- Department of Chemical Engineering, School of Chemistry and Chemical Engineering, Chongqing University, Chongqing, China
Succinic acid (SA), one of the 12 top platform chemicals produced from biomass, is a precursor of various high value-added derivatives. Specially, 1 mol CO2 is assimilated in 1 mol SA biosynthetic route under anaerobic conditions, which helps to achieve carbon reduction goals. In this review, methods for enhanced CO2 fixation in SA production and utilization of waste biomass for SA production are reviewed. Bioelectrochemical and bioreactor coupling systems constructed with off-gas reutilization to capture CO2 more efficiently were highlighted. In addition, the techno-economic analysis and carbon sequestration benefits for the synthesis of bio-based SA from CO2 and waste biomass are analyzed. Finally, a droplet microfluidics-based high-throughput screening technique applied to the future bioproduction of SA is proposed as a promising approach.
1 Introduction
Succinic acid (SA) is normally an intermediate metabolite of the tricarboxylic acid (TCA) cycle. As a C4 platform chemical, SA is the precursor for the synthesis of a variety of high value-added chemicals or materials, such as 1,4-butanediol (1,4-BDO), γ-butyrolactone, tetrahydrofuran, adipic acid, N-methyl pyrrolidone, and linear aliphatic esters (Kang et al., 2014; Tapin et al., 2020; Le and Nishimura, 2021). The most notable application of SA is that it could be used as a monomer for the production of biodegradable polybutylene succinate (PBS), which has a market value of $110 million (Amulya and Venkata Mohan, 2022). Therefore, the market demand for SA is huge. Traditionally, SA is chemically synthesized by maleic anhydride from petrochemical feedstocks, and Ni- or Pd-based catalysts have always been used to hydrogenate maleic anhydride into SA (Geyer et al., 2017; Zhuang et al., 2023). Despite the high conversion rate, there are still many drawbacks, which include complex operations, expensive catalysts, and serious pollution (Louasté and Eloutassi, 2020; Yao Zhang, 2020). Additionally, petroleum resources are non-renewable, and the burning of fossil fuels emits huge amounts of greenhouse gases, especially CO2, which causes a detrimental global warming (Martins et al., 2021). At the end of 2020, approximately 34.8 billion tons of CO2 were emitted from fossil fuels (Karakurt and Aydin, 2023). As a consequence, many scientists worldwide are endeavoring to explore green biosynthetic methods to obtain SA from waste and renewable feedstocks. Among these strategies, microbial fermentation for SA with utilization of CO2 and waste biomass as feedstocks is considered the most advantageous technology due to its simple biological operating conditions, mild enzyme-catalyzed process, and efficient CO2 fixation capacity. Meanwhile, CO2 biosequestration by microorganisms contributes to net zero emissions and is beneficial to obtaining carbon sequestration benefits (Ma et al., 2022; Song et al., 2024).
The biosynthesis of SA opens a novel pathway to utilize the greenhouse gas. Under anaerobic conditions, many wild-type strains, such as Actinobacillus succinogenes (Putri et al., 2020), Saccharomyces cerevisiae (Yan et al., 2014), Yarrowia lipolytica (Li et al., 2022), and Escherichia coli (Olajuyin et al., 2019), could utilize CO2 under the action of enzymes and accumulate SA (Priyadharsini et al., 2022). Theoretically, for 1 g of SA produced, 0.373 g of CO2 could be fixed (Amulya and Venkata Mohan, 2022). Thus, bio-SA is actually a kind of iconic CO2 fixation product through biomanufacturing, which helps human society to reach its global CO2 emissions reduction target (Belbute and Pereira, 2020). Furthermore, SA productivity could be enhanced by metabolic engineering or synthetic biology strategies, which also lead to increased CO2 fixation rates (CFRs) and higher carbon sequestration benefits. Moreover, the raw feedstocks for biosynthesis are less costly and more easily available than those for chemical synthesis. Renewable biomass resources, especially waste biomass such as sugarcane bagasse (Xu et al., 2021), corncob (Zhao et al., 2016), cassava root, etc (Thuy et al., 2017), are potential feedstocks for the production of SA and the reduction of carbon emissions. When compared to fossil-fuel-based SA, each ton of bio-based SA produced by biomass is predicted to reduce CO2 emissions by 4.50–5.00 tons (Gunnarsson et al., 2014).
In summary, the biosynthesis of SA can use CO2 and waste biomass hydrolysate to achieve higher carbon sequestration benefits and lower production costs, showing excellent environmental and economic benefits. This article reviews the current advances in CO2 fixation and waste biomass utilization during SA biosynthesis for carbon sequestration benefits. The extensive sources for SA fermentation and derivative products are shown in Figure 1. And the importance and possible improvements of efficient applied potential systems constructed by bioelectrochemical strategy and off-gas utilization through coupling bioreactors are summarized. In addition, the techno-economic analysis and the evaluation of carbon sequestration benefits for SA biorefineries are presented, which is conducive to advancing the industrialization of SA biosynthesis. Finally, a droplet microfluidics-based high-throughput screening technique applied to the future bioproduction of SA is proposed as a promising approach.
2 Enhanced CO2 fixation in SA biosynthesis
To date, a variety of natural or artificial SA biosynthesis pathways have been reported, of which four major metabolic pathways are often adopted, as shown in Figure 2 (Liu et al., 2022): 1) reductive TCA cycle; 2) oxidative TCA cycle; 3) the glyoxylate pathway; 4) the 3-hydroxypropionate cycle (3HP cycle) (Sanchez et al., 2005; Ahn et al., 2016; Vuoristo et al., 2016). The reductive TCA cycle is used by prokaryotes, such as E. coli, to produce SA and utilize CO2 (Choi et al., 2022). The beneficial effects of CO2 fixation and SA biosynthesis are reciprocal. On the one hand, CO2 can be fixed by multiple enzymes during the pathway of SA production, such as phosphoenolpyruvate carboxylase (PEPC), phosphoenolpyruvate carboxykinase (PCK), pyruvate carboxylase (PYC), malic acid enzyme (MAE), and so on. On the other hand, CO2 supply conditions also influence SA productivity and yield. In this section, the functions of enzymes involved in CO2 fixation during the production of SA are summarized, and the metabolic engineering strategies for the construction of engineered SA-producing strains are discussed. The increased benefits of microbial carbon sequestration are reflected in increased CFRs. As shown in Table 1, the efficiency of different pathways for immobilizing CO2 in the production of SA was listed.
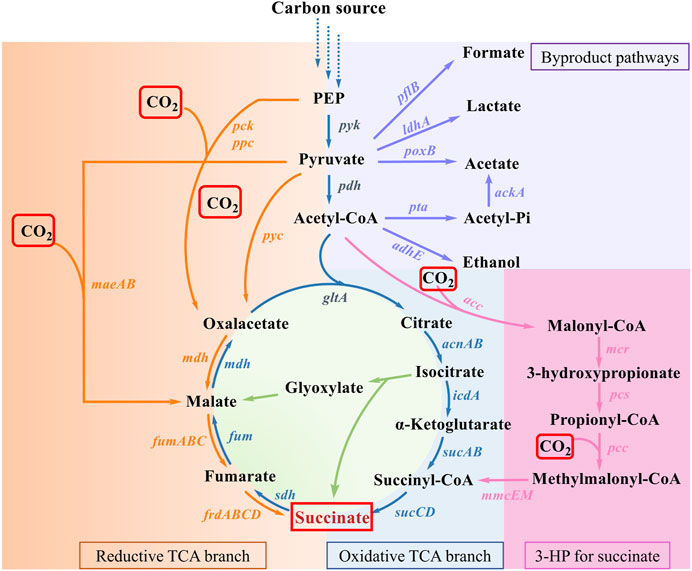
Figure 2. SA production pathways in microorganism. Genes of enzymes related to the pathways are presented next to the arrows, and each pathway is highlighted in a distinct color. Virtually, CO2 fixation occurs in reductive TCA branch and 3-HP for succinate. Abbreviations and genes: PEP, phosphoenolpyruvate; ackA, acetate kinase; acnAB, aconitase; adhE, alcohol dehydrogenase; gltA, citrate synthetase; icdA, isocitrate dehydrogenase; ldhA, lactic dehydrogenase; maeAB, malic enzyme; mdh, malate dehydrogenase; pck, PEP carboxykinase; pflB, pyruvate formatelyase; poxB, pyruvate oxidase; ppc, PEP carboxylase; pta, phosphotransacetylase; pyc, pyruvate carboxylase; sdh, succinate dehydrogenase; sucABCD, succinyl-CoA synthetase; acc, acetyl-CoA carboxylase; mcr, malonyl-CoA reductase; pcs, propionyl-CoA synthase; pcc, propionyl-CoA carboxylase; mmcEM, methylmalonyl-CoA epimerase and mutase; fumABC, fumarate hydratase; frdABCD, succinate dehydrogenase.
