated with chronic inflammation (11, 86). GLP-1RAs could be useful in WFS1-SD also in this context, due to their ability to modulate the immune system and inflammation by inducing a reduction in the production of proinflammatory cytokines (39).
Overall, current data suggest that GLP-1RAs could effectively be used as therapeutic agents in WFS1-SD. They can improve glycemic control and are promising candidates for delaying neuronal-related symptoms in patients with WFS1-SD. Future perspectives on this regard will be: (i) to verify that liraglutide alters the progression or improves the life expectancy of affected individuals by increasing the number of patients treated with this GLP-1RA; (ii) to reduce the number of administrations and increase the compliance of the patients by investigating if the GLP-1RA with longer half-life semaglutide may have the same effect; (iii) to investigate if the dual GLP-1RA/GIP agonists may provide even more promising results. In fact, other incretins, such as GIP, have also shown neuroprotective effects in animal models of neurodegenerative diseases. Newer dual GLP-1/GIP receptor agonists or so-called twincretins have also shown protective effects in murine models of AD and PD (87). These results are encouraging and suggest that the development of such dual agonists for the treatment of other neurodegenerative diseases such as WFS1-SD may be very promising.
All authors listed have made a substantial, direct, and intellectual contribution to the work and approved it for publication.
This work was supported by Ministero dell’Istruzione, dell’Università e della Ricerca (PRIN 2017WJZ9W9 to MP).
The authors declare that the research was conducted in the absence of any commercial or financial relationships that could be construed as a potential conflict of interest.
All claims expressed in this article are solely those of the authors and do not necessarily represent those of their affiliated organizations, or those of the publisher, the editors and the reviewers. Any product that may be evaluated in this article, or claim that may be made by its manufacturer, is not guaranteed or endorsed by the publisher.
1. Barrett T, Tranebjærg L, Gupta R, Rendtorff ND, Williams D, Wright B, et al. WFS1 spectrum disorder. In: Adam MP, Everman DB, Mirzaa GM, Pagon RA, Wallace SE, Bean LJH, et al, editors. GeneReviews(®). Seattle (WA): University of Washington (1993).
2. Kinsley BT, Swift M, Dumont RH, Swift RG. Morbidity and mortality in the wolfram syndrome. Diabetes Care (1995) 18(12):1566–70. doi: 10.2337/diacare.18.12.1566
3. Amr S, Heisey C, Zhang M, Xia XJ, Shows KH, Ajlouni K, et al. A homozygous mutation in a novel zinc-finger protein, ERIS, is responsible for wolfram syndrome 2. Am J Hum Genet (2007) 81(4):673–83. doi: 10.1086/520961
4. Pallotta MT, Tascini G, Crispoldi R, Orabona C, Mondanelli G, Grohmann U, et al. Wolfram syndrome, a rare neurodegenerative disease: from pathogenesis to future treatment perspectives. J Transl Med (2019) 17(1):238. doi: 10.1186/s12967-019-1993-1
5. Rigoli L, Caruso V, Salzano G, Lombardo F. Wolfram syndrome 1: from genetics to therapy. Int J Environ Res Public Health (2022) 19(6):3225. doi: 10.3390/ijerph19063225
