- 1Maseeh Department of Civil, Architectural and Environmental Engineering, University of Texas at Austin, Austin, TX, United States
- 2Department of Civil and Environmental Engineering, Utah State University, Logan, UT, United States
- 3Department of Engineering, Wake Forest University, Winston-Salem, NC, United States
Introduction: Natural hazards present significant risks to infrastructure and communities, emphasizing the importance of advanced tools and methods to better understand and mitigate these challenges. The Natural Hazards Engineering Research Infrastructure (NHERI) experimental facility at the University of Texas (NHERI@UTexas), funded by the National Science Foundation (NSF), addresses this need by offering unique resources for large-scale field testing.
Methods: NHERI@UTexas contributes unique, large-scale, literally one-of-a-kind, mobile dynamic shakers and associated instrumentation to investigate seismic, cyclic, and static in-situ testing. These capabilities allow researchers to conduct experiments on actual infrastructure and geotechnical systems under real-world conditions, often impossible to accurately simulate in the laboratory.
Results: NHERI@UTexas has supported pioneering research in several areas, including: (1) enhanced 2D/3D geotechnical and seismic subsurface imaging, (2) in-situ characterization of liquefaction resistance and nonlinear dynamic soil behavior, (3) development of in-situ nondestructive soil-foundation-structure interaction (SFSI) methods, and (4) rapid geotechnical assessments following natural hazard events. These efforts have advanced the validation and calibration of numerical model and methodologies using full-scale experimental data.
Discussion: NHERI@UTexas enable researchers to gain new insights and drive innovations in advancing resilient and sustainable solutions for natural hazards problems.
1 Introduction
The Natural Hazards Engineering Research Infrastructure (NHERI) is a nationwide, shared-use network of large-scale facilities dedicated to advancing natural hazards engineering research. The NHERI program is funded by the National Science Foundation (NSF) with goal of enhancing the resilience of the civil infrastructure and communities against earthquakes, windstorms, tsunamis, and other natural hazards. A key aspect of NHERI is its nationally distributed facilities, which provide state-of-the-art experimental, computational, and simulation tools to support diverse research needs. The NSF-supported facilities not only offer access to cutting-edge technology, but also provide comprehensive data management, enabling the collection, storage, and sharing of valuable research data. This wealth of resources, combined with collaboration opportunities across institutions, is made available to the broader research community, facilitating innovation and the development of effective solutions to mitigate the impact of natural hazards.
In alignment with this purpose, the NHERI@UTexas, Large-Scale Mobile Shakers facility, supported 20 shared-use projects and more than 25 non-shared-use projects from 2016 to 2024. Shared-use projects are funded by NSF which are typically led by researchers from other universities, often in collaboration with UT researchers, and focus on developing new testing techniques to achieve specific goals. Shared-use projects are typically funded by the Division of Civil, Mechanical, and Manufacturing Innovation (CMMI). However, NHERI@UTexas has recently expanded its scope to include efforts that advance the understanding of Earth’s systems, allowing it to also secure funding from the Division of Earth Sciences (EAR). Over the past 5 years, about half of the shared-use projects have been supported by EAR funding. From designing field studies and developing sensors to conduct tests, uploading, and analyzing data, each project typically lasts between 1 to 3 years. Non-shared-use projects have not been supported by NSF and have typically been conducted by researchers at UT. These types of projects generally contribute to improving the sustainability and resilience of civil infrastructure against natural hazards, including nuclear power plants and, more recently, Small Modular Reactor (SMR) facilities. With rising concerns about global warming, nuclear energy is becoming a key zero-emission clean energy option. SMRs offer enhanced safety, flexibility, and cost advantages, which makes them a promising solution in the future of nuclear power generation. The NHERI@UTexas facility has been actively involved in researching soil stiffness profiles for earthquake response studies ranging in depth up to 450 m for both new and existing nuclear power plants and is recognized as a leader in this area. These types of projects often last about 6 months. In this article, the equipment capabilities at NHERI@UTexas are discussed, and key areas of investigation and example shared-use projects are presented. These examples showcase how NHERI@UTexas equipment contributes to advancements in various areas of research. More information about NHERI@UTexas and the NSF-supported NHERI program can be found at https://utexas.designsafe-ci.org/.
2 Overview of NHERI@UTexas
NHERI@UTexas provides unique large, mobile dynamic shakers and associated instrumentation for in-situ testing of civil infrastructure. These innovative field-testing methods can be used to evaluate the behavior of existing infrastructure as well as enhance the design of future infrastructure, which will contribute to the development of more resilient communities. While laboratory shake tables at both small and large scales provide valuable insights into dynamic infrastructure behavior, focusing on these methodologies exclusively, without the ability to test real structural and geotechnical systems under actual field conditions, would leave a significant gap in the transformative tools needed for the next frontier of natural hazards research.
The equipment available at the NHERI@UTexas experimental facility includes: (1) five large, hydraulically-controlled shakers mounted on mobile platforms (i.e., trucks) that can provide wide-band dynamic excitation sources for geotechnical and structural systems, (2) a tractor-trailer necessary to transport the four largest shakers, (3) a supply truck with resources for mobile shaker maintenance and refueling in the field, (4) an instrumentation van that houses data acquisition systems and power generators, (5) an air-conditioned instrumentation trailer that serves as a work space in the field, and (6) a wide array of field instrumentation, data acquisition systems, and various sensors for measuring vibrational motions and pore water pressures (Stokoe, et al., 2020).
The five mobile shakers, shown in Figure 1 and summarized in Table 1, are named (1) T-Rex, (2) Liquidator, (3) Raptor, (4) Rattler, and (5) Thumper. The force and frequency generation capabilities of these shakers are presented in Figure 2. The two heaviest shakers are T-Rex (29,000 kg) and Liquidator (32,800 kg). T-Rex (Figure 1A) can be used to generate large dynamic forces in any of three directions (vertical, horizontal in-line, and horizontal cross-line), and the shaking direction can be changed with a simple push of a button by the operator. The shaking system, mounted on an off-road, all-wheel-drive vehicle, can produce a maximum force output of around 267 kN in the vertical direction, and around 134 kN in each horizontal direction, as shown in Figures 2A, B, respectively. In addition to T-Rex’s shaking capabilities, it can also be used to: (1) push cone penetrometers and other custom-made vibration and/or pressure-sensing instrumentation into the ground using a hydraulic ram located on the rear bumper of the vehicle (shown in Figure 3D), and (2) perform pull-over tests of large-scale structural models in the field using a hydraulically-operated winch on the front bumper of the vehicle. In total, T-Rex’s capabilities make it unique in the world. Liquidator (Figure 1B) is a unique, custom-built shaker designed specifically for low-frequency, large-motion operation. To change the shaking direction from the vertical mode to the cross-line horizontal (shear) mode requires approximately two working days at the manufacturer’s facilities in Tulsa, OK. The shaker can generate a maximum force output of approximately 89 kN in either mode down to a frequency of 1.3 Hz, as shown in Figure 2. However, a modified configuration where the entire off-road mobile platform is lifted off the ground and oscillates in the vertical mode allows Liquidator to generate maximum forces of 89 kN down to a frequency of 0.7 Hz. Below 0.7 Hz, the force level decreases but is still substantial to about 0.3 Hz. This modification provides unique capabilities that can facilitate deeper (1 km or more) active-source subsurface imaging (Stokoe, et al., 2019). Like T-Rex, the Liquidator shaking system is also housed on an off-road vehicle with hydraulic penetrometer/instrumentation pushing capabilities mounted on the rear steel bumper of the vehicle and a winch with pull-over capabilities mounted on the front steel bumper. Use of these pull-over capacities are illustrated in field studies with 1/4-scale bridge bents by Stokoe, et al. (2017).