It could be concluded that the main factors influencing the CO2 fixation rates and SA yield are as follows: the direction of central carbon metabolic flow, the activity of key enzymes in the reductive branch of TCA cycle, the solubility of CO2, and the assistance of transporters and cofactors.
The knockout of genes associated with by-product production is an effective strategy to reduce byproduct accumulation and increase the flow of central carbon metabolism to the reductive TCA cycle, which utilizes CO2. For instance, knockout of genes encoding lactate dehydrogenase (ldhA), pyruvate formate lyase (pflB), phosphotransacetylase (pta), and acetate kinase (ackA), all of which are effective in increasing SA production and CO2 fixation rates (Chatterjee et al., 2001; Jiang et al., 2010). This type of engineered strain, with relevant gene deletions in the by-product pathways, laid the foundation for the subsequent increase in SA production and CFRs.
Along with the endeavor to eliminate by-products, the identification and enhancement of the key enzymes involved in the reductive branch of the TCA cycle, such as malate dehydrogenase (MDH) and PYC, were also investigated (Olajuyin et al., 2019; Ahn et al., 2020). The conversion of OAA to malate, catalyzed by MDH under anaerobic conditions, is a crucial step for SA biosynthesis (Valle et al., 2021). The catalytic characteristics of MDH from different sources were investigated, and the optimal one, Corynebacterium glutamicum MDH (CgMDH), was introduced into Mannheimia succiniciproducens to develop a high-performance strain for SA production. The strain expressing cgmdh produces 134 g/L of SA, with a maximum CFR of 7.95 g/L/h, in a high-inoculum fed-batch fermentation, proving the importance of pathway reconstruction in strain development (Ahn et al., 2020). By selecting the gene of the key enzyme with higher enzyme activity and introducing it into the engineered strain, the yield of SA could be further improved.
The improvement of comprehensive metabolic performance usually requires the regulation of multiple metabolic pathways, and the most commonly used method is multi-enzyme co-expression. Overexpression of carbonic anhydrase (CA) and PEPC in E. coli DC1515 (ΔpflA, ΔldhA, and ΔptsG400) increased SA production from 0.750 to 16.3 g/L, and CFR increased 21.8-fold, which was 0.127 g/L/h (Wang et al., 2015; Huang et al., 2019). Additionally, overexpression of CA and PCK in Corynebacterium acetoacidophilum (ΔldhA) increased SA production from 24.0 to 27.9 g/L and CFR from 0.299 to 0.347 g/L/h (Qian and Zheng, 2023). Notably, the direct substrate of carboxylases is not CO2 but HCO3− (Craveiro et al., 2022; Zaidi et al., 2022). CA could accelerate the conversion of CO2 to bicarbonate, so its expression could increase the solubilization of CO2 in intracellular fluids so that enzymes such as PEPC or PCK could make better use of HCO3−. Although multi-enzyme co-expression is a successful strategy for SA production and CO2 utilization, the compatibility of each enzyme needs to be considered in practical applications (Wang et al., 2015; Hou et al., 2019).
Furthermore, the production of SA was also affected by global regulatory factors, NADH/NAD+ ratio, and ATP level. Although the inactivation of pfl and ldh enhances the conversion of PEP to OAA, it also restricts the regeneration of NAD+ from NADH produced during glycolysis and causes an excessive buildup of pyruvate. Nicotinic acid phosphoribosyltransferase (NAPRTase) is a rate-limiting enzyme of the NAD(H) synthesis system, and the overexpression of NAPRTase is conducive to the generation of NAD+ (Marletta et al., 2015). Therefore, the co-expression of NAPRTase and PYC in pflB, ldhA, and ppc deletion strains can reduce NADH/NAD+ ratio and redistribute the carbon flux. It resulted in a production of 12.1 g/L of SA and a CFR of 0.0835 g/L/h under anaerobic conditions (Liu et al., 2013). NAD+ could serve as an electron acceptor during anaerobic fermentation, while excessive energy will burden metabolism (Zhang et al., 2016). Li et al. overexpressed the soluble fumarate reductase from S. cerevisiae to regulate the NADH/NAD+ ratio and ATP level, and the SA yield reached 31.9 g/L, an increase of 39.0% compared to the control (Li J. et al., 2017). It has been proven that Cra (catabolite repressor/activator) vitally participates in the global regulation of carbon metabolism-related genes (Ishihama, 2010). Cra activates genes involved in PEP carboxylation and suppresses genes associated with glycolytic pathways (Cozzone and El-Mansi, 2005). Zhu et al. firstly constructed random mutagenesis libraries of cra by error-prone PCR and then determined the relative activity of key enzymes involved in SA metabolism. After integrating mutation sites, the optimal mutant strain finally produced SA 79.8 g/L (Zhu et al., 2016). Recently, reconfiguration of the reductive SA biosynthesis pathway in mitochondria by coupling oxidative and reductive TCA cycles for NADH regeneration was reported in the strictly aerobic yeast Yarrowia lipolytica, which produced 112 g/L SA in 62 h by batch replenishment fermentation at low pH in a yield of 0.790 g/g glucose (Cui et al., 2023).
During the process of SA synthesis, transporters responsible for C4-dicarboxylates transport could increase the metabolic flux and SA yield. Li et al. employed three SA transporters from different organisms in E. coli AFP111 to construct three engineered strains, and the maximum SA production could reach 68.7 g/L (Li X. et al., 2017). The results demonstrated that the efflux capacity of the engineered strain was still strong under a high concentration of SA. By overexpressing mgtA, which encodes the magnesium transporter, Wang et al. constructed an engineered E. coli strain to produce SA with MgCO3 or Mg(OH)2 as an alkaline neutralizer in fermentation. The final fermentation yield was increased to 0.860 g/g total sugar of SA with a CFR of 0.802 g/L/h (Wang J. et al., 2014). Mg2+ is an essential ion for numerous physiological processes, but the molecular mechanisms of Mg2+ channel and transporter regulation that maintain Mg2+ homeostasis are unclear (Tomita et al., 2017). Additionally, MgCO3 could also provide HCO3− to carboxylate PEP and maintain pH in the fermentation broth.
Although the regulation of the SA metabolism process is very complex, many factors could be considered to balance the metabolic process when the strains are modified to achieve efficient production of SA. As is shown in Table 2, the performance of engineered strains constructed by metabolic engineering to produce SA and fix CO2 is summarized.
3 Production of SA from waste biomass
As a renewable feedstock for the production of SA, the potential that biomass shows for carbon sequestration is also often discussed (Forfora et al., 2024). When compared to fossil-fuel-based chemicals, bio-based chemicals produced by biomass are predicted to reduce CO2 emissions (Hepburn et al., 2019). The hydrolysate of biomass also provides the feedstock for microbial capture, fixation, and sequestration of carbon dioxide. It usually requires three stages to produce SA from waste biomass. Firstly, the biomass needs to be pretreated to break its difficult-to-hydrolyze structure, and then the pre-treated biomass is enzymatically hydrolyzed to fermentable sugars, which could finally be fermented to SA (Narisetty et al., 2022; Pakchamni et al., 2022). Pretreated biomass could be used to produce SA by separate hydrolysis and fermentation (SHF) or by one-pot reaction methods such as simultaneous saccharification and fermentation (SSF), simultaneous saccharification and co-fermentation (SSCF), and consolidated bioprocessing (CBP) (Lu et al., 2021; Okolie et al., 2022). This section describes the enzymes required for the production of SA and the hydrolysis of waste biomass after relevant pretreatment.
3.1 Waste biomass pretreatment and utilization
Pretreatment of biomass includes physical, chemical, and biological methods. Alkali treatment is simple and effective and is a commonly used chemical treatment. For carbohydrate-rich sugarcane bagasse, alkali treatment with NaOH and enzymatic hydrolysis resulted in fermentable sugar yields of up to 88.7%. SA production using sugarcane bagasse hydrolysate was conducted, in which SA titer and yield were 33.2 g/L and 0.580 g/g, respectively (Ong et al., 2019). Additionally, sugarcane bagasse pre-treatment with alkaline hydrogen peroxide (AHP) at mild conditions could obtain a yield of 74.3% fermentable sugar. Followed by fed-batch fermentation to produce SA, all glucose and xylose could be utilized, and the obtained concentration and yield of SA reached 41.4 g/L and 0.290 g/g sugarcane bagasse raw material (Zhang et al., 2022).