6. Takei D, Ishihara H, Yamaguchi S, Yamada T, Tamura A, Katagiri H, et al. WFS1 protein modulates the free Ca(2+) concentration in the endoplasmic reticulum. FEBS Lett (2006) 580(24):5635–40. doi: 10.1016/Jfebslet.2006.09.007
7. Fonseca SG, Ishigaki S, Oslowski CM, Lu S, Lipson KL, Ghosh R, et al. Wolfram syndrome 1 gene negatively regulates ER stress signaling in rodent and human cells. J Clin Invest (2010) 120(3):744–55. doi: 10.1172/JCI39678
8. Groenendyk J, Agellon LB, Michalak M. Calcium signaling and endoplasmic reticulum stress. Int Rev Cell Mol Biol (2021) 363:1–20. doi: 10.1016/bs.ircmb.2021.03.003
9. Almanza A, Carlesso A, Chintha C, Creedican S, Doultsinos D, Leuzzi B, et al. Endoplasmic reticulum stress signalling - from basic mechanisms to clinical applications. FEBS J (2019) 286(2):241–78. doi: 10.1111/febs.14608
10. Angebault C, Fauconnier J, Patergnani S, Rieusset J, Danese A, Affortit CA, et al. ER-mitochondria cross-talk is regulated by the Ca(2+) sensor NCS1 and is impaired in wolfram syndrome. Sci. Signal (2018) 11(553). doi: 10.1126/scisignal.aaq1380
11. Panfili E, Mondanelli G, Orabona C, Belladonna ML, Gargaro M, Fallarino F, et al. Novel mutations in the WFS1 gene are associated with wolfram syndrome and systemic inflammation. Hum Mol Genet (2021) 30(3–4):265–76. doi: 10.1093/hmg/ddab040
12. Zhang X, et al. Comprehensive genetic analysis unraveled the missing heritability in a Chinese cohort with wolfram syndrome 1: clinical and genetic findings. Invest Ophthalmol Vis Sci (2022) 63(10):9. doi: 10.1167/iovs.63.10.9
13. Alías L, López de Heredia M, Luna S, Clivillé N, González-Quereda L, Gallano P, et al. Case report: de novo pathogenic variant in WFS1 causes wolfram-like syndrome debuting with congenital bilateral deafness. Front Genet (2022) 13:998898. doi: 10.3389/fgene.2022.998898
14. de Heredia ML, Clèries R, Nunes V. Genotypic classification of patients with wolfram syndrome: insights into the natural history of the disease and correlation with phenotype. Genet Med (2013) 15(7):497–506. doi: 10.1038/gim.2012.180
15. Smith CJ, Crock PA, King BR, Meldrum CJ, Scott RJ. Phenotype-genotype correlations in a series of wolfram syndrome families. Diabetes Care (2004) 27(8):2003–9. doi: 10.2337/diacare.27.8.2003
16. Hu K, Zatyka M, Astuti D, Beer N, Dias RP, Kulkarni A, et al. WFS1 protein expression correlates with clinical progression of optic atrophy in patients with wolfram syndrome. J Med Genet (2022) 59(1):65–74. doi: 10.1136/jmedgenet-2020-107257
17. Majander A, Jurkute N, Burté F, Brock K, João C, Huang H, et al. WFS1-associated optic neuropathy: genotype-phenotype correlations and disease progression. Am J Ophthalmol (2022) 241:9–27. doi: 10.1016/Jajo.2022.04.003
18. Frontino G, Raouf T, Canarutto D, Tirelli E, Di Tonno R, Rigamonti A, et al. Case report: off-label liraglutide use in children with wolfram syndrome type 1: extensive characterization of four patients. Front Pediatr (2021) 9:755365. doi: 10.3389/fped.2021.755365
19. Müller TD, Finan B, Bloom SR, D'Alessio D, Drucker DJ, Flatt PR, et al. Glucagon-like peptide 1 (GLP-1). Mol Metab (2019) 30:72–130. doi: 10.1016/Jmolmet.2019.09.010
20. Gribble FM, Reimann F. Metabolic messengers: glucagon-like peptide 1. Nat Metab (2021) 3(2):142–8. doi: 10.1038/s42255-020-00327-x
