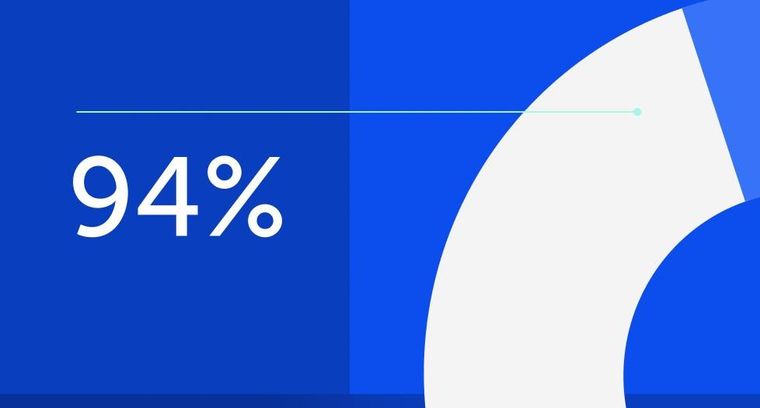
94% of researchers rate our articles as excellent or good
Learn more about the work of our research integrity team to safeguard the quality of each article we publish.
Find out more
ORIGINAL RESEARCH article
Front. Built Environ., 11 February 2025
Sec. Construction Materials
Volume 11 - 2025 | https://doi.org/10.3389/fbuil.2025.1535947
This article is part of the Research TopicInnovative Materials and Techniques for Sustainable ConstructionView all 3 articles
Rammed earth (RE) construction has gained increasing interest in recent years owing to sustainability demands in the construction industry and the advancement of digital fabrication techniques. However, the domination of the cement-stabilized RE material in the RE industry poses environmental concerns due to the substantial carbon emissions associated with cement production. In this study, bio-based alternatives to cement-stabilized RE are investigated through evaluating xanthan gum (XG) and animal glue (AG) as bio-binders for RE stabilization. Unconfined compressive strength tests are conducted on XG and AG-stabilized specimens for mechanical performance evaluation, and unstabilized RE samples as baseline for comparison. Results show that AG-stabilized specimens demonstrate a 294% strength improvement over unstabilized RE, reaching 6.86 MPa at 28 days, while XG-stabilized specimens achieve a 221% improvement. XG-stabilized specimens, however, exhibit susceptibility to microbial proliferation. The findings from this research demonstrate that XG and AG have the potential to be viable alternatives to mainstream RE construction methods, paving the way for advancing environmentally friendly RE construction.
The construction industry has embraced sustainability as a framework to address environmental challenges, emphasizing eco-conscious practices, circularity, and energy optimization (Citaristi, 2022; Gin et al., 2021). Local material utilization, represented by rammed earth construction, demonstrates both sustainability principles and economic viability in contemporary building practices (Matsumoto and Crook, 2021). Rammed earth (RE) construction, defined by the consecutive compaction of damp soil layers within formwork as shown in Figure 1, has been widely used to construct walls and buildings (Easton and Wright, 2007; Houben and Guillaud, 1994; Morel et al., 2021). RE is increasingly recognized as a low-tech, recyclable building material with good heat storage and moisture absorption properties (Mu et al., 2023). Originating millennia ago, this practice exhibits a widespread geographic footprint. Applications of RE have been recorded since the 14th century AD in China. From approximately 800 BC onwards, this construction technique is also prevalent in regions of North Africa and southern Europe (Jaquin et al., 2008). Nonetheless, given the labor-intensive nature of manual compaction in traditional RE methods (Walker et al., 2005), modern construction practices have shifted towards a predominant preference for concrete and timber construction, resulting in reduced application of RE.
Figure 1. Traditional rammed earth construction (Rammed earth consulting, 2013): (A) formwork; (B) ramming process using traditional manual rammers; (C) final rammed earth walls.
Recent records indicate a resurgence in the adoption of RE methods, attributed largely to the heightened emphasis on sustainable construction and advances in digital fabrication technologies (Ma et al., 2024a; Gomaa et al., 2023; Gomaa et al., 2022; Dabaieh and Sakr, 2015). Such a revival is particularly pronounced in regions encompassing Europe, Australia, New Zealand, and the United States (Easton and Wright, 2007). Recent work by Kloft et al. (2022), details the research infrastructure of the Collaborative Research Center TRR 277, emphasizing the role of digital fabrication technologies in enabling more sustainable construction practices. Ramage et al. (2015), highlight the relevance of natural construction materials, consistent with sustainable building trends. Recent research by Ramage et al. (2022), demonstrates the potential of sustainable materials for modern construction through innovative structural design, but further research is required to standardize and scale these emerging techniques towards widespread adoption. In contemporary RE construction, cement is adopted as a stabilizing agent to enhance the strength and durability of RE structures, significantly improving traditional methods (Losini et al., 2022). In detail, cement hydration acts to occupy the pores in the RE matrix, thereby enhancing particle cohesion and increasing compressive strength (Morel et al., 2007). Despite this, cement’s high CO2 emissions raise sustainability concerns (Dams et al., 2021). In response, some research is revisiting ancient building materials to provide sustainable options, demonstrated by the recent work of CobBauge project (Goodhew et al., 2019; University of Plymouth, 2023). The typical incorporation of cement ranges between 5% and 15% by weight (Ciancio et al., 2014). However, the production of cement is energetically demanding and might pose environmental concerns, accounting for approximately 8% of the global CO2 emissions (Zhang et al., 2012; Andrew, 2018). Additionally, for every metric ton of clinker produced, there is an associated generation of 0.2–0.4 metric tons of solid waste (Dams et al., 2021). In the meantime, cement production is associated with significant water consumption, ranging from 100 to 1,000 L per metric ton (Madlool et al., 2011). With the increased emphasis on carbon-neutral initiatives (Galluccio et al., 2019), the sustainability concerns surrounding cement-stabilized RE became more evident and warranted critical attention.