Ionic liquids (ILs) are a promising biomass pretreatment solvent with the advantages of being non-toxic, stable, and recyclable. Under relatively mild conditions, ILs can effectively dissolve cellulose, break the dense structure formed by lignin, and expose cellulose from the coating of lignin and hemicellulose. Pinewood was pretreated with 1-allyl-3-methylimidazolium chloride [AmimCl], and the enzymatic hydrolysis rate of pinewood extract could reach 72.2%. A. succinogenes 130Z can produce 20.7 g/L SA from pinewood extract with a CFR of 0.336 g/L/h (Wang C. et al., 2014). Additionally, mulberry stem (MS) was pretreated with cholinium-glycinate ([Ch][Gly]) and cholinium-alanate ([Ch][Ala]). Compared to untreated samples, glucose yield increased from 14.0% to 74.0%. A. succinogenes ATCC55618 was applied for SA fermentation with a productivity of 1.18 g/L/h and a CFR of 0.440 g/L/h (Pakdeedachakiat et al., 2019). In addition to lignocellulose, microalgal biomass could also be pretreated by ILs (Sorokina et al., 2020). With 1-butyl-3-methylimidazolium hydrogen sulfate ([BMIM][HSO4]), microalgal biomass was treated directly by biomass transesterification. The sugar yield of microalgae extract after acid hydrolysis was 81.1%. Being fermented with A. succinogenes 130Z, the purified hydrolysate obtained a SA productivity of 0.190 g/L/h and a CFR of 0.0708 g/L/h. In fact, microalgae themselves also have strong carbon sequestration capacity (Chiang et al., 2021), which also increases the effect of carbon capture, utilization and storage (CCUS) in the SA production process (Yang et al., 2022; Xu et al., 2023).
Corn fiber hydrolysate could be obtained by using optimized liquid hot water (LHW). Being fermented by A. succinogenes 130Z, corn fiber hydrolysate could produce 27.8 g/L SA with a CFR of 0.288 g/L/h (Vallecilla-Yepez et al., 2021). The sugarcane bagasse residue obtained by LHW and alkali-pretreated has better digestibility and a higher SA conversion rate. And Chen et al. developed an in-situ semi-simultaneous saccharification and co-fermentation process to produce SA from sugarcane bagasse, which could obtain 41.0 g/L SA with a CFR of 0.0921 g/L/h (Chen et al., 2021). Hassan et al. pretreated the oil palm empty fruit bunch (OPEFB) with inorganic salts to improve its delignification and saccharification yields and obtained a large amount of total reducing sugar (Hassan et al., 2020). Based on the inorganic salt pretreatment of OPEFB, SA is produced through SSF, with a production of 65.2 g/L and a yield of 0.650 g/g OPEFB (Khairil Anwar et al., 2021). Hydrolysis products obtained by dilute acid and enzyme pretreatment of durian shells were recently reported to be used as a carbon source for succinic acid production, with product yields of 49.0% and 63.0%, respectively. The fermentation of durian shell hydrolysate consumes 0.280–0.310 kg of carbon dioxide per kg of succinic acid produced, which is a potential technology for the production of carbon sequestration and high value-added chemicals (Woo et al., 2023). Therefore, a gentle approach to biomass pretreatment is key to the green manufacture of bio-based chemicals.
The biosynthesis of SA from biomass using mild pretreatment, enzymatic saccharification, and microbial fermentation provides an efficient and sustainable replacement for petroleum-based methods (Zhang et al., 2022). Additionally, oil palm trunk (OPT) juice could also be used as the sole carbon source to produce SA. It was demonstrated that when using OPT sap as the only substrate, the SA yield and productivity could reach 0.540 g/g and 0.350 g/L/h (Bukhari et al., 2019). This work shows that OPT sap contains enough nutrients for A. succinogenes 130Z to synthesize SA, and could reduce costs without the supplement of expensive nutrients. There are many cheap raw materials available to produce SA, such as mixed food waste and vegetable waste (Li C. et al., 2018; Li et al., 2019), and how to reduce costs while increasing the yield of products is still a problem to be considered in industrial production.
3.2 Hydrolytic enzymes for waste biomass
Waste biomass is a plentiful and cheap renewable carbon source, including agricultural residues such as sugarcane bagasse and wheat straw, as well as food waste generated by human activities (Pati et al., 2023). The main components of most of these biomasses are cellulose, hemicellulose, and lignin (Zerva et al., 2021). Naturally, a wide range of microorganisms carry a rich and sophisticated enzymatic arsenal, including cellulase, hemicellulase, ligninase, and auxiliary enzymes, which could completely degrade waste biomass into fermentable sugars (Guo H. et al., 2022; Guo X. et al., 2022). These hydrolytic enzymes have been extensively studied (Guo et al., 2023), such as cellulase in Bacillus tequilensis (ON754229) (Malik and Javed, 2024), hydrolase in cellulolytic nitrogen-fixing bacteria (Harindintwali et al., 2022), and β-glucosidase and xylanase in Trichoderma asperellum LYS1 (Mou et al., 2023). Newly identified hydrolases with excellent enzymatic properties could be obtained from nature, but the process is time-consuming and labor-intensive. In recent years, with the continued refinement of protein structure analyses and high-throughput screening, directed evolution, semi-rational and rational design have been used to rapidly improve the thermostability, catalytic activity, and substrate specificity of hydrolases (Dadwal et al., 2020).
Although numerous microorganisms applied to biomass hydrolysis have been investigated, microorganisms which are cellulolytic and SA-producing at the same time have rarely been reported. Yang et al. constructed an engineered yeast strain carrying the gene encoding endo-polygalacturonase from Aspergillus niger 1805 to produce oligogalacturonides directly from citrus peel wastes (Yang et al., 2020). Fathima et al. employed Clostridium phytofermentans DSM1183 to directly bioconvert waste water algal biomass into ethanol (Fathima et al., 2016). According to studies that bioconverting waste biomass into high-value compounds by hydrolase-producing strains, it may be possible to achieve a one-pot biosynthesis from waste biomass to SA by integrating the functions of hydrolase-producing strains and SA-producing strains. For instance, genes encoding hydrolytic enzymes could be introduced into SA-producing engineered strains, or cellulolytic microorganisms could be given the ability to produce SA, which could enable strains to produce SA directly from biomass. Moreover, hydrolase-producing microorganisms and SA-producing microorganisms could be co-cultured and fermented to realize one-pot biosynthesis from waste biomass to SA.
4 Process improvement for improved SA yield and carbon sequestration
4.1 Bioelectrochemical strategies
NADH is often required to provide reducing power during anaerobic fermentation of SA, for which an external potential supply can provide more energy required for the enzyme-catalyzed reaction, thus improving the SA biosynthesis process. Different levels of oxidation-reduction potential (ORP) were examined in SA production from glucose by E. coli to determine the optimal external potential supply (Liu et al., 2014). Since the ORP level affects enzyme activity and metabolic flux, cell growth and SA yield change with the ORP level ranging from −200 to −400 mV during anaerobic fermentation. At the redox potential level of −400 mV, SA production reached 28.6 g/L, with a yield increased by 39% compared to that of −200 mV. Amulya et al. also applied the bioelectrochemical system (BES) to SA production from CO2 by facultative anaerobic bacteria Citrobacter amalonaticus (Amulya and Venkata Mohan, 2021). When the constant applied potential was −800 mV, SA yield reached 14.4 g/L. In this context, the replenishment of external potential could effectively maintain the microbial redox reactions in the fermentation system and is especially conducive to the regeneration of NAD(H) (Barin et al., 2023).
Additionally, a novel electrochemical membrane bioreactor was designed to produce SA, which enables the in-situ separation of SA in the anode chamber through an anion exchange membrane (Stylianou et al., 2023). With the organic fraction of municipal solid waste (OFMSW) as feedstock, this electrochemical and bioreactor coupling strategy could produce SA 66.7 g/L with a yield of 0.510 g/g. In this electrochemical process, compared to the traditional process, the use of renewable power could replace the use of alkali and acid, increase SA productivity, and reduce SA purification operations.
4.2 Bioreactions coupling system
The primary benefit of SA production in anaerobic conditions is the ability of CO2 fixation during the conversion of PEP or pyruvate to oxaloacetate. When compared to fossil-fuel-based SA, each ton of bio-based SA is predicted to reduce CO2 emissions by 4.50–5.00 tons (Gunnarsson et al., 2014). Therefore, the generation of off-gases can be coupled with a fermentation-synthesis process to capture and utilize CO2 more efficiently.
Linked-fermentation strategies can help to produce multiple products at once and the substrates can be more fully utilized (Zhang et al., 2017; Gao et al., 2022; Yin and Wang, 2022). Saccharomyces cerevisiae and Actinobacillus succinogenes were co-cultured in the hydrolysate of sugarcane bagasse. S. cerevisiae produced EtOH and CO2 from glucose in the hydrolysate, and A. succinogenes used reducing sugars in the hydrolysate and CO2 to synthesize SA. After fermentation in a 1.5-L fermenter for 60 h, 22.0 g/L of EtOH and 22.1 g/L of SA were obtained, with a CFR of 0.137 g/L/h (Xu et al., 2021). Hence, combining the production of ethanol (EtOH) and SA could not only reduce CO2 emissions, but also supply a significant amount of CO2 for SA production.