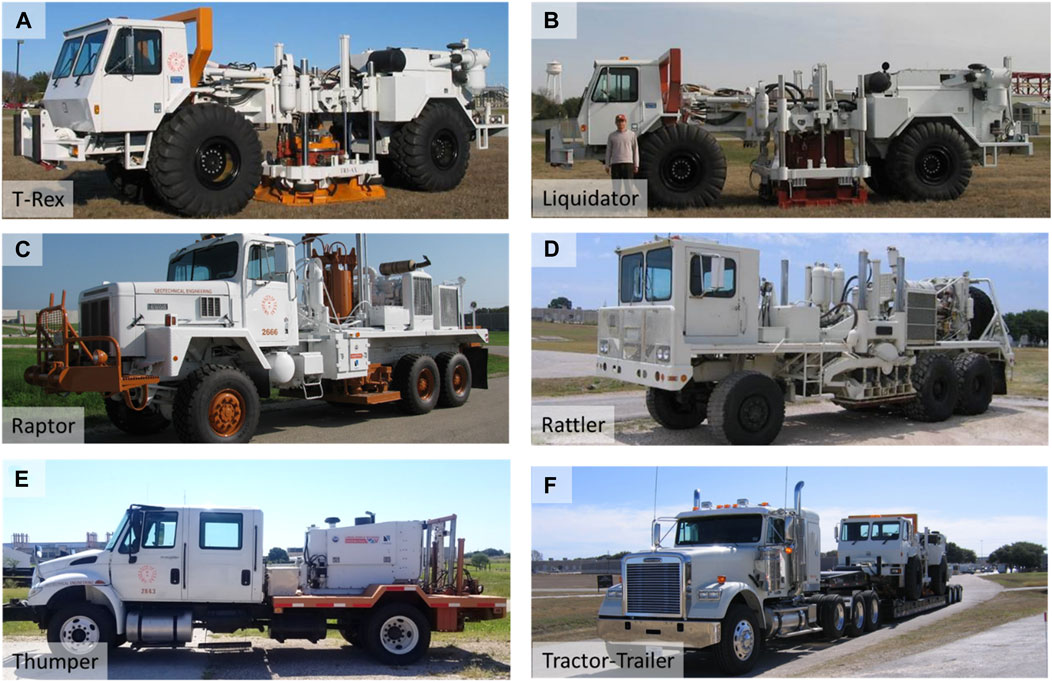
Figure 1. Photographs of the five mobile shakers and tractor-trailer rig available at NHERI@UTexas: (A) High-force, three-axis shaker called T-Rex, (B) Low-frequency, two-axis shaker called Liquidator, (C) Single-axis, vertical shaker called Raptor, (D) Single-axis, horizontal shaker called Rattler, (E) Urban, three-axis shaker called Thumper, and (F) Tractor-trailer rig, called the Big Rig, loaded with T-Rex (after Stokoe, et al., 2017).
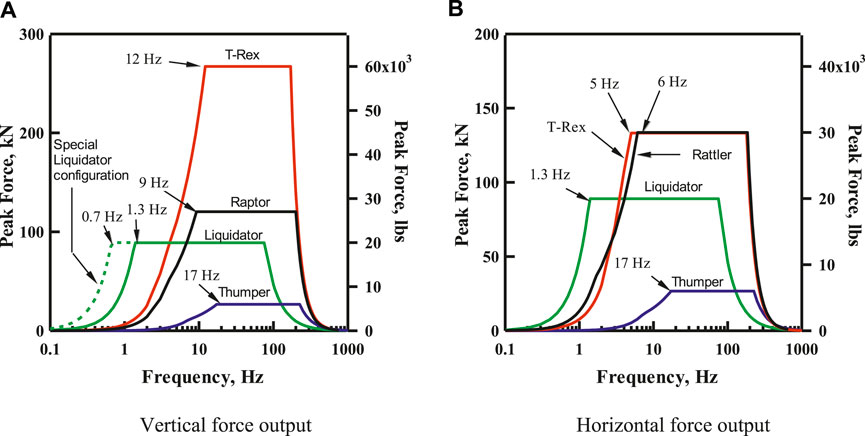
Figure 2. Theoretical force outputs of the five mobile shakers at NHERI@UTexas in the: (A) vertical mode and (B) horizontal mode (Stokoe, et al., 2017).
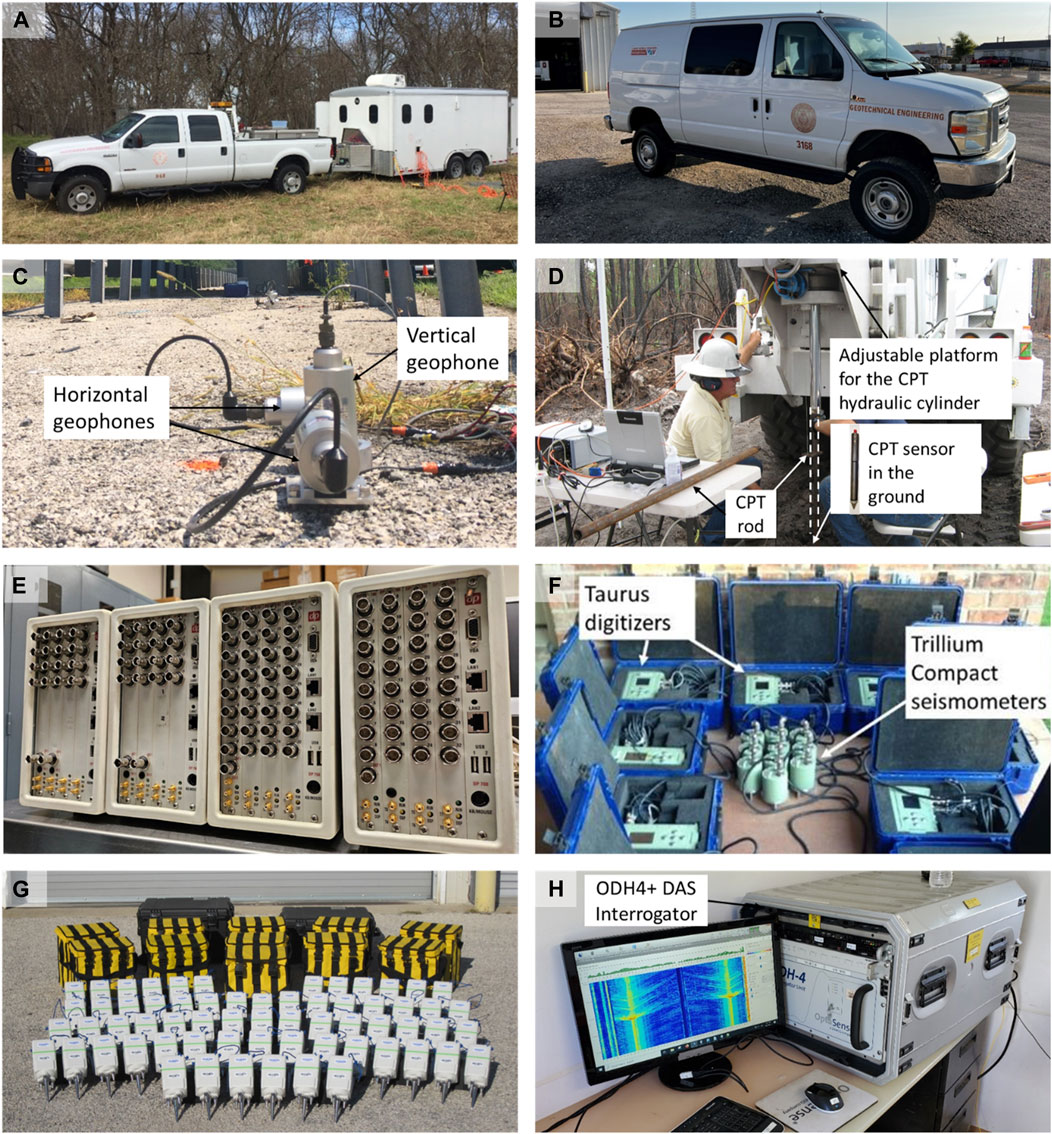
Figure 3. Photographs of the field supply truck, mobile instrumentation trailer and some associated instrumentation available at NHERI@UTexas: (A) Field supply truck and instrumentation trailer, (B) Customized Ford cargo van, (C) 1-Hz vertical geophones and cables, (D) Cone penetration test equipment, (E) Data Physics analyzers, (F) Trillium Compact Seismometers and Taurus Digitizers, (G) SmartSolo IGU-16HR 3C nodal stations, and (H) OptaSense ODH4 Distributed Acoustic Sensing (DAS) interrogator (after Stokoe, et al., 2017).