21. Graaf C, Donnelly D, Wootten D, Lau J, Sexton PM, Miller LJ, et al. Glucagon-like peptide-1 and its class b G protein-coupled receptors: a long march to therapeutic successes. Pharmacol Rev (2016) 68(4):954–1013. doi: 10.1124/pr.115.011395
22. Jones B, Bloom SR, Buenaventura T, Tomas A, Rutter GA. Control of insulin secretion by GLP-1. Peptides (2018) 100:75–84. doi: 10.1016/Jpeptides.2017.12.013
23. Nauck MA, Heimesaat MM, Behle K, Holst JJ, Nauck MS, Ritzel R, et al. Effects of glucagon-like peptide 1 on counterregulatory hormone responses, cognitive functions, and insulin secretion during hyperinsulinemic, stepped hypoglycemic clamp experiments in healthy volunteers. J Clin Endocrinol Metab (2002) 87(3):1239–46. doi: 10.1210/jcem.87.3.8355
24. Buteau J, El-Assaad W, Rhodes CJ, Rosenberg L, Joly E, Prentki M. Glucagon-like peptide-1 prevents beta cell glucolipotoxicity. Diabetologia (2004) 47(5):806–15. doi: 10.1007/s00125-004-1379-6
25. Li Y, Hansotia T, Yusta B, Ris F, Halban PA, Drucker DJ. Glucagon-like peptide-1 receptor signaling modulates beta cell apoptosis. J Biol Chem (2003) 278(1):471–8. doi: 10.1074/jbc.M209423200
26. Buteau J. GLP-1 receptor signaling: effects on pancreatic beta-cell proliferation and survival. Diabetes Metab (2008) 34(Suppl 2):S73–7. doi: 10.1016/S1262-3636(08)73398-6
27. Fusco J, Xiao X, Prasadan K, Sheng Q, Chen C, Ming YC, et al. GLP-1/Exendin-4 induces β-cell proliferation via the epidermal growth factor receptor. Sci Rep (2017) 7(1):9100. doi: 10.1038/s41598-017-09898-4
28. Nauck MA, Niedereichholz U, Ettler R, Holst JJ, Orskov C, Ritzel R, et al. Glucagon-like peptide 1 inhibition of gastric emptying outweighs its insulinotropic effects in healthy humans. Am J Physiol (1997) 273(5):E981–8. doi: 10.1152/ajpendo.1997.273.5.E981
29. Nauck MA, Meier JJ. Incretin hormones: their role in health and disease. Diabetes Obes Metab (2018) 20(Suppl 1):5–21. doi: 10.1111/dom.13129
30. Asmar A, Cramon PK, Asmar M, Simonsen L, Sorensen CM, Madsbad S, et al. The renal extraction and the natriuretic action of GLP-1 in humans depend on interaction with the GLP-1 receptor. J Clin Endocrinol Metab (2021) 106(1):e11–9. doi: 10.1210/clinem/dgaa643
31. Anderberg RH, Richard JE, Eerola K, López-Ferreras L, Banke E, Hansson C, et al. Glucagon-like peptide 1 and its analogs act in the dorsal raphe and modulate central serotonin to reduce appetite and body weight. Diabetes (2017) 66(4):1062–73. doi: 10.2337/db16-0755
32. During MJ, Cao L, Zuzga DS, Francis JS, Fitzsimons HL, Jiao X, et al. Glucagon-like peptide-1 receptor is involved in learning and neuroprotection. Nat Med (2003) 9(9):1173–9. doi: 10.1038/nm919
33. Fava S. Glucagon-like peptide 1 and the cardiovascular system. Curr Diabetes Rev (2014) 10(5):302–10. doi: 10.2174/1573399810666141030125830
34. Diz-Chaves Y, Herrera-Pérez S, González-Matías LC, Lamas JA, Mallo F. Glucagon-like peptide-1 (GLP-1) in the integration of neural and endocrine responses to stress. Nutrients (2020) 12(11):3304. doi: 10.3390/nu12113304
35. Katsurada K, Yada T. Neural effects of gut- and brain-derived glucagon-like peptide-1 and its receptor agonist. J Diabetes Investig (2016) 7(Suppl 1):64–9. doi: 10.1111/jdi.12464