There are other similar substrate co-fermentation strategies, such as co-production of SA and cadaverine (Gao et al., 2022). The SA pathway was introduced into cadaverine-producing strains, and a thermal switch system was established and optimized to realize a two-stage co-production. Cell proliferation and lysine synthesis occurred during the primary stage, while SA and cadaverine were produced during the following stage. In a 5 L bioreactor, the production of SA reached 28.4 g/L, while that of cadaverine was 55.6 g/L. In the whole process, decarboxylation of L-lysine to form cadaverine releases CO2, while carboxylation of PEP to form oxaloacetate fixes CO2 (Xi et al., 2022). Based on this fact we conclude that CO2 emissions could be greatly decreased during co-production, which would benefit the atom economy.
Additionally, a two-chamber bioreactor coupling device was constructed to produce EtOH and SA respectively and the CO2 generated from EtOH fermentation was directly supplied to SA fermentation. For instance, the CO2 generated in EtOH fermentation from corn was absorbed on a packed column that contained a solution of NaOH, KOH, or NH4OH. The obtained carbonate solution was used both for pH control and for the supply of CO2 for SA production (Nghiem and Senske, 2015). By optimizing the volume ratio of the working medium in the two-chamber bioreactor, the maximum yields of EtOH and SA from glucose were 0.510 g/g and 0.700 g/g, respectively. As a result, after fermentation in a 3 L fermenter for 70 h, 31.6 g/L of SA was obtained with a CFR of 0.168 g/L/h (Qian and Zheng, 2023).
Amulya et al. screened a Citrobacter amalonaticus to produce SA and studied its fixation of CO2 (Amulya and Mohan, 2019). They used H2 gas as an electron donor, and simultaneously externally supplied H2 and CO2 gases for fermentation during SA production. With sucrose as substrate, Citrobacter amalonificus could produce SA 12.1 g/L with a CO2 partial pressure of 1 bar. Furthermore, increasing CO2 partial pressure from 0.6 to 2 bar resulted in an increase from 0.210 to 0.460 g/L/h of SA productivity, and CFR increased from 0.0783 to 0.172 g/L/h (Amulya et al., 2020). Moreover, the production of the by-products under high-pressure CO2 conditions was significantly reduced, especially lactic acid and formic acid (Mota et al., 2019). Carbonate replaced with CO2 at different ratios also improves the yield and selectivity of SA, as gaseous CO2 penetrates cell membranes and is better utilized by cells (Yang et al., 2024). Therefore, it is believed that the most important factors affecting SA generation are the solubility of CO2 and extra electron donors (Tan et al., 2014). The positive impact of CO2 on the production of SA creates opportunities for sustainable collaboration between SA industries and other biofuel industries that generate CO2 (Annie Modestra et al., 2020). Although the additional supply of CO2 can increase SA production, commercial CO2 is relatively expensive and not suitable for industrial production. From the perspective of industrial application, the linked-fermentation strategy is more environmentally friendly and has more application potential than traditional SA fermentation (Su et al., 2021). However, the CO2 provided by the linked-fermentation strategy is limited, and the supply may be unstable, so the industrial production of SA with CO2 as the sole fermentation substrate remains a great challenge.
5 Techno-economic analysis and carbon sequestration benefits
The cost of feedstock significantly influences the economics of the manufacturing of bio-based SA (Stylianou et al., 2020). The use of CO2 and waste biomass as feedstocks to produce SA has high economic benefits and the production cost is also affected by the options of alkaline neutralizers. As mentioned above, although MgCO3 has better performance in fermentation, its high cost is not suitable for SA biorefineries. Replacing MgCO3 with a mixture of low-cost Mg(OH)2 and NH3·H2O could also obtain high SA yields (Wang J. et al., 2014).
A multiproduct model using pulp logs as biomass feedstock was studied, producing acetate, dimethyl ether and SA (Ghayur et al., 2019). In terms of the capacity to mitigate climate change, the advantage is that acetate is biosynthesized from biomass rather than being derived from fossil fuel, and dimethyl ether could be used for power generation. In terms of economy, the additional revenue from the sale of acetate and dimethyl ether compensates for the production costs. Therefore, a multiproduct SA biorefinery is technically and economically feasible, with the potential to be carbon-negative and to stimulate regional economics. The novel SA biorefinery could make full use of all the reducing sugars from the waste biomass to produce a wide range of chemicals, including SA, with CO2 as one of the feedstocks to produce the platform chemicals (Kumar et al., 2022). Therefore, the production of chemicals in biorefineries is also a process of CO2 integration, which has technical, financial and environmental advantages (Filippi et al., 2022).
Another simulation process is to produce SA from glycerol and add dimethyl sulfoxide as the electron acceptor (Thanahiranya et al., 2023). The model evaluates the performance of the selected situations according to techno-economic, efficiency, and greenhouse gas emissions. The results demonstrate that adding dimethyl sulfoxide to fermentation is the key to produce SA, which solves the problem of excessive glycerol (Carvalho et al., 2014; Kaur et al., 2020), and the best profit expected to be generated over 5 years is $190 million, with an internal rate of return of 33.3%. The next compelling step is to set up a pilot manufacturing plant to validate and optimize the models to reduce the risk of new synergies required for such approaches.
Several types of research have been done to investigate the environmental benefits of SA synthesis from waste biomass such as bread waste, the organic fraction of municipal solid waste (OFMSW), apple pomace, etc. The environmental impact of SA produced from waste bread is substantially lower than that of SA derived from fossil fuels. Furthermore, although the emissions of greenhouse gas were relatively higher compared to corn and sorghum grains, using waste bread eliminates the requirement for food crops and arable land (Gadkari et al., 2021). Additionally, OFMSW could also be used as a feedstock for SA fermentation as it contains 30.0%–60.0% carbohydrates (Stylianou et al., 2021). Considering the CO2 emissions from OFMSW landfill disposal, SA biorefining from OFMSW contributes 35.0% fewer CO2 emissions than traditional processes based on fossil-derived. Moreover, the established biorefinery generated sugar-rich hydrolysate with 100% recovery of oils/fats and 68.0% recovery of protein, which was then used to produce SA (Ladakis et al., 2022). This cradle-to-gate life cycle assessment (LCA) approach is also typically used for techno-economic analysis of chemical production to determine its environmental impact (Ögmundarson et al., 2020). As is shown in Table 3, the economic and carbon sequestration benefits of various SA biorefineries are listed.
The techno-economic analysis, life cycle assessment and carbon sequestration benefit evaluation of SA refineries are conducive to advancing the industrialization of SA biosynthesis. However, the energy consumed by SA biorefineries and the subsequent separation and purification processes are still the main obstacles to the large-scale production of bio-based SA. Although the fixation of CO2 for SA production can achieve high carbon sequestration benefits, a stable industrial supply of CO2 is still an unsolved problem. Owing to the above-mentioned disadvantages of bio-based SA, two suggestions could be proposed. One is to construct a more robust microbial host for SA fermentation with high yields and productivity at low CO2 supply. Another is to develop a fermentation process strategy with low-cost raw materials as the primary substrate and CO2 as a secondary substrate, which can help to realize a process with high economic competitiveness (Li et al., 2021). Bio-based products biorefineries would be more desirable and might eventually take the place of petroleum-based production if a method with higher energy efficiency was developed.
6 A new technology to assist SA biosynthesis—droplet microfluidics-based high-throughput screening
Currently, there are still some problems to be solved for SA biosynthesis, such as the fact that most succinic acid-producing strains prefer neutral pH conditions, but succinic acid formation acidifies the medium, leading to low robustness of the engineered bacteria and high cost of recovery of downstream products; and low activity of enzymes for hydrolyzing biomass. All these problems also limit the CO2 fixation during SA synthesis. To address the above problems, screening engineered strains with high SA production and acid tolerance by high-throughput screening techniques is a promising approach, taking efficiency and cost into account.
Conventional high-throughput screening platforms use 96 microplates, based on the principles of “arrangement” and “combination,” to systematically perform basic fluidic operations through automation, followed by detection and data processing to complete the analysis process. One or more high-yielding strains can be screened from a library of mutant strains (Hertzberg and Pope, 2000). Although the screening flux is indeed greatly improved compared to manual screening of strains, there are also major constraints, such as expensive instruments, inability to avoid cross-contamination, and a large difference in the efficiency and cost of data collection (Shi et al., 2023). To address the above problems, it is revolutionary to use droplet microfluidics instead of well plates to realize high-throughput screening. Compared to high-throughput screening with well plates, droplet microfluidics not only improves the flux by at least 4 orders of magnitude, but also drastically reduces the cost and time of each experiment (Ding et al., 2020).