Raptor and Rattler provide intermediate-level force generation. Raptor (Figure 1C) is called a compression-wave (P-wave) shaker in the geophysical exploration community. The maximum vertical force output is about 120 kN, as shown in Figure 2A. Raptor is ideal for situations where Thumper’s force output (discussed below) is not sufficient for the desired testing application and T-Rex’s triaxial shaking capability and/or higher force output is not required. Rattler (Figure 1D) is a horizontal (shear-wave) vibrator mounted on an off-road vehicle. Rattler has a frequency-force response which is similar to T-Rex in the shear mode, as shown in Figure 2B. By having two shear-wave vibrators (T-Rex and Rattler), this equipment can be used simultaneously with synchronized force outputs to generate a larger surface area of high shear strains. Thus, for in-situ liquefaction and nonlinear soil testing, soil beneath the two shakers, in which the instrumentation is placed, can be excited in a nearly plane-strain condition. T-Rex and Raptor can also be used in tandem to create similar conditions in the vertical direction. Since T-Rex, Liquidator, and Rattler are not street-legal, the 26-wheel, tractor-trailer rig, called the Big Rig and shown in Figure 1F, is generally used to transport them to the test site.
Thumper (shown in Figure 1E) is the smallest shaker and is mounted on a street-legal truck and has a moderate force output, making it ideal for testing in urban areas. The maximum force output of Thumper in the vertical or horizontal directions is about 27 kN, as shown in Figure 2. With around 2 hours of work in the field, Thumper’s direction of shaking can be changed at the test site. It is important to note that hydraulic take-off connections are provided on T-Rex, Liquidator, and Thumper, which can be used to power other hydraulic equipment at the site. For example, these three vibroseis could be used at the test site to run linear hydraulic actuators for in-situ, pushover or pullout testing of superstructure and substructure subassemblages in the field (Stokoe, et al., 2017). Also, the hydraulic shakers mounted on the T-Rex, Liquidator, and Thumper vehicles can also be removed and mounted on a structure, while the hydraulics and electronics on the associated truck can be used to run the shaker. The selection of an appropriate mobile shaker depends primarily on the desired frequency range, sufficient force output, and shaking direction. For instance, if a researcher aims to image deeper areas with a wide range of sensor deployments, Liquidator is an optimal choice due to its ability to vibrate down to 0.7 Hz with a special configuration. On the other hand, if the goal is to study structural responses to different shaking directions, T-Rex is ideal, as it can switch shaking directions within seconds. Additional details regarding cost and operational support are available at https://utexas.designsafe-ci.org/.
NHERI@UTexas instrumentation and field support vehicles are shown in Figure 3. The supply truck, shown in Figure 3A, carries fuel and spare parts for the shaker trucks. Additionally, there is a customized Ford cargo van (shown in Figure 3B) and a 2.4 m by 4.8 m instrumentation trailer (shown in Figure 3A) that both provide an air-conditioned workspace, data acquisition systems, and electrical power. The NHERI@UTexas facility also has a significant amount of field instrumentation, including: (1) four primary data acquisition systems (discussed below), (2) 85, 1-Hz vertical geophones (Figure 3C), (3) 24, 1-Hz horizontal geophones, (4) 6 high-capacity dynamic load cells, (5) 18 triaxial MEMS accelerometers, (6) cone penetrometer test (CPT) equipment and seismic CPT equipment (Figure 3D), (7) 6 direct push crosshole sensors, (8) 10, 120-seconds and 10, 20-seconds Trillium Compact broadband seismometers (Figure 3F), (9) 100 SmartSolo IGU-16HR 3C nodal stations (Figure 3G), and (10) OptaSense ODH4 Distributed Acoustic Sensing (DAS) interrogator (Figure 3H).
The four main data acquisition systems are a 96-channel Data Physics spectrum analyzer system, a SmartSolo with integrated data acquisition system, 10 three-channel Nanometrics Taurus and 10 three-channel Nanometrics Centaur digitizers, and a DAS interrogator. The Data Physics system uses the SignalCal 730 software to generate input signals (sinusoidal, stepped-sine, white noise, frequency sweeps, etc.) that drive the mobile shakers and to record output signals from various sensors. The Data Physics system (shown in Figure 3E) consists of four dynamic signal analyzers, which have a total of 96 channels. The Data Physics analyzers can be set up as four separate units with different sampling rates, or they can be linked together as a single system. The Data Physics spectrum analyzers have the capacity to record data for hours of time at a high sampling rate up to 200 kHz. The Data Physics control software can also be used to perform real-time frequency domain calculations and display auto-power spectra, transfer functions, coherency, and phase plots to facilitate reviewing and analyzing data in the field.
For field studies requiring a distributed sensor array, with sensors spaced hundreds of meters to a kilometer apart, such as in passive surface wave testing or topographic amplification studies, the SmartSolo, Taurus, and Centaur digitizers are suitable options, as shown in Figures 3F, G. The SmartSolo is a cost-effective, all-in-one nodal system that integrates the sensor, data logger, and battery, making it highly portable and easy to deploy. In contrast, while the Taurus and Centaur digitizers are less portable due to the need for external sensors, they offer a higher dynamic range, more flexibility in sensor choices, and greater versatility in sampling rates.
The OptaSense ODH4+ DAS Interrogator system, shown in Figure 3H, utilizes advanced fiber optic technology to detect vibrations over long distances. In a DAS system, laser pulses travel through the fiber optic cable, and any disturbances or acoustic signals along the cable cause changes in the backscattered light, which are then recorded and analyzed. This effectively turns the fiber into thousands of individual sensors, capturing data at precise points along its length. The OptaSense ODH4+ DAS Interrogator can operate with various fiber types, including single-mode, multi-mode, and enhanced high-backscatter fibers, offering a sampling rate of up to 100 kHz and selectable gauge lengths from 2 to 32 m.
3 Establishing a test site near Austin, TX for validating methodologies or simulation models
The mobility features inherent in the NHERI@UTexas facility have enabled projects across a wide geographical range, both domestically and internationally. Domestically, the facility has supported projects around the United States, including Hawaii, as shown in Figure 4. Internationally, NHERI@UTexas has also conducted several projects in Taiwan, Canada, and New Zealand (See Figure 4). This mobility feature allows researchers to perform tests in complex and site-specific ground conditions or on permanent structures in various locations. For example, the T-Rex shaker was used to investigate the 3D magma structure of the Kilauea summit in Hawaii. Additionally, the facility has contributed to assessing liquefaction susceptibility at several sites in the states of Washington and Oregon, where liquefaction events have previously been recorded. The only drawback of an out-of-state Texas project is the cost of transporting the large shakers.
To support researchers in validating methodologies or simulation models using the large mobile shakers without high travel costs, NHERI@UTexas has established a local testing site, Hornsby Bend (HB), approximately 3.5 km north of the Austin-Bergstrom International Airport in Austin, Texas. The site is located away from buildings and infrastructure, making it optimal to conduct vibration-related testing. Additionally, the site is situated on an alluvial plain with relatively simple geology. CPT test results show the upper 8 m consist of sandy silt and clay, followed by 5 m of clayey sand or clayey gravel with sand, underlain by shale. Numerous tests have been performed at this site particularly near a fiber optic cable line equipped for Distributed Acoustic Sensing (DAS), as shown in Figure 5. In 2021, two types of fiber optic cables were installed, and subsequent data collected using DAS interrogators have been analyzed to assess the benefits of this emerging technology. Recent publications presenting these results are included in Abbas et al., 2024b, Yust et al., 2022b, and Yust et al., 2023. These studies demonstrate the efficacy of DAS in capturing high-resolution waveforms across long arrays. This capability not only enhances 2D and 3D subsurface imaging but also enables support to other applications, such as damping measurements. Extensive testing has been conducted at the HB site to compare DAS results with other methods, including thirteen CPTs, two triangular Microtremor Array Measurements (MAM), downhole seismic testing at two boreholes, and four direct push crosshole tests. Additionally, borehole logs are available for both boreholes, with P-wave reflection and electrical resistivity testing scheduled for November 2024. This comprehensive dataset is available through DesignSafe (Vantassel et al., 2022; Yust et al., 2022a) and provides an invaluable resource for researchers seeking diverse analytical opportunities, supported by robust ground-truth information.