Bio-binders have been identified as a potential avenue to circumvent the use of cement, by transforming agricultural by-products into efficacious binding agents for RE. Recent studies have shown that bio-binders incorporating biochar demonstrate promising applications in both construction and environmental contexts (Xie et al., 2024). This aligns with broader research trends in sustainable construction materials, such as Javadian et al.’s work on bamboo-based composites for structural reinforcement (Javadian et al., 2020). Preliminary investigations have been conducted on 45 unique natural additives to assess their efficacy in stabilizing earthen materials (Fatehi et al., 2021; Losini et al., 2021). A gamut of natural substances including gums, lignin, tannins, proteins, and selected oils have been quantitatively investigated as potential eco-friendly binding agents for soil stabilization. Studies have shown that biopolymers like guar gum can provide higher compressive strength compared to cement stabilization in some cases (Muguda et al., 2017). Lignosulfonates have also been effective in increasing strength of clayey soils, with 0.75% treatment leading to a 44% increase in unconfined compressive strength after 28 days compared to un-stabilized soil (Ta’negonbadi and Noorzad, 2017). Proteins are another biopolymer class that can substantially increase soil strength, demonstrated by a 257% increase in California Bearing Ratio (CBR) strength of sand treated with 1% sodium caseinate after 14 days compared to untreated soil (Fatehi et al., 2018). Industrial lignin-rich byproducts have also shown promise as soil stabilizers. It is reported that 12% lignin can achieve 8% improved CBR strength than quicklime with 15 days desiccation time (Zhang et al., 2017).
Among the aforementioned natural additives, Xanthan gum (XG) is reported to exhibit high viscosity and stability over a wide pH range, while animal glue (AG) has shown time-dependent effectiveness in traditional construction methods (Kulshreshtha et al., 2022). XG can be generated via microbial fermentation of sugars derived from lignocellulosic biomass. Agricultural by-products such as coconut shells, passionfruit peels, straw, and corn cobs can be converted into fermentable sugars. These sugars can then be used as raw materials for industrial xanthan production (dos Santosa et al., 2016; Murad et al., 2019; Rashidi et al., 2023). AG adhesives, traditionally produced from animal skins and bones, can be manufactured using protein-rich agricultural wastes as raw materials. The collagen in these wastes can be hydrolyzed to produce binding agents with desired performance (Idris et al., 2010; Gunorubon and Misel, 2014; McKinney, 2023; Piercy et al., 2023).
While several studies have investigated natural additives for soil stabilization, the comparative performance of XG and AG as bio-binders for rammed earth remains unexplored. In this research, experimental tests are conducted to evaluate the compressive strength of XG and AG-stabilized rammed earth specimens against unstabilized RE baseline samples. The susceptibility of these stabilized specimens to microbial growth is also examined.
This section outlines the materials and experimental methodologies employed, including soil selection, bio-binders, sample preparation techniques, testing protocols, and analytical approaches.
The soil utilized in this paper is procured from a quarry situated in Mansfield region in Victoria, Australia. The soil has been classified by the quarry as red crushed rocks 20 mm minus. Its particle size distribution meets the stipulations set forth by Australian Standards AS 1726-1993 and AS 1289.3.6.2-1995 (Standards Australia, 1993, Standards Australia, 1995), as illustrated in Figure 2.
Figure 2. Raw soil composition: (A) soil with dimension less than 2.36 mm composing 13% by mass of raw soil; (B) soil with dimension between 13.2 and 2.36 mm composing 55% by mass of raw soil; (C) soil with dimension greater than 13.2 mm composing 32% by mass of raw soil.
To precisely control the moisture content for the RE samples, the soil undergoes desiccation for 24 h to eliminate the water composition according to the Australian standards AS 1289.2.1.1-2005 (Australian Standards, 2005). In addition, particle size distribution is also adjusted by post-sieving as suggested by the Australian Standards AS 1289.3.6.1-2009 (Australian Standards, 2009) for improved performance, which is listed in Table 1. The standard recommends 19 mm and 2.36 mm as the particle sizes for sieving. Given the 100 mm cube sample size in this investigation, the 19 mm sieve is unsuitable due to the sample-to-aggregate size ratio. As a result, sieve sizes of 13.2 mm and 2.36 mm are employed. Through this sequential sieving approach, the raw soil is fractionated into coarse (>13.2 mm), medium (13.2–2.36 mm), and fine (<2.36 mm) particles. The fractions are recombined in equal proportions of 33.3% each to ensure consistent particle size distribution across all specimens.
Ziboxan F80 (from Deosen Biochemical Ltd.) is adopted as the XG bio-binder in this study. It is an off-the-shelf product presented in a cream-colored powdered form. Ziboxan F80 is soluble in water at room temperature and has a particle size distribution wherein 100% of the particles pass through a 60-mesh screen (250 μm), and approximately 95% pass through an 80-mesh screen (180 μm). The viscosity of the selected XG ranges between 1,200 and 1,600 cp when dissolved in a 1% KCl solution to a concentration of 1%.