Therefore, if the high-throughput screening platform of droplet microfluidics can be used for the screening of key enzymes or strains in SA biosynthesis, it will undoubtedly accelerate the development of SA biosynthesis. Based on this, the combination of ultra-high-throughput droplet microfluidic screening platforms and spectroscopic techniques also provides for high-throughput screening of strains, such as Raman-activated cell sorting (RACS) (Nitta et al., 2020) and fluorescence-activated cell sorting (FACS) (Li C. et al., 2023). Combining a droplet-captured microfluidic cell-sorting device with a spectral analysis device allows individual cells to be analyzed by Raman microspectroscopy or fluorescence detection, and cells can be sorted as needed. By using in-depth computational analysis strategies and artificial intelligence, new enzymes or pathways for SA biosynthesis can be discovered and constructed, with AI-designed enzyme mutants often exhibiting higher activity and stability (Li R. et al., 2018; Gargiulo and Soumillion, 2021). The development of machine learning algorithms, such as AlphaFold2, has also facilitated the analysis of enzyme structures and properties, allowing the prediction of beneficial mutation sites based on the structure of key enzymes (Fang et al., 2022; Ge et al., 2023). In addition, the construction of SA-responsive biosensing cells may be the key to realizing high-throughput screening of SA-producing strains, and thus the development of SA-responsive bioelements is imminent. Based on this, we are recently designing a droplet microfluidic screening platform based on surface-enhanced Raman spectroscopy in order to realize high-throughput screening of strains with high SA production. This system, if successfully realized, will achieve detection and sorting of about 2 million single drops at 160 drops/s, which will be a great innovation. In addition, high-yielding SA-producing strains exhibit greater CO2 fixation capacity, leading to higher carbon sequestration benefits.
Taken together, these strategies help to select the best enzyme or strain mutant in the shortest possible time, creating a great opportunity for the modification of engineered strains to produce SA for utilization of CO2 and waste biomass as feedstock, and achieving higher carbon sequestration benefits.
7 Conclusion
The biosynthesis process of bio-based SA has been attracting attention. The rise of SA biorefineries from CO2 and waste biomass as feedstocks shows great potential for CO2 emission reduction and environmental protection. By exploring novel technologies of synthetic biology and integrating multiple disciplines, such as biosensing and artificial intelligence, the biosynthesis strategy could establish a green and sustainable future for SA production, achieve great techno-economic feasibility and CO2 sequestration efficiency, and result in harmony between man and the natural environment.
Author contributions
FL: Writing – original draft, Writing – review and editing. WL: Writing – original draft, Writing – review and editing. DW: Funding acquisition, Writing – review and editing. GH: Writing –review and editing. ZQ: Writing – review and editing. XX: Writing – review and editing. LH: Writing – review and editing. XL: Writing – review and editing. RL: Writing – review and editing.
Funding
The author(s) declare that financial support was received for the research, authorship, and/or publication of this article. This work was supported by the National Key Research and Development Program of China (2021YFC2103300 and 2022YFC2105700), the National Natural Science Foundation of China (22378032), Fundamental Research Funds for the Central Universities (2022CDJHLW006 and 2023CDJXY-047), Scientific Research Foundation of State Key Lab. of Coal Mine Disaster Dynamics and Control (2011DA105287-FW202103), Chongqing Outstanding Youth Fund (cstc2021jcyj-jqX0013), Youth project of science and technology research program of Chongqing Education Commission of China (KJQN201900112) and Human Resources and Social Security Bureau of Chongqing (cx2023036).
Conflict of interest
The authors declare that the research was conducted in the absence of any commercial or financial relationships that could be construed as a potential conflict of interest.
Publisher’s note
All claims expressed in this article are solely those of the authors and do not necessarily represent those of their affiliated organizations, or those of the publisher, the editors and the reviewers. Any product that may be evaluated in this article, or claim that may be made by its manufacturer, is not guaranteed or endorsed by the publisher.
References
Ahn, J. H., Jang, Y. S., and Lee, S. Y. (2016). Production of succinic acid by metabolically engineered microorganisms. Curr. Opin. Biotechnol. 42, 54–66. doi:10.1016/j.copbio.2016.02.034
Ahn, J. H., Seo, H., Park, W., Seok, J., Lee, J. A., Kim, W. J., et al. (2020). Enhanced succinic acid production by Mannheimia employing optimal malate dehydrogenase. Nat. Commun. 11, 1970. doi:10.1038/s41467-020-15839-z
Amulya, K., Kopperi, H., and Venkata Mohan, S. (2020). Tunable production of succinic acid at elevated pressures of CO2 in a high pressure gas fermentation reactor. Bioresour. Technol. 309, 123327. doi:10.1016/j.biortech.2020.123327
Amulya, K., and Mohan, S. V. (2019). Fixation of CO2, electron donor and redox microenvironment regulate succinic acid production in Citrobacter amalonaticus. Sci. Total. Environ. 695, 133838. doi:10.1016/j.scitotenv.2019.133838
Amulya, K., and Venkata Mohan, S. (2021). Augmenting succinic acid production by bioelectrochemical synthesis: influence of applied potential and CO2 availability. Chem. Eng. J. 411, 128377. doi:10.1016/j.cej.2020.128377
Amulya, K., and Venkata Mohan, S. (2022). Green hydrogen based succinic acid and biopolymer production in a biorefinery: adding value to CO2 from acidogenic fermentation. Chem. Eng. J. 429, 132163. doi:10.1016/j.cej.2021.132163
Annie Modestra, J., Katakojwala, R., and Venkata Mohan, S. (2020). CO2 fermentation to short chain fatty acids using selectively enriched chemolithoautotrophic acetogenic bacteria. Chem. Eng. J. 394, 124759. doi:10.1016/j.cej.2020.124759
Barin, R., Biria, D., and Ali Asadollahi, M. (2023). Nicotinamide adenine dinucleotide hydrogen regeneration in a microbial electrosynthesis system by Enterobacter aerogenes. Bioelectrochemistry 149, 108309. doi:10.1016/j.bioelechem.2022.108309
Belbute, J. M., and Pereira, A. M. (2020). Reference forecasts for CO2 emissions from fossil-fuel combustion and cement production in Portugal. Energy Policy 144, 111642. doi:10.1016/j.enpol.2020.111642
Bukhari, N. A., Loh, S. K., Nasrin, A. B., Luthfi, A. a.I., Harun, S., Abdul, P. M., et al. (2019). Compatibility of utilising nitrogen-rich oil palm trunk sap for succinic acid fermentation by Actinobacillus succinogenes 130Z. Bioresour. Technol. 293, 122085. doi:10.1016/j.biortech.2019.122085
Carvalho, M., Matos, M., Roca, C., and Reis, M. a.M. (2014). Succinic acid production from glycerol by Actinobacillus succinogenes using dimethylsulfoxide as electron acceptor. New Biotechnol. 31, 133–139. doi:10.1016/j.nbt.2013.06.006
Chatterjee, R., Millard, C. S., Champion, K., Clark, D. P., and Donnelly, M. I. (2001). Mutation of the ptsG gene results in increased production of succinate in fermentation of glucose by Escherichia coli. Appl. Environ. Microbiol. 67, 148–154. doi:10.1128/AEM.67.1.148-154.2001
Chen, J., Yang, S., Alam, M. A., Wang, Z., Zhang, J., Huang, S., et al. (2021). Novel biorefining method for succinic acid processed from sugarcane bagasse. Bioresour. Technol. 324, 124615. doi:10.1016/j.biortech.2020.124615