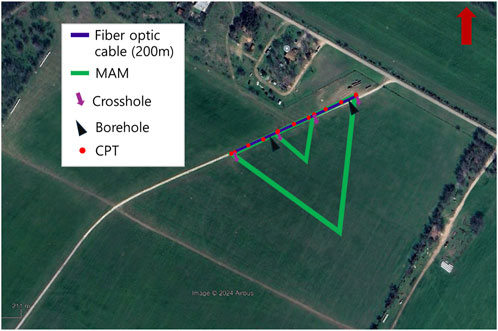
Figure 5. A satellite image of the Hornsby Bend (HB) site showing the various testing locations near the fiber optic cable line.
4 Key areas of investigation
The NHERI@UTexas facility is focused on four main challenges. These challenges are: (1) performing deeper, more accurate, higher resolution, 2D/3D subsurface geotechnical imaging through the use of cutting-edge equipment and analysis methods, (2) developing innovative testing techniques to propagate waves to greater depths for in situ characterization of the nonlinear dynamic response and liquefaction resistance of complex geomaterials, (3) developing rapid, in-situ methods for nondestructive structural evaluation and soil-foundation-structure interaction (SFSI) studies to better understand how structures respond to seismic and dynamic forces, and (4) conducting rapid investigation of geotechnical parameters at high-profile and natural hazard events for preventing secondary hazards and immediate risk assessment (after Stokoe et al., 2017). These challenges are substantial, yet the unique equipment resources at NHERI@UTexas are well-positioned to help researchers to address them. The progress achieved in each area over the past 10 years using NHERi@UTexas equipment is outlined, and advancements are described in the sections below.
4.1 Performing deeper, more accurate, higher resolution, 2D/3D subsurface imaging
The progress in non-invasive imaging has revolutionized healthcare, with technologies like X-rays, CAT scans, ultrasounds, and MRIs now an essential part of modern diagnostics. Despite ultrasound imaging only gaining popularity in the United States in the 1970s, it is now ubiquitous, with 3D color ultrasound imagery accessible in commercial settings. This trajectory suggests a promising parallel for subsurface imaging in civil engineering applications. Developing high-resolution, 3D images of the subsurface that include precise elastic properties, such as shear modulus, constrained modulus, and Poisson’s ratio, could fundamentally change the approach to designing resilient and sustainable infrastructure. The NHERI@UTexas facility is already equipped with the advanced technology necessary to drive this development, marking a crucial step toward in making this vision a reality.
In addition to enhancing civil infrastructure and geotechnical systems, NHERI@UTexas also supports improved seismic imaging of natural topography, such as magma systems beneath volcanoes. This capability can provide vital information for understanding geological processes and assessing natural hazards. As these imaging techniques continue to progress, their potential applications in environmental monitoring and resource management will grow substantially, fostering a future where sophisticated subsurface imaging becomes as accessible and essential as ultrasound technology is today. Although many examples exist, the focus in this article is on two sites where advanced subsurface imaging enhanced engineering research and practice: one site demonstrates 2D/3D imaging of geotechnical systems, and the other site is focused on natural topography.
4.1.1 Continuous 2D/3D in-situ profiling for anomaly detection in newberry, Florida
The comprehensive experiments and projects conducted over the last 10 years at a dry retention pond in Newberry, Florida, a site known for its karst cavities and subsurface anomalies, illustrate significant advancements in 2D and 3D subsurface imaging techniques. This work demonstrates the continuous support from NHERI@UTexas, emphasizing the facility’s commitment to enhancing our understanding of complex subsurface environments.
Tran and Hiltunen (2011) conducted surface wave testing at the Newberry site, but the study was confined to a smaller area and used less advanced equipment. The findings from the surface wave testing indicated that the subsurface is composed of medium-density fine sand and silt ranging in depth from 2 to 10 m overlying a highly variable limestone. Subsequently, Tran et al. (2013), Tran et al. (2020) applied full waveform inversion (FWI) techniques to detect subsurface anomalies. These studies utilized equipment from the NHERI@UTexas, including the uniaxial, small vibroseis called Thumper. In their 2013 study, Tran et al. employed 2D FWI and successfully identified an underground anomaly that was later confirmed to be a void through Standard-Penetration-Test (SPT) soundings. However, the predicted depth of the void was greater than its actual depth, which was attributed to the difference between the measured wavefield, and the assumed plane strain condition used in the 2D FWI. Building on this work, in 2020, Tran et al. expanded the FWI studies by exciting the ground at 65 locations within a 2D grid of 48 vertical geophones arranged in a 4 m × 12 m configuration, covering an area of 12 m × 36 m. The 3D FWI analysis resulted in a subsurface model identifying three voids, although only two of these were confirmed by SPT soundings. These earlier studies highlighted the challenges in accurately detecting and imaging subsurface anomalies using real field data, primarily due to limitations such as smaller spatial coverage, less dense spatial sampling, and the use of single-component sensors. While NHERI@UTexas supported the equipment, more advanced sources, such as the broadband T-Rex vibroseis shaker truck, were not utilized. Additionally, the presence of sinkholes of varying sizes and depths combine with some sinkholes filled with uncontrolled material, complicated the subsurface imaging efforts. These limitations highlighted the need for more comprehensive and technologically advanced approaches to subsurface imaging, particularly in complex karst environments like the Newberry site.
Our current study builds on this previous work, representing a significant advancement in experimental design and data collection. Supported by the U.S. National Science Foundation (Grant Numbers CMMI-2120155, CMMI-1930697, and CMMI-2037900), this experiment combined an extensive 2D DAS fiber-optic cable network with a dense array of three-component nodal seismometers. This setup is shown in Figure 6 and includes approximately 2 km of DAS cable deployed in a zigzag pattern, creating 1920 horizontal component channels, complemented by 144 three-component nodal seismometers arranged in a 12 × 12 grid. This configuration provides unprecedented spatial resolution over the 75 m × 155 m experimental area. In the experiment, the powerful T-Rex vibroseis truck is utilized as the active source for these measurements, exciting the ground at 260 distinct locations in three directions. Additionally, impact sources were also employed at 286 locations within the instrumented area, resulting in a total of 367 source positions. The shot locations are categorized as “Shots Inside” (SI) and “Shots Outside” (SO), with the SO positions further divided into south (SOS), west (SOW), and north (SON) locations. This strategic arrangement provides excellent spatial coverage and enables a wide range of measurements, offering unique opportunities for developing and testing advanced subsurface anomaly detection and imaging techniques (Abbas et al., 2024a). The integration of high-density DAS technology with traditional seismometers, along with the use of both active and passive sources, represents a significant leap in geophysical imaging capabilities. This study addresses crucial needs in earthquake engineering, geohazard assessment, and infrastructure development, particularly in complex karst environments like Newberry, Florida. By providing open-access data through DesignSafe (Abbas et al., 2023), this experiment is contributing significantly to the broader scientific community, facilitating further research and development in geophysical imaging techniques and paving the way for more accurate and higher-resolution 2D/3D subsurface geotechnical imaging.