The specific AG adopted in this study is bovine gelatines derived from cattle skins and available in powder form on the market (Gelita Gelatine produced by GELITA Australia Pty Ltd., 2018). The adhesive strength of AG can be categorized into various grades termed as “blooms”. In this research, AG with 200 Bloom is examined (190–210 g) and 250 Bloom (240–260 g), which are indicative of the gelatine’s adhesive strength. Both AG with 200 Bloom and 250 Bloom are classified as 14 mesh (1,400 μm) powders and are soluble in water at 55°C–65°C.
The key parameters that determine the performance of XG and AG are the associated viscosity for XG and the bloom strength for AG. These attributes significantly influence their respective binding and gelling capabilities. Additionally, factors such as solubility and concentration can also affect the performance of these bio-binders (GELITA Australia Pty Ltd, 2018, GELITA Australia Pty Ltd, 2019; Deosen Biochemical Ltd, 2020). Therefore, preliminary tests are necessary to determine the suitable mixing method and concentration for each bio-binder.
Preliminary investigations are conducted to determine optimal water content and bio-binder concentrations. Test specimens of 100 mm cubes are prepared with water content ratios of 5%, 7%, and 10%, and bio-binder concentrations of 1%, 2%, and 3% for both XG and AG. Specimens are subjected to compression testing after seven-day desiccation under controlled conditions at 20°C and 50% relative humidity. Drop tests are performed following (Keable and Keable, 2011) guidelines to assess suitable moisture content. Based on workability constraints and preliminary strength results, the optimum water and binder ratios are selected.
Wet and dry mixing techniques are established construction practices (Burroughs, 2008; Kumar, 2009). In this study, distinct mixing protocols are adopted for XG and AG while maintaining binder and water contents at 1% and 10% of soil dry weight, respectively. XG is incorporated through dry mixing with soil prior to adding water at room temperature at 20°C, whereas AG undergoes wet mixing by first dissolving in hot water at 70°C before soil incorporation. These mixing approaches are selected based on preliminary trials to optimize binder distribution and effectiveness, with detailed results presented in Section 3.1. A Hobart A200-1102 mixer is used with 3 min mixing duration to ensure thorough binder dispersion throughout the soil matrix.
A customized metal mold is designed and fabricated to confine and shape the RE samples with a size of 100 × 100 × 100 mm as shown in Figure 3. Bolt assembly is implemented on the side walls to facilitate demolding. In addition, the side walls are fastened with the base plate to prevent vertical movement during ramming. Each mold section has an equal thickness of 15 mm. To improve surface quality of the RE samples and facilitate the demolding process, polyethylene films are attached to the inner walls of the mold as a release layer.
Figure 3. Customized RE mold: (A) mold assembled by bolts horizontally and vertically; (B) inner wall of the mold without release layer; (C) inner wall of the mold with release layer.
In this study, the mixture is deposited and compacted into five successive layers. The number of layers is chosen based on the sample height of 100 mm, resulting in a layer thickness of approximately 20 mm. This layering approach ensures uniform compaction throughout the sample, which is essential for achieving consistent compression results. To ensure effective and efficient compaction, each layer undergoes a compaction duration of 15–20 s using a Kawasaki KPT-2L lightweight industrial sand tamper. The Kawasaki KPT-2L delivers rapid strikes at 1,000 blows/minute, encompassing a 65 mm stroke length, and a 50 mm butt diameter. After compaction, the top surface of each sample is flattened to obviate stress concentration in the compression tests. The formwork is removed immediately after compaction. Prepared samples are then stored under controlled environmental conditions (20°C and 50% relative humidity). The mix proportions for stabilized and un-stabilized samples are investigated and presented in the results section. The detailed preparation methodology to manufacture RE samples can be seen in Figure 4.
Figure 4. RE sample preparation process: (A) Kawasaki KPT-2L rammer; (B) soil mixing; (C) first layer of soil before ramming; (D) pneumatic ramming; (E) first layer of soil after ramming; (F) detailed compaction of the final top surface; (G) demolding the RE sample; (H) fresh sample after demolding.
To evaluate the stabilized strength of the produced RE samples, unconfined compressive strength (UCS) tests are conducted at intervals of 7 and 28 days, respectively according to Sun et al. (2022) and Yan et al. (2022). A Shimadzu Autograph AGS-X Universal Tester is utilized to perform the UCS as shown in Figure 5. Considering the size of the RE samples, a compression loading rate of 500 N/s is applied (Ma et al., 2024b).
To investigate the sustainability and reusability of the RE samples produced with bio-binders, demolished wastes from the compression tests are examined through visual inspection for microbial growth. The specimens are stored under controlled environmental conditions at 20°C and 50% relative humidity for 28 days.
Preliminary dissolution experiments are conducted to verify the solubility of XG and AG with a concentration of 1% of the total weight of soil. According to recommendations from the suppliers, XG and AG can be dissolved in water at 20°C and 70°C, respectively. The dissolution experiment shows that AG can dissolve in 70°C water rapidly and uniformly. In contrast, XG dissolves slowly at room temperature and forms flocculent aggregate see Figure 6. Furthermore, it is found that XG shows poor workability when dissolved in hot water at the selected concentrations and exhibits clumpy distribution in cold water. Based on the above findings, dry mixing is selected for XG and wet mixing is selected for AG to facilitate their uniform distribution in the RE mixing and maximize their binding performance.