Chiang, Y. Y., Nagarajan, D., Lo, Y. C., Chen, C. Y., Ng, I. S., Chang, C. H., et al. (2021). Succinic acid fermentation with immobilized Actinobacillus succinogenes using hydrolysate of carbohydrate-rich microalgal biomass. Bioresour. Technol. 342, 126014. doi:10.1016/j.biortech.2021.126014
Choi, K. R., Ahn, Y.-J., and Lee, S. Y. (2022). Bacterial conversion of CO2 to organic compounds. J. CO2 Util. 58, 101929. doi:10.1016/j.jcou.2022.101929
Choi, S., Song, H., Lim, S. W., Kim, T. Y., Ahn, J. H., Lee, J. W., et al. (2016). Highly selective production of succinic acid by metabolically engineered Mannheimia succiniciproducens and its efficient purification. Biotechnol. Bioeng. 113, 2168–2177. doi:10.1002/bit.25988
Chung, S.-C., Park, J.-S., Yun, J., and Park, J. H. (2017). Improvement of succinate production by release of end-product inhibition in Corynebacterium glutamicum. Metab. Eng. 40, 157–164. doi:10.1016/j.ymben.2017.02.004
Cozzone, A. J., and El-Mansi, M. (2005). Control of isocitrate dehydrogenase catalytic activity by protein phosphorylation in Escherichia coli. J. Mol. Microbiol. Biotechnol. 9, 132–146. doi:10.1159/000089642
Craveiro, R., Dusschooten, F., Nabais, A. R., Boboescu, I., Lo, C., Neves, L. A., et al. (2022). Deep eutectic systems for carbonic anhydrase extraction from microalgae biomass to improve carbon dioxide solubilization. J. CO2 Util. 65, 102225. doi:10.1016/j.jcou.2022.102225
Cui, Z., Gao, C., Li, J., Hou, J., Lin, C. S. K., and Qi, Q. (2017). Engineering of unconventional yeast Yarrowia lipolytica for efficient succinic acid production from glycerol at low pH. Metab. Eng. 42, 126–133. doi:10.1016/j.ymben.2017.06.007
Cui, Z., Zhong, Y., Sun, Z., Jiang, Z., Deng, J., Wang, Q., et al. (2023). Reconfiguration of the reductive TCA cycle enables high-level succinic acid production by Yarrowia lipolytica. Nat. Commun. 14, 8480. doi:10.1038/s41467-023-44245-4
Dadwal, A., Sharma, S., and Satyanarayana, T. (2020). Progress in ameliorating beneficial characteristics of microbial cellulases by genetic engineering approaches for cellulose saccharification. Front. Microbiol. 11, 1387. doi:10.3389/fmicb.2020.01387
Dickson, R., Mancini, E., Garg, N., Woodley, J. M., Gernaey, K. V., Pinelo, M., et al. (2021). Sustainable bio-succinic acid production: superstructure optimization, techno-economic, and lifecycle assessment. Energy Environ. Sci. 14, 3542–3558. doi:10.1039/d0ee03545a
Ding, Y., Howes, P. D., and Demello, A. J. (2020). Recent advances in droplet microfluidics. Anal. Chem. 92, 132–149. doi:10.1021/acs.analchem.9b05047
Fang, K., Fang, J., Han, L., Yin, J., Liu, T., and Wang, X. (2022). Systematic evaluation of chiral fungicide penflufen for the bioactivity improvement and input reduction using alphafold2 models and transcriptome sequencing. J. Hazard. Mater. 440, 129729. doi:10.1016/j.jhazmat.2022.129729
Fathima, A. A., Sanitha, M., Kumar, T., Iyappan, S., and Ramya, M. (2016). Direct utilization of waste water algal biomass for ethanol production by cellulolytic Clostridium phytofermentans DSM1183. Bioresour. Technol. 202, 253–256. doi:10.1016/j.biortech.2015.11.075
Filippi, K., Papapostolou, H., Alexandri, M., Vlysidis, A., Myrtsi, E. D., Ladakis, D., et al. (2022). Integrated biorefinery development using winery waste streams for the production of bacterial cellulose, succinic acid and value-added fractions. Bioresour. Technol. 343, 125989. doi:10.1016/j.biortech.2021.125989
Forfora, N., Azuaje, I., Vivas, K. A., Vera, R. E., Brito, A., Venditti, R., et al. (2024). Evaluating biomass sustainability: why below-ground carbon sequestration matters. J. Clean. Prod. 439, 140677. doi:10.1016/j.jclepro.2024.140677
Gadkari, S., Kumar, D., Qin, Z. H., Ki Lin, C. S., and Kumar, V. (2021). Life cycle analysis of fermentative production of succinic acid from bread waste. Waste Manag. 126, 861–871. doi:10.1016/j.wasman.2021.04.013
Gao, S., Lu, J., Wang, T., Xu, S., Wang, X., Chen, K., et al. (2022). A novel co-production of cadaverine and succinic acid based on a thermal switch system in recombinant Escherichia coli. Microb. Cell Fact. 21, 248. doi:10.1186/s12934-022-01965-4
Gargiulo, S., and Soumillion, P. (2021). Directed evolution for enzyme development in biocatalysis. Curr. Opin. Chem. Biol. 61, 107–113. doi:10.1016/j.cbpa.2020.11.006
Ge, Y., Liu, P., Chen, Q., Qu, M., Xu, L., Liang, H., et al. (2023). Machine learning-guided the fabrication of nanozyme based on highly-stable violet phosphorene decorated with phosphorus-doped hierarchically porous carbon microsphere for portable intelligent sensing of mycophenolic acid in silage. Biosens. Bioelectron. 237, 115454. doi:10.1016/j.bios.2023.115454
Geyer, R., Jambeck, J. R., and Law, K. L. (2017). Production use and fate of all plastics ever made. Sci. Adv. 3, e1700782. doi:10.1126/sciadv.1700782
Ghayur, A., Verheyen, T. V., and Meuleman, E. (2019). Techno-economic analysis of a succinic acid biorefinery coproducing acetic acid and dimethyl ether. J. Clean. Prod. 230, 1165–1175. doi:10.1016/j.jclepro.2019.05.180
Gunnarsson, I. B., Alvarado-Morales, M., and Angelidaki, I. (2014). Utilization of CO2 fixating bacterium Actinobacillus succinogenes 130Z for simultaneous biogas upgrading and biosuccinic acid production. Environ. Sci. Technol. 48, 12464–12468. doi:10.1021/es504000h
Guo, H., He, T., and Lee, D.-J. (2022a). Contemporary proteomic research on lignocellulosic enzymes and enzymolysis: a review. Bioresour. Technol. 344, 126263. doi:10.1016/j.biortech.2021.126263
Guo, H., Zhao, Y., Chang, J. S., and Lee, D. J. (2023). Enzymes and enzymatic mechanisms in enzymatic degradation of lignocellulosic biomass: a mini-review. Bioresour. Technol. 367, 128252. doi:10.1016/j.biortech.2022.128252
Guo, X., An, Y., Liu, F., Lu, F., and Wang, B. (2022b). Lytic polysaccharide monooxygenase - a new driving force for lignocellulosic biomass degradation. Bioresour. Technol. 362, 127803. doi:10.1016/j.biortech.2022.127803
Harindintwali, J. D., Wang, F., Yang, W., Zhou, J., Muhoza, B., Mugabowindekwe, M., et al. (2022). Harnessing the power of cellulolytic nitrogen-fixing bacteria for biovalorization of lignocellulosic biomass. Ind. Crops Prod. 186, 115235. doi:10.1016/j.indcrop.2022.115235
Hassan, N., Khairil Anwar, N. a.K., and Idris, A. (2020). Strategy to enhance the sugar production using recyclable inorganic salt for pre-treatment of oil palm empty fruit bunch (OPEFB). BioResources 15, 4912–4931. doi:10.15376/biores.15.3.4912-4931
Hepburn, C., Adlen, E., Beddington, J., Carter, E. A., Fuss, S., Mac Dowell, N., et al. (2019). The technological and economic prospects for CO2 utilization and removal. Nature 575, 87–97. doi:10.1038/s41586-019-1681-6
Hertzberg, R. P., and Pope, A. J. (2000). High-throughput screening: new technology for the 21st century. Curr. Opin. Chem. Biol. 4, 445–451. doi:10.1016/S1367-5931(00)00110-1
Hou, Y., Gao, B., Cui, J., Tan, Z., Qiao, C., and Jia, S. (2019). Combination of multi-enzyme expression fine-tuning and co-substrates addition improves phenyllactic acid production with an Escherichia coli whole-cell biocatalyst. Bioresour. Technol. 287, 121423. doi:10.1016/j.biortech.2019.121423
Huang, M., Cheng, J., Chen, P., Zheng, G., Wang, D., and Hu, Y. (2019). Efficient production of succinic acid in engineered Escherichia coli strains controlled by anaerobically-induced nirB promoter using sweet potato waste hydrolysate. J. Environ. Manage. 237, 147–154. doi:10.1016/j.jenvman.2019.02.041
Ioannidou, S. M., Filippi, K., Kookos, I. K., Koutinas, A., and Ladakis, D. (2022). Techno-economic evaluation and life cycle assessment of a biorefinery using winery waste streams for the production of succinic acid and value-added co-products. Bioresour. Technol. 348, 126295. doi:10.1016/j.biortech.2021.126295