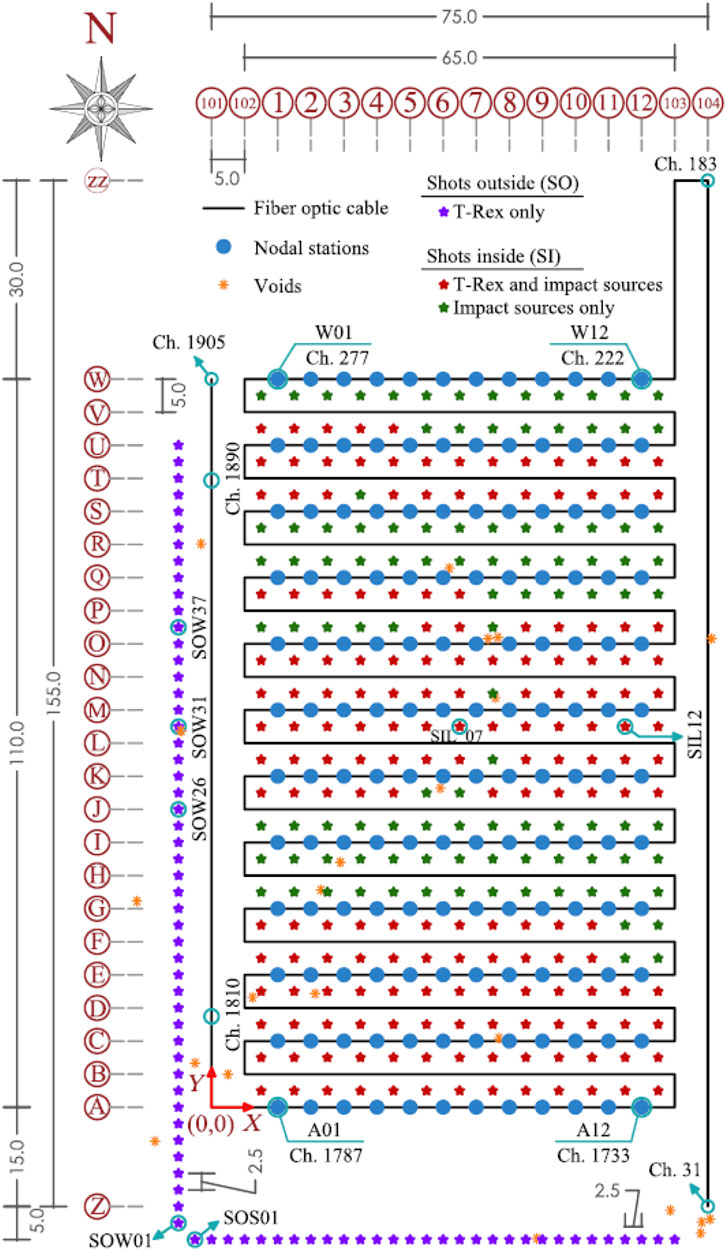
Figure 6. Schematic layout of the test site showing locations of the: 3C geophone nodal stations, fiber-optic cable, T-Rex and impact shots, and voids that are visible from the ground surface. The layout is comprehensive, including all the line numbers, letters, and dimensions in meters used to arrange the equipment (from Abbas et al., 2024a).
4.1.2 Imaging of structure and magma system beneath the summit of Kilauea Volcano
The Kīlauea volcano in Hawaii is one of the world’s most active volcanoes and has attracted scientific interest for centuries. Despite extensive research on various aspects of the volcano, a definitive understanding of the size and configuration of Kīlauea’s magmatic plumbing system remains elusive. Past estimates of the volumes of the magma system have varied significantly, ranging from 0.2 to 240 cubic kilometers (Decker, 1987; Denlinger, 1997; Fiske and Kinoshita, 1969; Pietruszka et al., 2015; Poland et al., 2014). These estimates have proposed differing storage systems, from disconnected dikes and sills to large subterranean bodies with established connections. The lack of size and configuration of the magmatic plumbing inhibits scientists’ ability to predict the volume and duration of eruptions, resulting in extensive damage to the infrastructure and nearly destroying 2,000 properties in May 2018. In response to the challenges posed by the 2018 eruption, Congress allocated supplemental funding to the U.S. Geological Survey (USGS) for rebuilding the Hawaiian Volcano Observatory and enhancing research capabilities. USGS proposed a passive imaging experiment and, in collaboration with academic partners, secured additional NSF funding for active source seismic imaging through the NSF project titled “Collaborative Research: Active and Passive Seismic Imaging of the Three-Dimensional Structure and Magma System beneath the Summit of Kilauea Volcano” (Award Number, EAR-2218645/2218646).
This project on the Kilauea summit marks the largest and most complex field experiment ever conducted on an active volcano, with more seismic nodes and a larger active source component than previous studies at sites like Yellowstone and Mount St. Helens. Approximately 1,815 seismometers were deployed across Kīlauea’s summit region, including areas affected by the 2018 caldera collapse. A central component of the experiment was the 34-ton triaxial vibroseis shaker truck called T-Rex supported by NEHRI@UTexas. In total, T-Rex shook at 396 locations around the summit of the Kilauea volcano. At 132 of these shot points, a 3-direction sweep was performed. At the rest of the 264 shot points, a one-direction sweep was performed. A photograph of T-Rex at a shot point on the summit of the Kilauea volcano is shown in Figure 7A. With data from both the T-Rex active source and natural seismicity recorded by the HVO and summit nodes, the researchers detected approximately 35,000 local earthquakes within 30 km of the center of Kīlauea, resulting in nearly 192 million waveforms for analysis. This extensive data set is enabling the creation of cross-sectional images of the volcano’s internal structure, similar to CT scans in medical imaging as shown in Figure 7B (Denlinger and Flinders, 2024). These images will gradually reveal the configuration of magma system, offering new insights into how Kīlauea stores and transports magma, feeds distant lava flows, and collapses at the summit. These findings will not only enhance scientific understanding of Kīlauea’s eruption mechanisms and magma storage but also provide valuable information for emergency managers, policymakers, and the public on the hazards posed by this evolving volcano system. Such insights are vital for informing future monitoring and response strategies, potentially minimizing the risks to nearby communities.
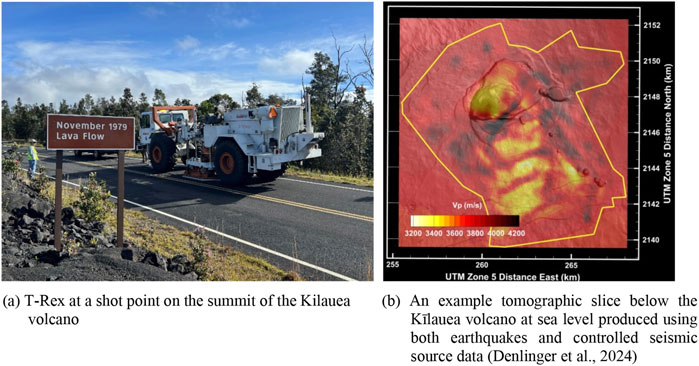
Figure 7. Seismic imaging of the three-dimensional structure and magma system beneath the summit of Kilauea volcano project: (A) T-Rex at a shot point on the summit of the Kilauea volcano. (B) An example tomographic slice below the Kīlauea volcano at sea level produced using both earthquakes and controlled seismic source data (Denlinger et al., 2024).
4.2 Characterizing the nonlinear dynamic response and liquefaction resistance of complex geomaterials in situ
Natural geotechnical materials, including soil and rock, play a critical role in the performance of our nation’s infrastructure during earthquakes and other natural hazards, such as hurricanes and floods. For example, the devastating effects of soil liquefaction and site amplification have been observed in essentially every significant earthquake. Additionally, the impact of geotechnical materials during hurricanes and floods is significant, often governed by compacted soils that form levees, dams, and dikes, as well as the underlying natural materials. The poor performance of levees during hurricanes can lead to extensive inundation, as evidenced by the failure of levees surrounding New Orleans, Louisiana, during Hurricane Katrina in 2005. Furthermore, global warming compounds these risks, intensifying the frequency and severity of extreme weather events. Rising temperatures, increased precipitation, and higher sea levels increases the susceptibility of certain soils to liquefaction, posing even greater challenges for earthquake-prone and flood-impacted areas. Unfortunately, natural geotechnical materials are the least investigated, most variable, and least controlled of all materials that form part of the U.S. infrastructure inventory (Coduto et al., 2015). Therefore, a significant challenge to making our infrastructure resilient and sustainable is characterizing the nonlinear dynamic response and liquefaction resistance of complex geomaterials in situ.