36. Reich N, Hölscher C. The neuroprotective effects of glucagon-like peptide 1 in alzheimer's and parkinson's disease: an in-depth review. Front Neurosci (2022) 16:970925. doi: 10.3389/fnins.2022.970925
37. Zhao X, Wang M, Wen Z, Lu Z, Cui L, Fu C, et al. GLP-1 receptor agonists: beyond their pancreatic effects. Front Endocrinol (Lausanne) (2021) 12:721135. doi: 10.3389/fendo.2021.721135
38. Diz-Chaves Y, Mastoor Z, Spuch C, González-Matías LC, Mallo F. Anti-inflammatory effects of GLP-1 receptor activation in the brain in neurodegenerative diseases. Int J Mol Sci (2022) 23(17):9583. doi: 10.3390/ijms23179583
39. Bendotti G, Montefusco L, Lunati ME, Usuelli V, Pastore I, Lazzaroni E, et al. The anti-inflammatory and immunological properties of GLP-1 receptor agonists. Pharmacol Res (2022) 182:106320. doi: 10.1016/Jphrs.2022.106320
40. Qian Z, Chen H, Xia M, Chang J, Li X, Ye S, et al. Activation of glucagon-like peptide-1 receptor in microglia attenuates neuroinflammation-induced glial scarring via rescuing arf and rho GAP adapter protein 3 expressions after nerve injury. Int J Biol Sci (2022) 18(4):1328–46. doi: 10.7150/ijbs.68974
41. Lee CH, Jeon SJ, Cho KS, Moon E, Sapkota A, Jun HS, et al. Activation of glucagon-like peptide-1 receptor promotes neuroprotection in experimental autoimmune encephalomyelitis by reducing neuroinflammatory responses. Mol Neurobiol (2018) 55(4):3007–20. doi: 10.1007/s12035-017-0550-2
42. Lee YS, Park MS, Choung JS, Kim SS, Oh HH, Choi CS, et al. Glucagon-like peptide-1 inhibits adipose tissue macrophage infiltration and inflammation in an obese mouse model of diabetes. Diabetologia (2012) 55(9):2456–68. doi: 10.1007/s00125-012-2592-3
43. Andreasen CR, Andersen A, Knop FK, Vilsbøll T. How glucagon-like peptide 1 receptor agonists work. Endocr Connect (2021) 10(7):R200–12. doi: 10.1530/EC-21-0130
44. Nauck MA, Quast DR, Wefers J, Meier JJ. GLP-1 receptor agonists in the treatment of type 2 diabetes - state-of-the-art. Mol Metab (2021) 46:101102. doi: 10.1016/Jmolmet.2020.101102
45. Carlessi R, Chen Y, Rowlands J, Cruzat VF, Keane KN, Egan L, et al. GLP-1 receptor signalling promotes β-cell glucose metabolism via mTOR-dependent HIF-1α activation. Sci Rep (2017) 7(1):2661. doi: 10.1038/s41598-017-02838-2
46. Tsunekawa S, Yamamoto N, Tsukamoto K, Itoh Y, Kaneko Y, Kimura T, et al. Protection of pancreatic beta-cells by exendin-4 may involve the reduction of endoplasmic reticulum stress; in vivo and in vitro studies. J Endocrinol (2007) 193(1):65–74. doi: 10.1677/JOE-06-0148
47. Cunha DA, Ladrière L, Ortis F, Igoillo-Esteve M, Gurzov EN, Lupi R, et al. Glucagon-like peptide-1 agonists protect pancreatic beta-cells from lipotoxic endoplasmic reticulum stress through upregulation of BiP and JunB. Diabetes (2009) 58(12):2851–62. doi: 10.2337/db09-0685
48. Monti G, Gomes Moreira D, Richner M, Mutsaers HAM, Ferreira N, Jan A. GLP-1 receptor agonists in neurodegeneration: neurovascular unit in the spotlight. Cells (2022) 11(13). doi: 10.3390/cells11132023
49. Yang S, Lin C, Zhuo X, Wang J, Rao S, Xu W, et al. Glucagon-like peptide-1 alleviates diabetic kidney disease through activation of autophagy by regulating AMP-activated protein kinase-mammalian target of rapamycin pathway. Am J Physiol Endocrinol Metab (2020) 319(6):E1019–30. doi: 10.1152/ajpendo.00195.2019