Figure 6. Dissolution of XG (left) and AG (right): (A) before dissolution; (B) XG dissolved in 20°C water and AG dissolved in 70°C water; (C) dissolved XG and AG after 24 h desiccation time in 20°C.
To maximize binding performance, bio-binder concentration and moisture content are investigated. Concentrations are calculated as percentages of soil weight. UCS are conducted on stabilized and un-stabilized RE samples with varying constitutions. Each constitution has 3 samples and a 7-day desiccation period as specified in Table 2.
In preliminary drop tests, unstabilized RE specimens with a 10% water content retain a nearly monolithic form upon impact. In contrast, those containing 5% or 7% water shatter into multiple fragments. Therefore, 10% water is considered the optimal within the selection range. The incorporation of 3% XG and AG in stabilized samples leads to excessive viscosity, resulting in poor workability during mixing. Consequently, these mixture designs are excluded from further consideration. Among the stabilized formulations, samples with 1% XG and 1% AG attained average strengths of 0.92 MPa and 1.36 MPa, respectively, outperforming their 2% XG (0.69 MPa) and 2% AG (1.33 MPa) counterparts. Based on the preliminary 7-day outcomes, 1% XG and AG binder concentrations are selected for maximizing the RE compressive strength. 10% moisture content is applied to both stabilized and un-stabilized RE samples. UCS tests after 7-day and 28-day desiccation periods are carried out to compare the stabilized soil samples with all selected bio-binders as well as the un-stabilized samples. For each category, three samples are prepared, resulting in a total of 24 samples, which are categorized as shown in Table 3.
The final rammed earth samples with 1% XG, 1% AG, and un-stabilized counterparts after 28-day desiccation are presented in Figure 7. Table 4 shows the UCS results of RE samples with the different stabilization settings, after 7-day or 28-day desiccation. The average strength of each sample category is visualized in Figure 8 for comparison.
Figure 8. Maximum compressive strength of RE samples stabilized by different settings over 7 and 28 days.
At 7 days of desiccation, un-stabilized RE samples present a compressive strength of 1.02 MPa on average, whereas AG-stabilized samples (200 and 250 Bloom) exhibited higher strength at 2.33 and 3.07 MPa on average. By 28 days, AG with 200 Bloom recorded 6.86 MPa, slightly outperforming AG with 250 Bloom at 6.73 MPa. Interestingly, 1% XG surpasses AG counterpart at 7 days with an average strength of 3.74 MPa, yet it trails at 28 days, where it records 5.58 MPa. Meanwhile, the un-stabilized RE samples show a steady increment, achieving 1.74 MPa at the 28-day mark. It is evident that the desiccation period and binder type critically influence the compressive strength of stabilized RE.
The peak recorded strength of the RE samples stands at 6.86 MPa for AG with 200 Bloom at 28 days, followed by 6.73 MPa for AG with 250 Bloom at 28 days and 5.58 MPa for XG at 28 days. In comparison, the un-stabilized RE samples underperform the stabilized RE samples by 72.7%–56.2% in 7 days and 74.6%–68.8% in 28 days. The 7-day and 28-day results offer insights into the binder efficacy over time.
Binder-soil interactions can influence microbial growth and subsequently affect material properties. Therefore, microbial observations are implemented using raw soil mixed with 1% XG and 1% AG 200 Bloom, respectively. They are stored under controlled conditions at 20°C and 50% humidity for 28 days without ramming. Figure 9 shows that extensive fungus appeared in the soil-XG combination, whereas no evident fungus is found in the soil-AG combination. This observation implies that AG exhibits longer durability as a bio-binder for RE, while XG-stabilized RE may have fast degradation due to growth of fungus. Further investigation into fungal development differences between loose and compacted specimens could provide insight into effects of density and air voids on microbial resistance. Post treatments such as protective coatings are required for XG-stabilized RE to mitigate fungus damage and attain comparable durability.
Figure 9. Microbial growth: (A) fungus (circled by yellow dash line) identified in soil mixed with XG, (B) no visible fungus can be identified in soil mixed with AG. Both soils from (A) and (B) are stored in 20°C with 50% humidity for 28 days.
Crushed RE samples are assessed after UCS. Demolished soil from AG-stabilized samples exhibits typical soil sizes (≤20 mm), which is ready for reuse (Figure 10). Nevertheless, demolished wastes from XG-stabilized samples result in large agglomeration (≥80 mm).
Figure 10. Demolished soils from UCS tests from: (A) three XG-stabilized RE samples; (B) three AG-stabilized RE samples.
These observations provide preliminary insights into the reusability of RE stabilized by bio-binders. However, further studies are required to assess the performance, durability, and practicality of using demolished soil in new RE construction.
This study investigates the potential of using XG and AG as bio-binders for rammed earth stabilization. As discussed by Morel et al. (2021), earth materials naturally embed properties aligning with sustainability and circular economy goals. The production of AG and XG from agricultural waste products aligns with the principles of sustainable construction and circular economy principles. The unconfined compressive strength results in this study demonstrate that both XG and AG introduce notable strength enhancements compared to un-stabilized soil samples. When compared to unstabilized baseline samples, XG-stabilized RE demonstrated a strength increase of 267% at 7 days, reaching 3.74 MPa compared to 1.02 MPa. AG-stabilized specimens showed improvements of 128% and 201% for 200 Bloom and 250 Bloom variants respectively during the same period. After 28 days of desiccation, AG 200 Bloom achieved the highest strength gain of 294% at 6.86 MPa relative to the baseline of 1.74 MPa, followed by AG 250 Bloom at 287% and XG-stabilized specimens at 221%.