Nonlinear dynamic soil properties are required in predicting the response of geotechnical and structural systems during earthquakes and hurricanes. The key nonlinear properties include: (1) the variation of shear modulus (G) and material damping ratio in shear (D) with shear strain (γ), and (2) how these properties vary with soil type and number of cycles of loading. These properties are typically expressed as G-log γ and D-log γ relationships, as the shear strains induced during natural hazards can easily range over a factor of 1,000 (γ from below 0.001% to above 1.0%). Before the Network for Earthquake Engineering Simulation (NEES) and NHERI programs were funded by NSF, these dynamic soil properties could not be measured in the field due to the challenges of generating controlled, sinusoidal loading across a wide range of strains and number of loading cycles. Therefore, the field G-log γ and D-log γ relationships were empirically estimated by combining large-strain nonlinear measurements from small-scale dynamic laboratory testing of intact or reconstituted soil specimens with the limited, low-strain, field seismic testing that was available at that time.
4.2.1 In situ nonlinear measurement using a helical pile
Over the past 20 years, the NEES/NHERI@UTexas mobile shakers facility has pioneered and advanced a generalized, staged-loading approach to measuring the in-situ G-log γ and pore-water pressure-log γ relationships. This form of in situ parametric testing is essential for both understanding the limitations of empirical methods and for testing geotechnical materials that cannot be easily or cost-effectively assessed in the laboratory. The latest technique developed by NHERI@UTexas involves installing a helical pile to a depth of the target liquefiable soil layer, as shown in Figure 8A. One of the four vibroseises shown in Figure 1 and capable of controlled shaking in the vertical direction is positioned on top of the helical pile. Vertical waveforms generated by the vibroseis propagate directly into the soil at the desired testing depth. This approach overcomes limitations of conventional in-situ liquefaction testing, where the vibroseis excitation occurs at the ground surface. In traditional methods, rapid attenuation of cyclic strain with depth restricts the effective testing zone to approximately 2 m. With this new enhanced technique using the helical pile, cyclic strains at any depth can be achieved at levels of 0.3%–0.4%.
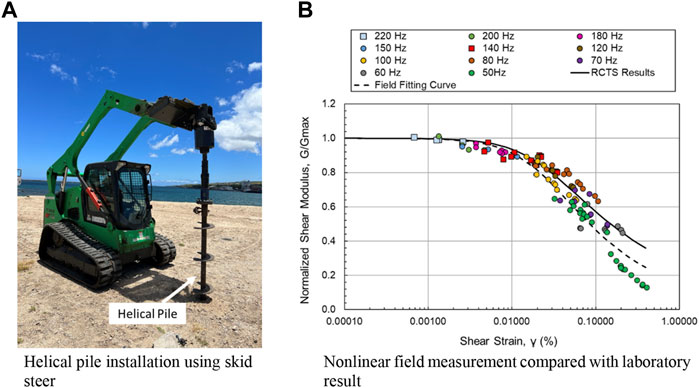
Figure 8. Nonlinear field measurements using the helical pile: (A) Helical pile installation using a skid steer and (B) Comparison of normalized shear modulus versus shear-strain relationship from field and laboratory tests.
Two NSF-funded projects are utilizing this pioneering liquefaction testing technique to measure nonlinear dynamic soil properties in situ. The first project, titled “RAPID/Collaborative Research: Investigating the Liquefaction Susceptibility of Calcareous Sand in Hawaii with an Enhanced NHERI@UTexas Large Mobile Shaker” (Award Numbers CMMI-2317659/2317660), focuses on studying the cyclic behavior of calcareous soils at the Kawaihae Harbor. Initial results indicate differences between field and laboratory data, likely due to limited resources on remote islands. For example, the low-capacity skid steer required for installing the helical pile may inadvertently disturb the soil. The second project, titled “Engineering Research Center for Bio-mediated and Bio-inspired Geotechnics” (Award Number EEC-1449501), examines the nonlinear properties of soils treated using the Microbially-Induced Desaturation (MID) method. Here, the nonlinear field measurements obtained from the testing pad are consistent with laboratory results, as shown in Figure 8B. The results from a depth of 2.95 m demonstrate the effectiveness of this enhanced testing technique, surpassing the limitations of conventional methods. The maximum strain level achieved at this depth reached 0.4%, a significant improvement in capturing in situ nonlinear soil behavior. These promising findings build upon the initial research conducted in Hawaii, which included improvements such as sensor array modifications, vibroseis adjustments, sensor recalibration, and crosshole sensor installations to ensure high-quality data. Insights from these projects have contributed valuable knowledge for evaluating and mitigating earthquake hazards in liquefaction-prone areas.
4.3 Developing rapid, in situ methods for nondestructive structural evaluation and soil-foundation-structure interaction (SFSI) studies
The NHERI@UTexas facility also offers equipment uniquely capable of conducting in-situ testing on structural engineering systems. Unlike the vast majority of structural engineering experimental research that comprises quasi-static, pseudo-dynamic, or shake table tests to characterize structural performance under idealized boundary conditions, NHERI@UTexas’s equipment enables the examination of complex soil-foundation-structure interaction (SFSI) behaviors in real-world settings. Traditional experimental research on SFSI often involves on small-scale models (scaled 1:30 to 1:100) tested in uniform soil containers on shake tables or in centrifuges, which may not accurately represent construction materials, methods, or varied soil conditions. While scaled laboratory studies are valuable, NHERI@UTexas facilitates a wide range in field testing that incorporates a range of soil environments, offering a realistic approach to understanding SFSI in full-scale infrastructure. The NHERI@UTexas shakers enable multiple testing approaches, including indirect excitation of the structure by shaking the surrounding soil, direct excitation by placing the shaker mechanism on or attaching it directly to the structure, and quasi-static testing methods in situ. Originally designed for geotechnical applications, the shakers generate maximum force outputs at relatively high frequencies, which may limit their ability to induce nonlinear or damaging responses in large-scale structures. For testing where nonlinear behavior is essential, smaller-scale structural specimens can be designed to align with force and frequency capacities of the mobile shakers.
4.3.1 Seismic isolation of embedded foundations using periodic barriers
An NSF-funded project titled “Collaborative Research: Seismic Isolation of Embedded Foundations using Periodic Barriers to Create Resilient Structures” (Award Number CMMI-1761597) is an example of using the mobile shakers for direct and indirect dynamic testing of a soil-foundation-structure system in the field. The aim of this research effort is to develop a periodic barrier capable of effectively attenuating incoming seismic waves. The project goals include: (1) deriving the analytical solution of the Rayleigh-wave frequency band gap of periodic metamaterial, (2) conducting passive isolation tests and active isolation tests to evaluate the performance of the barriers, and (3) establishing a finite element model for a comprehensive parametric study of periodic barriers. Field testing is being performed to examine the feasibility of the periodic barrier for seismic isolation, facilitated by NHERI@UTexas’s state-of-the-art equipment. The T-Rex mobile shaker is being used to provide surface wave excitation in three directions—vertical, horizontal crossline, and horizontal inline—while an array of surface sensors from NHERI@UTexas are being used to record real-time ground and structural responses as shown in Figure 9. Effectiveness of the periodic foundation and the periodic barriers were determined based on the motions recorded with these sensors.
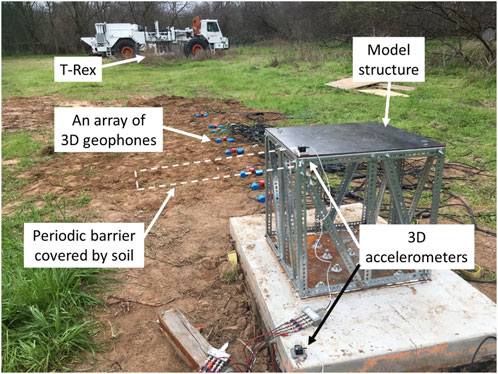
Figure 9. A photograph of T-Rex used as a vibrational source in passive isolation tests with a model structure accompanied by arrays of 3D geophones and 3D accelerometers.