50. Chen J, Wang Z, Mao Y, Zheng Z, Chen Y, Khor S, et al. Liraglutide activates autophagy via GLP-1R to improve functional recovery after spinal cord injury. Oncotarget (2017) 8(49):85949–68. doi: 10.18632/oncotarget.20791
51. Zummo FP, Krishnanda SI, Georgiou M, O'Harte FP, Parthsarathy V, Cullen KS, et al. Exendin-4 stimulates autophagy in pancreatic β-cells via the RAPGEF/EPAC-Ca(2+)-PPP3/calcineurin-TFEB axis. Autophagy (2022) 18(4):799–815. doi: 10.1080/15548627.2021.1956123
52. Arden C. A role for glucagon-like peptide-1 in the regulation of β-cell autophagy. Peptides (2018) 100:85–93. doi: 10.1016/Jpeptides.2017.12.002
53. Zummo FP, Cullen KS, Honkanen-Scott M, Shaw JAM, Lovat PE, Arden C. Glucagon-like peptide 1 protects pancreatic β-cells from death by increasing autophagic flux and restoring lysosomal function. Diabetes (2017) 66(5):1272–85. doi: 10.2337/db16-1009
54. Muralidharan C, Linnemann AK. β-cell autophagy in the pathogenesis of type 1 diabetes. Am J Physiol Endocrinol Metab (2021) 321(3):E410–6. doi: 10.1152/ajpendo.00151.2021
55. Quan W, Lim YM, Lee MS. Role of autophagy in diabetes and endoplasmic reticulum stress of pancreatic β-cells. Exp Mol Med (2012) 44(2):81–8. doi: 10.3858/emm.2012.44.2.030
56. Jung HS, Chung KW, Won Kim J, Kim J, Komatsu M, Tanaka K, et al. Loss of autophagy diminishes pancreatic beta cell mass and function with resultant hyperglycemia. Cell Metab (2008) 8(4):318–24. doi: 10.1016/Jcmet.2008.08.013
57. Stavoe AKH, Holzbaur ELF. Autophagy in neurons. Annu Rev Cell Dev Biol (2019) 35:477–500. doi: 10.1146/annurev-cellbio-100818-125242
58. Lee YS, Jun HS. Anti-inflammatory effects of GLP-1-Based therapies beyond glucose control. Mediators Inflamm 2016 (2016), 3094642. doi: 10.1155/2016/3094642
59. Hölscher C. Protective properties of GLP-1 and associated peptide hormones in neurodegenerative disorders. Br J Pharmacol (2022) 179(4):695–714. doi: 10.1111/bph.15508
60. Zhu J, Han J, Liu L, Liu Y, Xu W, Li X, et al. Clinical expert consensus on the assessment and protection of pancreatic islet β-cell function in type 2 diabetes mellitus. Diabetes Res Clin Pract (2023) 197:110568. doi: 10.1016/Jdiabres.2023.110568
61. Guyton J, Jeon M, Brooks A. Glucagon-like peptide 1 receptor agonists in type 1 diabetes mellitus. Am J Health Syst Pharm (2019) 76(21):1739–48. doi: 10.1093/ajhp/zxz179
62. Hope DCD, Vincent ML, Tan TMM. Striking the balance: GLP-1/Glucagon Co-agonism as a treatment strategy for obesity. Front Endocrinol (Lausanne) (2021) 12:735019. doi: 10.3389/fendo.2021.735019
63. Sinha R, Papamargaritis D, Sargeant JA, Davies MJ. Efficacy and safety of tirzepatide in type 2 diabetes and obesity management. J Obes Metab Syndr (2023). doi: 10.7570/jomes22067
64. Hirsch IB. The future of the GLP-1 receptor agonists. Jama (2019) 321(15):1457–8. doi: 10.1001/jama.2019.2941
65. Bakbak E, Terenzi DC, Trac JZ, Teoh H, Quan A, Glazer SA, et al. Lessons from bariatric surgery: can increased GLP-1 enhance vascular repair during cardiometabolic-based chronic disease? Rev. Endocr Metab Disord (2021) 22(4):1171–88. doi: 10.1007/s11154-021-09669-7