Recent studies on cement stabilization (Amede et al., 2024) reveal that achieving equivalent UCS values to AG-stabilized RE using 1% AG content requires approximately 14% cement content. For one ton of rammed earth mixture, AG stabilization necessitates 10 kg of AG, costing 150 AUD, compared to 140 kg of cement, costing 70 AUD. Nevertheless, the production of Portland cement requires an extremely high temperature of 1,450°C for limestone calcination and clinker formation, normally achieved through fossil fuel combustion (Worrell et al., 2008; Scrivener et al., 2018). This high-temperature processing is a major contributor to industrial energy consumption and CO2 emissions (Mikulčić et al., 2013; Schneider et al., 2011). In contrast, animal glue extraction operates at significantly lower temperatures of 60°C–65°C, representing substantially reduced thermal energy requirements in comparison to cement production (Idris et al., 2010; Negash G. et al., 2020). Although AG dissolution requires initial heating to 55°C–65°C before mixing and is priced higher at 15 AUD/kg compared to cement, its significantly lower required content of 1% versus 14% makes it an efficient alternative. Combined with its derivation from agricultural waste, AG offers a promising opportunity to reduce cement usage, which accounts for approximately 8% of global CO2 emissions. These findings indicate that bio-binders represented by AG and XG could potentially replace cement in rammed earth applications while maintaining structural performance and reducing environmental impact.
The microbial observation reveals that the XG-soil combination provides an ideal environment for fungal growth, whereas the AG-soil combination shows no microbial presence after 28 days. This implies degraded durability for XG-stabilized rammed earth. Additional protective surface treatments would be necessary to mitigate biodeterioration mechanisms if XG is preferred as the binder. In contrast, AG seems to present innate resistance to microbial damage.
Waste characterization studies indicate the demolished AG-stabilized rammed earth fragments into typical soil particle sizes, retaining intrinsic soil attributes. Hence, construction wastes from AG-stabilized rammed earth have potential to be reused for new construction without grinding. However, the XG-soil matrix exhibits large agglomerations after demolition, necessitating additional processing before reuse.
While providing detailed analysis, further controlled tests are required to systematically evaluate sustainability and performance of bio-binders in rammed earth. Although AG shows promise over XG, standardized methods must isolate binder chemistry effects on stabilization and durability. Refining mixing processes could optimize distribution for both binders. Future assessment should focus on evaluating impacts related to preparation consistency using standardized techniques. Only through further rigorous testing can optimal bio-based formulae be uncovered to support durable, low carbon rammed earth construction in line with sustainability objectives prioritizing reduced emissions and waste.
This study provides a foundation for further research on rammed earth stabilized with bio-binders, particularly AG and XG. The stabilization mechanisms of AG and XG in RE require further investigation to optimize their strength contribution. Future studies are necessary to investigate the durability, water resistance, reusability, and life cycle assessment to facilitate the practical application of these bio-binders. Analysis of new water demand and fine particle content are needed to further justify the reusability of the AG and XG-stabilized rammed earth. Building upon the findings of this study, further research can contribute to the wider adoption of bio-stabilized rammed earth as a sustainable construction technique, aligning with the principles of eco-friendly and circular economy practices.
In this study, xanthan gum (XG) and animal glue (AG) are evaluated as bio-binders for rammed earth as sustainable alternatives to cement. Concentrations of bio-binders and moisture contents are determined to be 1% and 10%, respectively, to maximize the compressive strength of the RE. The preparation procedures of XG-stabilized and AG-stabilized RE are systematically investigated, where XG demonstrates suitability for dry mixing, and AG for wet mixing. UCS tests are conducted on the produced RE samples with 7- and 28-day desiccation times. Although XG-stabilized RE has the highest compressive strength at 3.74 MPa after 7 days, its compressive strength is lower than AG-stabilized RE after 28 days. Notably, AG-stabilized RE in the 200 Bloom configuration yields the highest compressive strength at 6.86 MPa in 28 days, outperforming both the 5.58 MPa achieved by XG-stabilized RE and 1.74 MPa achieved by unstabilized RE. Additionally, AG-stabilized RE samples attain adequate reusability in terms of anti-fungal properties and fine particle distribution after crushing, which are less prominent in the XG-stabilized RE. Although further research is required to investigate the durability of the two bio-binders for RE, both XG and AG present great opportunities as environmentally friendly alternatives to cement as RE stabilizers. The research outcome is useful to facilitate the advancement of RE materials in future architecture and infrastructure applications.
The raw data supporting the conclusions of this article will be made available by the authors, without undue reservation.
AA: Conceptualization, Data curation, Formal Analysis, Investigation, Methodology, Visualization, Writing–original draft. JM: Formal Analysis, Methodology, Supervision, Visualization, Writing–review and editing. MG: Conceptualization, Supervision, Writing–review and editing. FG: Methodology, Writing–review and editing. YX: Conceptualization, Funding acquisition, Supervision, Writing–review and editing, Resources.
The author(s) declare that financial support was received for the research, authorship, and/or publication of this article. This work is funded by the Australian Research Council (FL190100014).