Key findings from this study (Zhang et al., 2022; Wang et al., 2022; Huang et al., 2020; Huang et al., 2021) demonstrate the vibration mitigation potential of periodic barriers and foundations, as well as the impact of various excitation methods and directions of motion on the screening performance. In Figure 10, the results of the field tests with T-Rex show significant vibration reduction across multiple frequencies and directions, which ambient vibrations alone would not reveal. The advanced excitation capabilities of T-Rex provided a comprehensive view of the total system, revealing the broad frequency-band gaps achieved by combining periodic barriers and foundations. Numerical simulations, primarily using 2D finite element models, showed good agreement with the field experiments, validating their use in predicting and optimizing the barrier performance. While these test programs successfully demonstrated the effectiveness of metamaterial-based isolation systems for both active and passive vibration mitigation, further in-situ field testing of complex systems is necessary to better address research needs related to broadband vibration control and optimization of barrier-foundation systems for various applications.
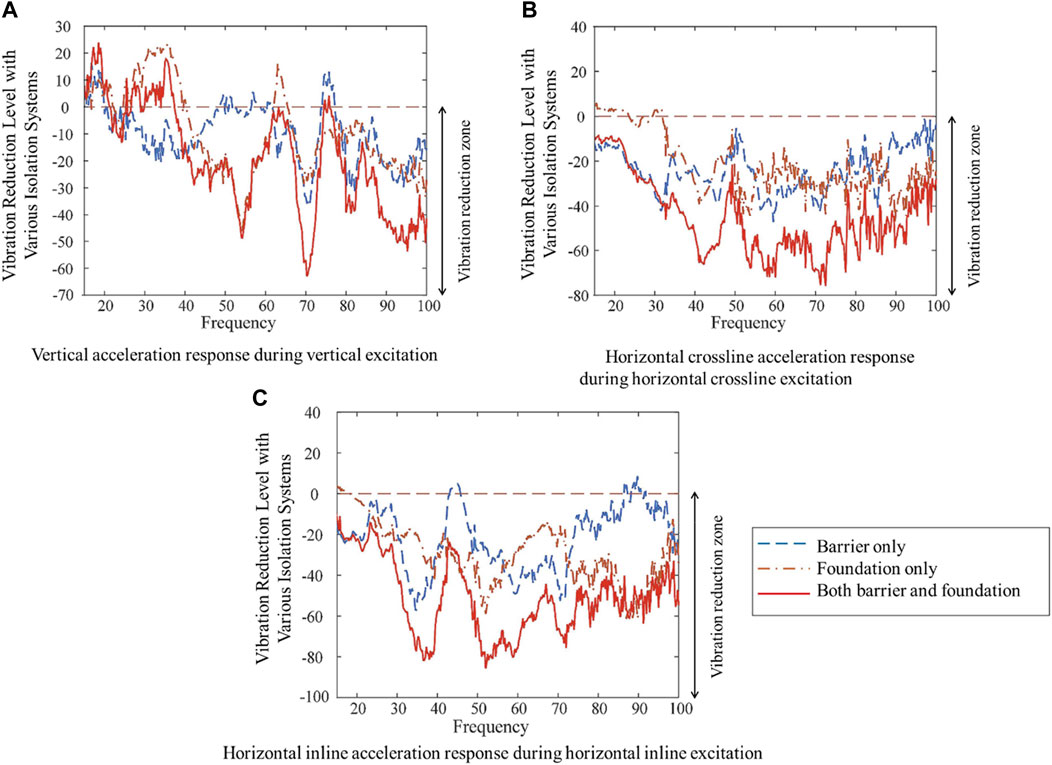
Figure 10. Vibration reduction level of the wave isolation system composed of the periodic barrier and periodic foundation obtained from seismic excitation tests in all three directions generated by T-Rex (Zhang et al., 2022). (A) Vertical acceleration response during vertical excitation. (B) Horizontal crossline acceleration response during horizontal crossline excitation. (C) Horizontal inline acceleration response during horizontal inline excitation.
4.4 Rapid field investigation of geotechnical parameters at high-profile and natural hazard events
The mobile capability of the NHERI@UTexas facility can be leveraged to rapidly investigate geotechnical parameters after devastating natural or man-made hazards. An example of this rapid field response is the investigation of the Champlain Towers South collapse, a project funded by NIST through the NSF NHERI program. In collaboration with Utah State University, NHERI@UTexas utilized an urban vibroseis called, Thumper, to assess the foundation and surrounding soil conditions with minimal disturbance to the site. Given the high-profile nature of the Champlain Towers South investigation, data publication is restricted at this time.
5 Summary
The specialized, mobile field equipment available at the NHERI@UTexas facility for dynamically and cyclically loading the natural and built environments is discussed in this article and examples are presented. This facility offers researchers worldwide access, under the NSF NHERI shared-use policy, to five large, hydraulically controlled shakers, a tractor-trailer for transporting the largest shakers, field-support vehicles, and an extensive array of field instrumentation and sensors. The NHERI@UTexas facility targets four main challenges: (1) performing deeper, more accurate, higher resolution 2D/3D subsurface geotechnical imaging, (2) characterizing the nonlinear dynamic response and liquefaction resistance of complex geomaterials in situ, (3) developing in-situ methods to perform nondestructive soil-foundation-structure interaction (SFSI) studies, and (4) rapid investigation of geotechnical parameters at high-profile and natural hazard events (after Stokoe et al., 2017). In this article, examples of how this unique equipment has been applied to support the goals of numerous researchers and also outlines the enhancements made to expand field testing capabilities.
Data availability statement
The original contributions presented in the study are included in the article/supplementary material, further inquiries can be directed to the corresponding authors.
Author contributions
KS: Writing–original draft, Writing–review and editing, Funding acquisition, Project administration, Resources, Supervision. SH: Data curation, Formal Analysis, Project administration, Validation, Visualization, Writing–original draft, Writing–review and editing. BC: Data curation, Methodology, Project administration, Writing–original draft, Writing–review and editing. PC: Methodology, Writing–original draft, Writing–review and editing. RG: Methodology, Writing–original draft, Writing–review and editing.
Funding
The author(s) declare that financial support was received for the research, authorship, and/or publication of this article. The U.S. National Science Foundation financially supports the development and operation of the NHERI@UTexas Equipment Sites under grant CMMI-2037900. The research projects included in this paper were funded by grants CMMI-2120155, CMMI-1930697, CMMI-2100889, EAR-2218645/2218646, CMMI-2317659/2317660, EEC-1449501, and CMMI-1761597.
Acknowledgments
The authors would like to thank the U. S. National Science Foundation for the financial support to develop and operate the NHERI@UTexas Equipment Sites under grant CMMI-2037900. The authors also would like to thank the following researchers who utilized the NHERI@UTexas equipment facility including Drs. Arash Khosravifar, Armin Stuedlein, Diane Moug, Clinton Wood, Edward Kavazanjian, Khiem Tran, Roger Denlinger, Jyotsna Sharma, Leon van Paassen, Susan Bilek, Joel Johnson, Daniel Cadol, Danica Roth, and Nenad Gucunski. Special thanks also go to the graduate students and staff members at the University of Texas at Austin who assisted in the field work, including Drs. Benchen Zhang, Julia Roberts, Gunwoong Kim, Reihaneh Hosseini, Zhongze Xu, Farnyuh Menq, Jihwan Lee, Chenghao Bai, Cecil Hoffpauir, Andrew Valentine, Elido Ruiz, and Robert Kent.
Conflict of interest
The authors declare that the research was conducted in the absence of any commercial or financial relationships that could be construed as a potential conflict of interest.
Generative AI statement
The author(s) declare that Gen AI was used in the creation of this manuscript.
Publisher’s note
All claims expressed in this article are solely those of the authors and do not necessarily represent those of their affiliated organizations, or those of the publisher, the editors and the reviewers. Any product that may be evaluated in this article, or claim that may be made by its manufacturer, is not guaranteed or endorsed by the publisher.