66. Zhang L, Zhang W, Tian X. The pleiotropic of GLP-1/GLP-1R axis in central nervous system diseases. Int J Neurosci (2023) 1–19. doi: 10.1080/00207454.2021.1924707
67. Li Y, Glotfelty EJ, Karlsson T, Fortuno LV, Harvey BK, Greig NH. The metabolite GLP-1 (9-36) is neuroprotective and anti-inflammatory in cellular models of neurodegeneration. J Neurochem (2021) 159(5):867–86. doi: 10.1111/jnc.15521
68. Grieco M, Giorgi A, Gentile MC, d'Erme M, Morano S, Maras B, et al. Glucagon-like peptide-1: a focus on neurodegenerative diseases. Front Neurosci (2019) 13:1112. doi: 10.3389/fnins.2019.01112
69. Hunter K, Hölscher C. Drugs developed to treat diabetes, liraglutide and lixisenatide, cross the blood brain barrier and enhance neurogenesis. BMC Neurosci (2012) 13:33. doi: 10.1186/1471-2202-13-33
70. Chen SD, Chuang YC, Lin TK, Yang JL. Alternative role of glucagon-like peptide-1 receptor agonists in neurodegenerative diseases. Eur J Pharmacol (2023) 938:175439. doi: 10.1016/Jejphar.2022.175439
71. Parsons CG. CNS repurposing - potential new uses for old drugs: examples of screens for alzheimer's disease, parkinson's disease and spasticity. Neuropharmacology (2019) 147:4–10. doi: 10.1016/Jneuropharm.2018.08.027
72. Mitchell PD, Salter BM, Oliveria JP, El-Gammal A, Tworek D, Smith SG, et al. Glucagon-like peptide-1 receptor expression on human eosinophils and its regulation of eosinophil activation. Clin Exp Allergy (2017) 47(3):331–8. doi: 10.1111/cea.12860
73. Toki S, Newcomb DC, Printz RL, Cahill KN, Boyd KL, Niswender KD, et al. Glucagon-like peptide-1 receptor agonist inhibits aeroallergen-induced activation of ILC2 and neutrophilic airway inflammation in obese mice. Allergy (2021) 76(11):3433–45. doi: 10.1111/all.14879
74. Sedman T, Rünkorg K, Krass M, Luuk H, Plaas M, Vasar E, et al. Exenatide is an effective antihyperglycaemic agent in a mouse model of wolfram syndrome 1. J Diabetes Res (2016) 2016:9239530. doi: 10.1155/2016/9239530
75. Kondo M, Tanabe K, Amo-Shiinoki K, Hatanaka M, Morii T, Takahashi H, et al. Activation of GLP-1 receptor signalling alleviates cellular stresses and improves beta cell function in a mouse model of wolfram syndrome. Diabetologia (2018) 61(10):2189–201. doi: 10.1007/s00125-018-4679-y
76. Scully KJ, Wolfsdorf JI. Efficacy of GLP-1 agonist therapy in autosomal dominant WFS1-related disorder: a case report. Horm Res Paediatr (2020) 93(6):409–14. doi: 10.1159/000510852
77. Plaas M, Seppa K, Reimets R, Jagomäe T, Toots M, Koppel T, et al. Wfs1- deficient rats develop primary symptoms of wolfram syndrome: insulin-dependent diabetes, optic nerve atrophy and medullary degeneration. Sci Rep (2017) 7(1):10220. doi: 10.1038/s41598-017-09392-x
78. Toots M, Seppa K, Jagomäe T, Koppel T, Pallase M, Heinla I, et al. Preventive treatment with liraglutide protects against development of glucose intolerance in a rat model of wolfram syndrome. Sci Rep (2018) 8(1):10183. doi: 10.1038/s41598-018-28314-z
79. Jagomäe T, Seppa K, Reimets R, Pastak M, Plaas M, Hickey MA, et al. Early intervention and lifelong treatment with GLP1 receptor agonist liraglutide in a wolfram syndrome rat model with an emphasis on visual neurodegeneration, sensorineural hearing loss and diabetic phenotype. Cells (2021) 10(11). doi: 10.3390/cells10113193