Ishihama, A. (2010). Prokaryotic genome regulation: multifactor promoters, multitarget regulators and hierarchic networks. FEMS Microbiol. Rev. 34, 628–645. doi:10.1111/j.1574-6976.2010.00227.x
Jiang, M., Liu, S. W., Ma, J. F., Chen, K. Q., Yu, L., Yue, F. F., et al. (2010). Effect of growth phase feeding strategies on succinate production by metabolically engineered Escherichia coli. Appl. Environ. Microbiol. 76, 1298–1300. doi:10.1128/AEM.02190-09
Kang, K. H., Hong, U. G., Jun, J. O., Song, J. H., Bang, Y., Choi, J. H., et al. (2014). Hydrogenation of succinic acid to γ-butyrolactone and 1,4-butanediol over mesoporous rhenium–copper–carbon composite catalyst. J. Mol. Catal. A Chem. 395, 234–242. doi:10.1016/j.molcata.2014.08.032
Karakurt, I., and Aydin, G. (2023). Development of regression models to forecast the CO2 emissions from fossil fuels in the BRICS and MINT countries. Energy 263, 125650. doi:10.1016/j.energy.2022.125650
Kaur, J., Sarma, A. K., Jha, M. K., and Gera, P. (2020). Valorisation of crude glycerol to value-added products: perspectives of process technology, economics and environmental issues. Biotechnol. Rep. 27, e00487. doi:10.1016/j.btre.2020.e00487
Khairil Anwar, N. a.K., Hassan, N., Mohd Yusof, N., and Idris, A. (2021). High-titer bio-succinic acid production from sequential alkalic and metal salt pretreated empty fruit bunch via simultaneous saccharification and fermentation. Ind. Crops Prod. 166, 113478. doi:10.1016/j.indcrop.2021.113478
Kumar, A. N., Sarkar, O., Chandrasekhar, K., Raj, T., Narisetty, V., Mohan, S. V., et al. (2022). Upgrading the value of anaerobic fermentation via renewable chemicals production: a sustainable integration for circular bioeconomy. Sci. Total Environ. 806, 150312. doi:10.1016/j.scitotenv.2021.150312
Kwon, Y. D., Kwon, O. H., Lee, H. S., and Kim, P. (2007). The effect of NADP-dependent malic enzyme expression and anaerobic C4 metabolism in Escherichia coli compared with other anaplerotic enzymes. J. Appl. Microbiol. 103, 2340–2345. doi:10.1111/j.1365-2672.2007.03485.x
Ladakis, D., Stylianou, E., Ioannidou, S.-M., Koutinas, A., and Pateraki, C. (2022). Biorefinery development, techno-economic evaluation and environmental impact analysis for the conversion of the organic fraction of municipal solid waste into succinic acid and value-added fractions. Bioresour. Technol. 354, 127172. doi:10.1016/j.biortech.2022.127172
Lai, M. J., Tsai, J. C., and Lan, E. I. (2022). CRISPRi-enhanced direct photosynthetic conversion of carbon dioxide to succinic acid by metabolically engineered cyanobacteria. Bioresour. Technol. 366, 128131. doi:10.1016/j.biortech.2022.128131
Le, S. D., and Nishimura, S. (2021). Influence of metal ratio on alumina-supported CuPd catalysts for the production of tetrahydrofuran from succinic acid. Appl. Catal. A 616, 118063. doi:10.1016/j.apcata.2021.118063
Lee, J. W., Yi, J., Kim, T. Y., Choi, S., Ahn, J. H., Song, H., et al. (2016). Homo-succinic acid production by metabolically engineered Mannheimia succiniciproducens. Metab. Eng. 38, 409–417. doi:10.1016/j.ymben.2016.10.004
Li, C., Gao, X., Qi, H., Zhang, W., Li, L., Wei, C., et al. (2023a). Substantial improvement of an epimerase for the synthesis of D-allulose by biosensor-based high-throughput microdroplet screening. Angew. Chem. Int. Ed. 62, e202216721. doi:10.1002/anie.202216721
Li, C., Ong, K. L., Cui, Z., Sang, Z., Li, X., Patria, R. D., et al. (2021). Promising advancement in fermentative succinic acid production by yeast hosts. J. Hazard. Mater. 401, 123414. doi:10.1016/j.jhazmat.2020.123414
Li, C., Ong, K. L., Yang, X., and Lin, C. S. K. (2019). Bio-refinery of waste streams for green and efficient succinic acid production by engineered Yarrowia lipolytica without pH control. Chem. Eng. J. 371, 804–812. doi:10.1016/j.cej.2019.04.092
Li, C., Xiao, Y., Sang, Z., Yang, Z., Xu, T., Yang, X., et al. (2022). Inhibition kinetics of bio-based succinic acid production by the yeast Yarrowia lipolytica. Chem. Eng. J. 442, 136273. doi:10.1016/j.cej.2022.136273
Li, C., Yang, X., Gao, S., Chuh, A. H., and Lin, C. S. K. (2018a). Hydrolysis of fruit and vegetable waste for efficient succinic acid production with engineered Yarrowia lipolytica. J. Clean. Prod. 179, 151–159. doi:10.1016/j.jclepro.2018.01.081
Li, C., Yang, X., Gao, S., Wang, H., and Lin, C. S. K. (2017a). High efficiency succinic acid production from glycerol via in situ fibrous bed bioreactor with an engineered Yarrowia lipolytica. Bioresour. Technol. 225, 9–16. doi:10.1016/j.biortech.2016.11.016
Li, J., Li, Y., Cui, Z., Liang, Q., and Qi, Q. (2017b). Enhancement of succinate yield by manipulating NADH/NAD+ ratio and ATP generation. Appl. Microbiol. Biotechnol. 101, 3153–3161. doi:10.1007/s00253-017-8127-6
Li, K., Li, C., Zhao, X.-Q., Liu, C.-G., and Bai, F.-W. (2023b). Engineering Corynebacterium glutamicum for efficient production of succinic acid from corn stover pretreated by concentrated-alkali under steam-assistant conditions. Bioresour. Technol. 378, 128991. doi:10.1016/j.biortech.2023.128991
Li, R., Wijma, H. J., Song, L., Cui, Y., Otzen, M., Tian, Y. E., et al. (2018b). Computational redesign of enzymes for regio- and enantioselective hydroamination. Nat. Chem.l Bio. 14, 664–670. doi:10.1038/s41589-018-0053-0
Li, X., Zhang, W., Wu, M., Xin, F., Dong, W., Wu, H., et al. (2017c). Performance and mechanism analysis of succinate production under different transporters in Escherichia coli. Biotechnol. Bioprocess Eng. 22, 529–538. doi:10.1007/s12257-017-0086-3
Lin, H., Bennett Gn Fau - San, K.-Y., and San, K. Y. (2005). Fed-batch culture of a metabolically engineered Escherichia coli strain designed for high-level succinate production and yield under aerobic conditions. Biotechnol. Bioeng. 90, 775–779. doi:10.1002/bit.20458
Liu, R., Liang, L., Jiang, M., Ma, J., Chen, K., Jia, H., et al. (2014). Effects of redox potential control on succinic acid production by engineered Escherichia coli under anaerobic conditions. Process Biochem. 49, 740–744. doi:10.1016/j.procbio.2014.02.010
Liu, R., Liang, L., Wu, M., Chen, K., Jiang, M., Ma, J., et al. (2013). CO2 fixation for succinic acid production by engineered Escherichia coli co-expressing pyruvate carboxylase and nicotinic acid phosphoribosyltransferase. Biochem. Eng. J. 79, 77–83. doi:10.1016/j.bej.2013.07.004
Liu, X., Feng, X., Ding, Y., Gao, W., Xian, M., Wang, J., et al. (2020). Characterization and directed evolution of propionyl-CoA carboxylase and its application in succinate biosynthetic pathway with two CO2 fixation reactions. Metab. Eng. 62, 42–50. doi:10.1016/j.ymben.2020.08.012
Liu, X., Zhao, G., Sun, S., Fan, C., Feng, X., and Xiong, P. (2022). Biosynthetic pathway and metabolic engineering of succinic acid. Front. Bioeng. Biotechnol. 10, 843887. doi:10.3389/fbioe.2022.843887
Louasté, B., and Eloutassi, N. (2020). Succinic acid production from whey and lactose by Actinobacillus succinogenes 130Z in batch fermentation. Biotechnol. Rep. 27, e00481. doi:10.1016/j.btre.2020.e00481
Lu, J., Li, J., Gao, H., Zhou, D., Xu, H., Cong, Y., et al. (2021). Recent progress on bio-succinic acid production from lignocellulosic biomass. World J. Microbiol. Biotechnol. 37, 16. doi:10.1007/s11274-020-02979-z
Ma, Z., Cheah, W. Y., Ng, I. S., Chang, J. S., Zhao, M., and Show, P. L. (2022). Microalgae-based biotechnological sequestration of carbon dioxide for net zero emissions. Trends Biotechnol. 40, 1439–1453. doi:10.1016/j.tibtech.2022.09.002