The authors gratefully acknowledge the financial support provided by the Australian Research Council (FL190100014). They are also grateful for the valuable support from Olnee Rammed Earth Pty Ltd. and Gelita Australia Pty Ltd.
The authors declare that the research was conducted in the absence of any commercial or financial relationships that could be construed as a potential conflict of interest.
The author(s) declare that no Generative AI was used in the creation of this manuscript.
All claims expressed in this article are solely those of the authors and do not necessarily represent those of their affiliated organizations, or those of the publisher, the editors and the reviewers. Any product that may be evaluated in this article, or claim that may be made by its manufacturer, is not guaranteed or endorsed by the publisher.
Amede, E. A., Aklilu, G. G., Kidane, H. W., and Dalbiso, A. D. (2024). Examining the viability and benefits of cement-stabilized rammed earth as an affordable and durable walling material in Addis Ababa, Ethiopia. Cogent. Eng. 11, 2318249. doi:10.1080/23311916.2024.2318249
Andrew, R. M. (2018). Global CO 2 emissions from cement production. Earth. Syst. Sci. 10, 195–217. doi:10.5194/essd-10-195-2018
Burroughs, S. (2008). Soil property criteria for rammed earth stabilization. J. Mater. Civ. Eng. 20, 264–273. doi:10.1061/(asce)0899-1561(2008)20:3(264)
Ciancio, D., Beckett, C. T. S., and Carraro, J. A. H. (2014). Optimum lime content identification for lime stabilised rammed earth. Constr. Build. Mater. 53, 59–65. doi:10.1016/j.conbuildmat.2013.11.077
Citaristi, I. (2022). United nations environment programme—UNEP, in the europa directory of international organizations 2022. London: Routledge, 193–199.
Dabaieh, M., and Sakr, M. (2015). “Transdisciplinarity in rammed earth construction for contemporary practice,” in The international conference on vernacular heritage, sustainability and earthen architecture (London, UK: Taylor and Francis), 107–114.
Dams, B., Maskell, D., Shea, A., Allen, S., Cascione, V., Walker, P., et al. (2021). Upscaling non-residential bio-based circular construction in the United Kingdom. IOP. Conf. Ser. Earth. Environ. Sci. 855, 012015. doi:10.1088/1755-1315/855/1/012015
Dos Santosa, F. P., Jra, A. M. O., Nunesa, T. P., de Farias Silvab, C. E., and de Souza Abud, A. K. (2016). Bioconversion of agro-industrial wastes into xanthan gum. Chem. Eng. 49, 145–150.
Fatehi, H., Abtahi, S. M., Hashemolhosseini, H., and Hejazi, S. M. (2018). A novel study on using protein based biopolymers in soil strengthening. Constr. Build. Mater. 167, 813–821. doi:10.1016/j.conbuildmat.2018.02.028
Fatehi, H., Ong, D. E. L., Yu, J., and Chang, I. (2021). Biopolymers as green binders for soil improvement in geotechnical applications: a review. Geosciences 11, 291. doi:10.3390/geosciences11070291
Galluccio, S., Beirau, T., and Pöllmann, H. (2019). Cement as a climate killer: using industrial residues to produce carbon neutral alternatives. Available at: https://pressemitteilungen.pr.uni-halle.de/index.php?modus=pmanzeige&pm_id=3083 (Accessed December 14, 2023).
GELITA Australia Pty Ltd (2018). 250 Bloom 14 Mesh edible bovine gelatine (Revision D) Product specification.
GELITA Australia Pty Ltd (2019). 200 bloom 14 mesh edible bovine gelatine (Revision C) Product specification.
Gin, Y., Saner, B. B., and Ramage, M. H. (2021). “Robotic 3D printing with earthen materials as a novel sustainable construction method,” in Proceedings of the IASS annual symposium 2020/21 and the 7th international conference on spatial structures, 1–10.
Gomaa, M., Jabi, W., Soebarto, V., and Xie, Y. M. (2022). Digital manufacturing for earth construction: a critical review. J. Clean. Prod. 338, 130630. doi:10.1016/j.jclepro.2022.130630
Gomaa, M., Schade, S., Bao, D. W., and Xie, Y. M. (2023). Automation in rammed earth construction for industry 4.0: precedent work, current progress and future prospect. J. Clean. Prod. 398, 136569. doi:10.1016/j.jclepro.2023.136569
Goodhew, S., Carfrae, J., Hood-Cree, K., Fox, M., Boutouil, M., and Streiff, F. (2019). Building with earth: how we are working to revive an ancient, sustainable building technique. Constr. Res. Innov. 10, 105–108. doi:10.1080/20450249.2019.1700077
Gunorubon, A. J., and Misel, U. (2014). Production of glues from animal bones. ARPN. J. Eng. Appl. Sci. 9, 1592–1597.
Houben, H., and Guillaud, H. (1994). Earth construction: a comprehensive guide. London, UK: Intermediate Technology Publications.
Idris, A., Saed, K., and Hung, Y.-T. (2010). Animal glue production from skin wastes Editors L. K. Wang, J.-H. Tay, S. T. L. Tay, and Y.-T. Hung (Totowa, NJ: Humana Press), 685–697.