References
Abbas, A., Cox, B. R., Tran, K. T., Corey, I., and Dawadi, N. (2024a). An open-access data set of active-source and passive-wavefield DAS and nodal seismometer measurements at the Newberry Florida site. Seismol. Res. Lett. 95, 1082–1098. doi:10.1785/0220230216
Abbas, A., Cox, B. R., Tran, K. T., Corey, I., Dawadi, N., and Menq, F. (2023). Active-source and passive-wavefield DAS and nodal station measurements at the Newberry Florida site. DesignSafe-CI. doi:10.17603/ds2-50eh-7v93
Abbas, A., Cox, B. R., Tran, K. T., Kumar, K., and Crocker, J. (2024b). Emerging technologies and advanced analyses for non-invasive near-surface site characterization. Soils and Rock. Int. J. Geotechnical Environ. Eng. doi:10.28927/SR.2024.006923
Coduto, D. P., Kitch, W. A., and Yeung, M. R. (2015). Foundation design: principles and practices. 3rd Edition. Upper Saddle River, NJ: Prentice Hall.
Decker, R. W. (1987). “Dynamics of Hawaiian volcanoes: an overview,” in Volcanism in Hawaii: papers to commemorate the 75th anniversary of the founding of the Hawaiian Volcano Observatory (Reston, VA: U.S. Geol. Surv. Prof. Pap.),1350, 997–1018.
Denlinger, R., O’Connell, D. R. H., Lin, G., Roecker, S., and Bennington, N. (2024). An unprecedented experiment to map Kīlauea’s summit magma system. Eos 105. doi:10.1029/2024EO240392
Denlinger, R. P. (1997). A dynamic balance between magma supply and eruption rate at Kīlauea volcano, Hawaii. J. Geophys. Res. 102 (18), 18091–18100. doi:10.1029/97JB01071
Denlinger, R. P., and Flinders, A. (2024). Density structure of Kīlauea volcano: implications for magma storage and transport. Geophys. J. Int. 237 (1), 1339–1352. doi:10.1093/gji/ggae101
Fiske, R. S., and Kinoshita, W. T. (1969). Inflation of Kīlauea volcano prior to its 1967-1968 eruption: vertical and horizontal deformation give clues regarding the structure of an active Hawaiian volcano. Science 165, 341–349. doi:10.1126/science.165.3891.341
Huang, H. W., Wang, J., Zhao, C., and Mo, Y. L. (2020). Two-dimensional finite-element simulation of periodic barriers. J. Eng. Mech. 165, 147. doi:10.1061/(asce)em.1943-7889.0001891
Huang, H. W., Zhang, B., Wang, J., Menq, F. Y., Nakshatrala, K. B., Mo, Y. L., et al. (2021). Experimental study on wave isolation performance of periodic barriers. Soil Dyn. Earthquake Eng. 144, 106602. doi:10.1016/j.soildyn.2021.106602
Pietruszka, A. J., Heaton, D. E., Marske, J. P., and Garcia, M. O. (2015). Two magma bodies beneath the summit of Kīlauea Volcano unveiled by isotopically distinct melt deliveries from the mantle. Earth Planet. Sci. Lett. 413, 90–100. doi:10.1016/j.epsl.2014.12.040
Poland, M. P., Miklius, A., and Montgomery-Brown, E. K. (2014). “Magma supply, storage, and transport at shield-stage Hawaiian volcanoes,” in Characteristics of Hawaiian volcanoes (Reston, VA: U.S. Geol. Surv. Prof. Pap.), 1801, 179–234. doi:10.3133/pp18015
Stokoe, K. H., Cox, B., Clayton, P., and Menq, F. (2017). NHERI@UTEXAS experimental facility: large-scale mobile shakers for natural-hazards field studies. Santiago: Chile: 16th World Conference on Earthquake.
Stokoe, K. H., Hwang, S., Cox, B. R., Menq, F., Roberts, J., and Park, K. (2019). “Field studies of the natural and built environments using large mobile shakers,” in 7th international conference on earthquake geotechnical engineering (7 ICEGE) (Roma: Italy), 17–20.
Stokoe, K. H., Menq, F., Zhang, B., and Kim, G. (2020). “Field assessment of microbially-induced carbonate precipitation (MICP) and microbially-induced desaturation and precipitation (MIDP) methods,” in Geotechnical engineering report GR20-01, geotechnical engineering center (Austin, TX: the University of Texas at Austin).
Tran, K. T., and Hiltunen, D. R. (2011). Inversion of first-arrival time using simulated annealing. J. Environ. Eng. Geophys 16, 25–35. doi:10.2113/JEEG16.1.25
Tran, K. T., McVay, M., Faraone, M., and Horhota, D. (2013). Sinkhole detection using 2D full seismic waveform tomography. Geophysics 78 (5), R175–R183. doi:10.1190/geo2013-0063.1
Tran, K. T., Nguyen, T. D., Hiltunen, D. R., Stokoe, K. H., and Menq, F. (2020). 3D full-waveform inversion in time-frequency domain: field data application. J. Appl. Geophys. 178, 104078. doi:10.1016/j.jappgeo.2020.104078
Vantassel, J. P., Cox, B. R., Hubbard, P. G., Yust, M. B. S., and Menq, F. (2022). “Active-source, near-surface, surface-wave measurements using distributed acoustic sensing (DAS) and traditional geophones,” in Characterization of the NHERI@UTexas Hornsby Bend test site [version 2]. DesignSafe-CI. doi:10.17603/ds2-bz52-ep82
Wang, J., Huang, H. W., Zhang, B., Menq, F.-Y., Nakshatrala, K. B., Mo, Y. L., et al. (2022). Active isolation tests of metamaterial-based barriers and foundation. Eng. Struct. 260, 114253. doi:10.1016/j.engstruct.2022.114253
Yust, M. B. S., Cox, B. R., Menq, F., Hubbard, P. G., and Vantassel, J. P. (2022a). “Active-source, near-surface, surface-wave measurements using distributed acoustic sensing (DAS), cone penetration testing (CPT), and downhole (DH) testing,” in Characterization of the NHERI@UTexas Hornsby Bend test site [version 2]. DesignSafe-CI. doi:10.17603/ds2-6ap5-sk09
Yust, M. B. S., Cox, B. R., Vantassel, J. P., Hubbard, P. G., Boehm, C., and Krischer, L. (2022b). DAS for 2D MASW imaging: a case study on the benefits of flexible sub-array processing. arXiv 2210, 14261. doi:10.48550/arXiv.2210.1426
Yust, M. B. S., Cox, B. R., Vantassel, J. P., Hubbard, P. G., Boehm, C., and Krischer, L. (2023). Near-surface 2D imaging via FWI of DAS data: an examination on the impacts of FWI starting model. Geosciences 13 (3), 63. doi:10.3390/geosciences13030063
Keywords: NHERI@UTexas, large mobile shakers, in-situ seismic testing, subsurface imaging, liquefaction testing, soil-structure interaction
Citation: Stokoe KH, Hwang S, Cox BR, Clayton PM and Gilbert RB (2025) NHERI@UTexas experimental facility with large-scale mobile shakers for field studies: a decade of discovery in natural hazards engineering. Front. Built Environ. 10:1524030. doi: 10.3389/fbuil.2024.1524030
Received: 06 November 2024; Accepted: 04 December 2024;
Published: 07 January 2025.
Edited by:
Jack Cadigan, Engineer Research and Development Center (ERDC), United StatesReviewed by:
Filippo Gatti, Université Paris-Saclay, FranceLiangjie Qi, Xi’an University of Architecture and Technology, China
Copyright © 2025 Stokoe, Hwang, Cox, Clayton and Gilbert. This is an open-access article distributed under the terms of the Creative Commons Attribution License (CC BY). The use, distribution or reproduction in other forums is permitted, provided the original author(s) and the copyright owner(s) are credited and that the original publication in this journal is cited, in accordance with accepted academic practice. No use, distribution or reproduction is permitted which does not comply with these terms.
*Correspondence: Kenneth H. Stokoe, ay5zdG9rb2VAbWFpbC51dGV4YXMuZWR1; Sungmoon Hwang, c3VuZ21vb25AdXRleGFzLmVkdQ==