80. Seppa K, Toots M, Reimets R, Jagomäe T, Koppel T, Pallase M, et al. GLP-1 receptor agonist liraglutide has a neuroprotective effect on an aged rat model of wolfram syndrome. Sci Rep (2019) 9(1):15742. doi: 10.1038/s41598-019-52295-2
81. Seppa K, Jagomäe T, Kukker KG, Reimets R, Pastak M, Vasar E, et al. Liraglutide, 7,8-DHF and their co-treatment prevents loss of vision and cognitive decline in a wolfram syndrome rat model. Sci Rep (2021) 11(1):2275. doi: 10.1038/s41598-021-81768-6
82. Png D, Yeoh E, Tan C, Lim SC. A pair of siblings with wolfram syndrome: a review of the literature and treatment options. J Investig Med High Impact Case Rep (2023) 11:23247096221150631. doi: 10.1177/23247096221150631
83. Yusta B, Baggio LL, Estall JL, Koehler JA, Holland DP, Li H, et al. GLP-1 receptor activation improves beta cell function and survival following induction of endoplasmic reticulum stress. Cell Metab (2006) 4(5):391–406. doi: 10.1016/Jcmet.2006.10.001
84. Schäfer SA, Müssig K, Staiger H, Machicao F, Stefan N, Gallwitz B, et al. A common genetic variant in WFS1 determines impaired glucagon-like peptide-1-induced insulin secretion. Diabetologia (2009) 52(6):1075–82. doi: 10.1007/s00125-009-1344-5
85. Panagaki T, Michael M, Hölscher C. Liraglutide restores chronic ER stress, autophagy impairments and apoptotic signalling in SH-SY5Y cells. Sci Rep (2017) 7(1):16158. doi: 10.1038/s41598-017-16488-x
86. Morikawa S, Blacher L, Onwumere C, Urano F. Loss of function of WFS1 causes ER stress-mediated inflammation in pancreatic beta-cells. Front Endocrinol (Lausanne) (2022) 13:849204. doi: 10.3389/fendo.2022.849204
Keywords: Wolfram syndrome type 1, wolframin (WFS1), GLP-1RAs, diabetes, neurodegeneration
Citation: Panfili E, Frontino G and Pallotta MT (2023) GLP-1 receptor agonists as promising disease-modifying agents in WFS1 spectrum disorder. Front. Clin. Diabetes Healthc. 4:1171091. doi: 10.3389/fcdhc.2023.1171091
Received: 21 February 2023; Accepted: 22 May 2023;
Published: 02 June 2023.
Edited by:
Didac Mauricio, University of Vic - Central University of Catalonia, SpainReviewed by:
Seley Gharanei, University of Warwick, United KingdomCopyright © 2023 Panfili, Frontino and Pallotta. This is an open-access article distributed under the terms of the Creative Commons Attribution License (CC BY). The use, distribution or reproduction in other forums is permitted, provided the original author(s) and the copyright owner(s) are credited and that the original publication in this journal is cited, in accordance with accepted academic practice. No use, distribution or reproduction is permitted which does not comply with these terms.
*Correspondence: Maria Teresa Pallotta, bWFyaWEucGFsbG90dGFAdW5pcGcuaXQ=
Disclaimer: All claims expressed in this article are solely those of the authors and do not necessarily represent those of their affiliated organizations, or those of the publisher, the editors and the reviewers. Any product that may be evaluated in this article or claim that may be made by its manufacturer is not guaranteed or endorsed by the publisher.
Research integrity at Frontiers
Learn more about the work of our research integrity team to safeguard the quality of each article we publish.