Jaquin, P. A., Augarde, C. E., and Gerrard, C. M. (2008). Chronological description of the spatial development of rammed earth techniques. Int. J. Archit. Herit. 2, 377–400. doi:10.1080/15583050801958826
Javadian, A., Smith, I. F. C., and Hebel, D. E. (2020). Application of sustainable bamboo-based composite reinforcement in structural-concrete beams: design and evaluation. Mater 13, 696. doi:10.3390/ma13030696
Keable, J., and Keable, R. (2011). Rammed earth structures: a code of practice. Rugby: Practical Action.
Kloft, H., Dörfler, K., Bährens, M., Dielemans, G., Diller, J., Dörrie, R., et al. (2022). Die Forschungsinfrastruktur des SFB TRR 277 AMC Additive Fertigung im Bauwesen. Bautechnik 99, 758–773. doi:10.1002/bate.202200076
Kulshreshtha, Y., Vardon, P. J., Du, Y., Habert, G., Vissac, A., Morel, J.-C., et al. (2022). Biological stabilisers in earthen construction: a mechanistic understanding of their response to water-ingress. Constr. Technol. Archit. 1, 529–539. doi:10.4028/www.scientific.net/cta.1.529
Kumar, P. P. (2009). Stabilised rammed earth for walls: materials, compressive strength and elastic properties. Bangalore, India: Department of Civil Engineering, Indian Institute of Science. Available at: https://etd.iisc.ac.in/handle/2005/987 (Accessed December 25, 2023).
Losini, A. E., Grillet, A. C., Bellotto, M., Woloszyn, M., and Dotelli, G. (2021). Natural additives and biopolymers for raw earth construction stabilization–a review. Constr. Build. Mater. 304, 124507. doi:10.1016/j.conbuildmat.2021.124507
Losini, A. E., Grillet, A.-C., Woloszyn, M., Lavrik, L., Moletti, C., Dotelli, G., et al. (2022). Mechanical and microstructural characterization of rammed earth stabilized with five biopolymers. Mater 15, 3136. doi:10.3390/ma15093136
Ma, J., Abdelaal, A., Zhang, H., Zhou, A., Fu, Y., and Xie, Y. M. (2024a). Ultra-compressed earth block stabilized by bio-binder for sustainable building construction. Case. Stud. Constr. Mater. 21, e03523. doi:10.1016/j.cscm.2024.e03523
Ma, J., Zhang, H., San Ha, N., and Xie, Y. M. (2024b). “Exploiting auxetic confinement for enhancing structural performance of earth-based construction,” in Proceedings of the IASS 2024 symposium: redefining the art of structural design (Switzerland: Zurich), 26–30.
Madlool, N. A., Saidur, R., Hossain, M. S., and Rahim, N. A. (2011). A critical review on energy use and savings in the cement industries. Renew. Sustain. Energy. Rev. 15, 2042–2060. doi:10.1016/j.rser.2011.01.005
Matsumoto, T., and Crook, J. (2021). Sustainable and inclusive housing in Ethiopia: a policy assessment. London: Coalition for Urban Transitions.
McKinney, J. (2023). Animal glue. Available at: https://justuseglue.com/animal-glue/ (Accessed December 14, 2023).
Mikulčić, H., Vujanović, M., and Duić, N. (2013). Reducing the CO2 emissions in Croatian cement industry. Appl. Energy 101, 41–48. doi:10.1016/j.apenergy.2012.02.083
Morel, J.-C., Charef, R., Hamard, E., Fabbri, A., Beckett, C., and Bui, Q.-B. (2021). Earth as construction material in the circular economy context: practitioner perspectives on barriers to overcome. Philos. Trans. R. Soc. Lond. B. Biol. Sci. 376, 20200182. doi:10.1098/rstb.2020.0182
Morel, J.-C., Pkla, A., and Walker, P. (2007). Compressive strength testing of compressed earth blocks. Constr. Build. Mater. 21, 303–309. doi:10.1016/j.conbuildmat.2005.08.021
Mu, J., Yu, S., and Hao, S. (2023). Quantitative evaluation of thermal conductivity of earth materials with different particle size distributions. Renew. Sustain. Energy. Rev. 184, 113574. doi:10.1016/j.rser.2023.113574
Muguda, S., Booth, S. J., Hughes, P. N., Augarde, C. E., Perlot, C., Bruno, A. W., et al. (2017). Mechanical properties of biopolymer-stabilised soil-based construction materials. Géotech. Lett. 7, 309–314. doi:10.1680/jgele.17.00081
Murad, H. A., Abo-Elkhair, A. G., and Azzaz, H. H. (2019). Production of xanthan gum from nontraditional substrates with perspective of the unique properties and wide industrial applications. JSMC. Microbiol. 1, 6.
Negash G, T., Emiru, A., Amare, D., and Reda, M. (2020). “Production and characterization of glue from tannery hide trimming waste,” in Advances of Science and Technology: 7th EAI international conference, ICAST 2019. Bahir dar, Ethiopia (Springer International Publishing), 59–70.
Piercy, E., Verstraete, W., Ellis, P. R., Banks, M., Rockström, J., Smith, P., et al. (2023). A sustainable waste-to-protein system to maximise waste resource utilisation for developing food-and feed-grade protein solutions. Green. Chem. 25, 808–832. doi:10.1039/d2gc03095k
Ramage, M. H., Gatóo, A., and Al Asali, M. W. (2022). “Complex simplicity—design of innovative sustainable thin-shell masonry structures,” in From corbel arches to double curvature vaults: analysis, conservation and restoration of architectural heritage masonry structures (Cham, Switzerland: Springer), 257–281.
Ramage, M. H., Hall, T., and Rich, P. (2015). “Light earth designs: natural material, natural structure,” in Earthen architecture: past, present and future (London, UK: Taylor and Francis Group), 305–310.
Rammed earth consulting (2013). Afghanistan is a hard place to build: rammed earth works. Available at: https://rammedearthconsulting.com/rammed-earth-afghanistan.htm?form=MG0AV3 (Accessed November 11, 2024).
Rashidi, A. R., Azelee, N. I. W., Zaidel, D. N. A., Chuah, L. F., Bokhari, A., El Enshasy, H. A., et al. (2023). Unleashing the potential of xanthan: a comprehensive exploration of biosynthesis, production, and diverse applications. Bioproc. Biosyst. Eng. 46, 771–787. doi:10.1007/s00449-023-02870-9
Schneider, M., Romer, M., Tschudin, M., and Bolio, H. (2011). Sustainable cement production present and future. Cem. Concr. Res. 41, 642–650. doi:10.1016/j.cemconres.2011.03.019
Scrivener, K. L., John, V. M., and Gartner, E. M. (2018). Eco-efficient cements: potential economically viable solutions for a low-CO2 cement-based materials industry. Cem. Concr. Res. 114, 2–26. doi:10.1016/j.cemconres.2018.03.015
Standards Australia (1993). Geotechnical site investigations (AS 1726-1993). Sydney, Australia: Standards Australia.
Standards Australia (1995). Methods of testing soils for engineering purposes - soil classification tests - determination of the particle size distribution of a soil - analysis by sieving in combination with hydrometer analysis (subsidiary method) (AS 1289.3.6.2-1995). Sydney, Australia: Standards Australia.
Standards Australia (2005). AS 1289.2.1.1-2005, Methods of testing soils for engineering purposes - soil moisture content tests - determination of the moisture content of a soil - oven drying method. Sydney, Australia: Standards Australia.
Standards Australia (2009). AS 1289.3.6.1-2009, Methods of testing soils for engineering purposes - soil classification tests - determination of the particle size distribution of a soil - standard method by sieving in a moist condition. Sydney, Australia: Standards Australia.
Sun, Y., Wang, G., and Li, Y. (2022). Study on ultrasonic nondestructive testing of self-compacting concrete under uniaxial compression test. Mater 15, 4412. doi:10.3390/ma15134412
Ta’negonbadi, B., and Noorzad, R. (2017). Stabilization of clayey soil using lignosulfonate. Transp. Geotech. 12, 45–55. doi:10.1016/j.trgeo.2017.08.004
University of Plymouth (2023). The CobBauge project. Plymouth, UK: University of Plymouth. Available at: https://www.plymouth.ac.uk/research/cobbauge (Accessed December 14, 2023).
Walker, P., Keable, R., Martin, J., and Maniatidis, V. (2005). Rammed earth: design and construction guidelines. Rugby, UK: IHS BRE Press.
Worrell, E., Galitsky, C., and Price, L. (2008). Energy efficiency improvement and cost saving opportunities for cement making. LBNL-Rev. 54036, 159.
Xie, Y., Wang, H., Guo, Y., Wang, C., Cui, H., and Xue, J. (2024). Mechanical performance and water resistance of biochar admixture lightweight magnesium oxychloride cement. Sci. Total Environ. 912, 168773. doi:10.1016/j.scitotenv.2023.168773
Yan, J., Yuan, K., Zhang, F., and Guo, L. (2022). Study on mechanical properties and constitutive equation of earth materials under uniaxial compression. Appl. Sci. 13, 19. doi:10.3390/app13010019
Zhang, C., Tian, H., Chen, G., Chappelka, A., Xu, X., Ren, W., et al. (2012). Impacts of urbanization on carbon balance in terrestrial ecosystems of the Southern United States. Environ. Pollut. 164, 89–101. doi:10.1016/j.envpol.2012.01.020
Keywords: bio-binders, rammed earth, mechanical properties, sustainable materials, circular economy
Citation: Abdelaal A, Ma J, Gomaa M, Giustozzi F and Xie YM (2025) Stabilizing rammed earth using xanthan gum or animal glue as bio-binder. Front. Built Environ. 11:1535947. doi: 10.3389/fbuil.2025.1535947
Received: 28 November 2024; Accepted: 20 January 2025;
Published: 11 February 2025.
Edited by:
Vasudha Katare, National Institute of Technology Warangal, IndiaReviewed by:
Hindavi Gavali, NICMAR University Pune, IndiaCopyright © 2025 Abdelaal, Ma, Gomaa, Giustozzi and Xie. This is an open-access article distributed under the terms of the Creative Commons Attribution License (CC BY). The use, distribution or reproduction in other forums is permitted, provided the original author(s) and the copyright owner(s) are credited and that the original publication in this journal is cited, in accordance with accepted academic practice. No use, distribution or reproduction is permitted which does not comply with these terms.
*Correspondence: Yi Min Xie, bWlrZS54aWVAcm1pdC5lZHUuYXU=
Disclaimer: All claims expressed in this article are solely those of the authors and do not necessarily represent those of their affiliated organizations, or those of the publisher, the editors and the reviewers. Any product that may be evaluated in this article or claim that may be made by its manufacturer is not guaranteed or endorsed by the publisher.
Research integrity at Frontiers
Learn more about the work of our research integrity team to safeguard the quality of each article we publish.