Malik, W. A., and Javed, S. (2024). Enhancement of cellulase production by cellulolytic bacteria SB125 in submerged fermentation medium and biochemical characterization of the enzyme. Int. J. Biol. Macromol. 263, 130415. doi:10.1016/j.ijbiomac.2024.130415
Marletta, A. S., Massarotti, A., Orsomando, G., Magni, G., Rizzi, M., and Garavaglia, S. (2015). Crystal structure of human nicotinic acid phosphoribosyltransferase. FEBS Open Bio 5, 419–428. doi:10.1016/j.fob.2015.05.002
Martins, T., Barreto, A. C., Souza, F. M., and Souza, A. M. (2021). Fossil fuels consumption and carbon dioxide emissions in G7 countries: empirical evidence from ARDL bounds testing approach. Environ. Pollut. 291, 118093. doi:10.1016/j.envpol.2021.118093
Mota, M. J., Lopes, R. P., Simões, M. M. Q., Delgadillo, I., and Saraiva, J. A. (2019). Effect of high pressure on paracoccus denitrificans growth and polyhydroxyalkanoates production from glycerol. Appl. Biochem. Biotechnol. 188, 810–823. doi:10.1007/s12010-018-02949-0
Mou, L., Pan, R., Liu, Y., Jiang, W., Zhang, W., Jiang, Y., et al. (2023). Isolation of a newly Trichoderma asperellum LYS1 with abundant cellulase-hemicellulase enzyme cocktail for lignocellulosic biomass degradation. Enzyme Microb. Technol. 171, 110318. doi:10.1016/j.enzmictec.2023.110318
Narisetty, V., Okibe, M. C., Amulya, K., Jokodola, E. O., Coulon, F., Tyagi, V. K., et al. (2022). Technological advancements in valorization of second generation (2G) feedstocks for bio-based succinic acid production. Bioresour. Technol. 360, 127513. doi:10.1016/j.biortech.2022.127513
Nghiem, N. P., and Senske, G. E. (2015). Capture of carbon dioxide from ethanol fermentation by liquid absorption for use in biological production of succinic acid. Appl. Biochem. Biotechnol. 175, 2104–2113. doi:10.1007/s12010-014-1369-1
Nitta, N., Iino, T., Isozaki, A., Yamagishi, M., Kitahama, Y., Sakuma, S., et al. (2020). Raman image-activated cell sorting. Nat. Commun. 11, 3452. doi:10.1038/s41467-020-17285-3
Ögmundarson, Ó., Herrgård, M. J., Forster, J., Hauschild, M. Z., and Fantke, P. (2020). Addressing environmental sustainability of biochemicals. Nat. Sustain. 3, 167–174. doi:10.1038/s41893-019-0442-8
Okolie, J. A., Epelle, E. I., Tabat, M. E., Orivri, U., Amenaghawon, A. N., Okoye, P. U., et al. (2022). Waste biomass valorization for the production of biofuels and value-added products: a comprehensive review of thermochemical, biological and integrated processes. Process Saf. Environ. Prot. 159, 323–344. doi:10.1016/j.psep.2021.12.049
Olajuyin, A. M., Yang, M., Thygesen, A., Tian, J., Mu, T., and Xing, J. (2019). Effective production of succinic acid from coconut water (Cocos nucifera) by metabolically engineered Escherichia coli with overexpression of Bacillus subtilis pyruvate carboxylase. Biotechnol. Rep. (Amst) 24, e00378. doi:10.1016/j.btre.2019.e00378
Ong, K. L., Li, C., Li, X., Zhang, Y., Xu, J., and Lin, C. S. K. (2019). Co-fermentation of glucose and xylose from sugarcane bagasse into succinic acid by Yarrowia lipolytica. Biochem.Eng. J. 148, 108–115. doi:10.1016/j.bej.2019.05.004
Pakchamni, P., Afedzi, A. E. K., and Parakulsuksatid, P. (2022). Optimization of alkaline-assisted organosolv pretreatment of sugarcane trash for the production of succinic acid using response surface methodology. Biocatal. Agric. Biotechnol. 43, 102374. doi:10.1016/j.bcab.2022.102374
Pakdeedachakiat, W., Phruksaphithak, N., and Boontawan, A. (2019). Pretreatment of mulberry stem by cholinium-based amino acid ionic liquids for succinic acid fermentation and its application in poly(butylene) succinate production. Bioresour. Technol. 291, 121873. doi:10.1016/j.biortech.2019.121873
Pati, S., De, S., and Chowdhury, R. (2023). Exploring the hybrid route of bio-ethanol production via biomass co-gasification and syngas fermentation from wheat straw and sugarcane bagasse: model development and multi-objective optimization. J. Clean. Prod. 395, 136441. doi:10.1016/j.jclepro.2023.136441
Prabhu, A. A., Ledesma-Amaro, R., Lin, C. S. K., Coulon, F., Thakur, V. K., and Kumar, V. (2020). Bioproduction of succinic acid from xylose by engineered Yarrowia lipolytica without pH control. Biotechnol. Biofuels 13, 113. doi:10.1186/s13068-020-01747-3
Priyadharsini, P., Nirmala, N., Dawn, S. S., Baskaran, A., Sundarrajan, P., Gopinath, K. P., et al. (2022). Genetic improvement of microalgae for enhanced carbon dioxide sequestration and enriched biomass productivity: review on CO2 bio-fixation pathways modifications. Algal Res. 66, 102810. doi:10.1016/j.algal.2022.102810
Putri, D. N., Sahlan, M., Montastruc, L., Meyer, M., Negny, S., and Hermansyah, H. (2020). Progress of fermentation methods for bio-succinic acid production using agro-industrial waste by Actinobacillus succinogenes. Energy Rep. 6, 234–239. doi:10.1016/j.egyr.2019.08.050
Qian, J., and Zheng, P. (2023). Fixation of CO2 from ethanol fermentation for succinic acid production in a dual-chamber bioreactor system. Biochem.Eng. J. 191, 108809. doi:10.1016/j.bej.2023.108809
Sanchez, A. M., Bennett, G. N., and San, K. Y. (2005). Novel pathway engineering design of the anaerobic central metabolic pathway in Escherichia coli to increase succinate yield and productivity. Metab. Eng. 7, 229–239. doi:10.1016/j.ymben.2005.03.001
Sawisit, A., Jantama, K., Zheng, H., Yomano, L. P., York, S. W., Shanmugam, K. T., et al. (2015). Mutation in galP improved fermentation of mixed sugars to succinate using engineered Escherichia coli AS1600a and AM1 mineral salts medium. Bioresour. Technol. 193, 433–441. doi:10.1016/j.biortech.2015.06.108
Shaji, A., Shastri, Y., Kumar, V., Ranade, V. V., and Hindle, N. (2021). Economic and environmental assessment of succinic acid production from sugarcane bagasse. ACS Sustain. Chem. Eng. 9, 12738–12746. doi:10.1021/acssuschemeng.1c02483
Shi, L., Liu, S., Li, X., Huang, X., Luo, H., Bai, Q., et al. (2023). Droplet microarray platforms for high-throughput drug screening. Microchim. Acta 190, 260. doi:10.1007/s00604-023-05833-9
Song, Y., Xie, L., Zhang, X., Hu, Z., Li, S., Zhang, P., et al. (2024). Enhancement of biomass, lipid accumulation, and carbon sequestration potential in microalgae via cultivation with Aggregation-Induced emission Light-Conversion films. Chem. Eng. J. 483, 149148. doi:10.1016/j.cej.2024.149148
Sorokina, K. N., Samoylova, Y. V., Gromov, N. V., Ogorodnikova, O. L., and Parmon, V. N. (2020). Production of biodiesel and succinic acid from the biomass of the microalga Micractinium sp. IC-44. Bioresour. Technol. 317, 124026. doi:10.1016/j.biortech.2020.124026
Stylianou, E., Carvajal-Arroyo, J. M., Ladakis, D., Lin, C. S. K., Eßmann, V., Dörr, S., et al. (2023). Development of an electrochemical membrane bioreactor for succinic acid production and in situ separation with engineered Yarrowia lipolytica cultivated on municipal biowaste hydrolysate. Chem. Eng. J. 466, 142877. doi:10.1016/j.cej.2023.142877
Stylianou, E., Pateraki, C., Ladakis, D., Cruz-Fernández, M., Latorre-Sánchez, M., Coll, C., et al. (2020). Evaluation of organic fractions of municipal solid waste as renewable feedstock for succinic acid production. Biotechnol. Biofuels 13, 72. doi:10.1186/s13068-020-01708-w
Stylianou, E., Pateraki, C., Ladakis, D., Damala, C., Vlysidis, A., Latorre-Sánchez, M., et al. (2021). Bioprocess development using organic biowaste and sustainability assessment of succinic acid production with engineered Yarrowia lipolytica strain. Biochem.Eng. J. 174, 108099. doi:10.1016/j.bej.2021.108099
Su, X., Xue, Q., Sun, M., Liu, J., Wong, M. H., Wang, C., et al. (2021). Co-production of polysaccharides, ginsenosides and succinic acid from Panax ginseng residue: a typical industrial herbal waste. Bioresour. Technol. 331, 125073. doi:10.1016/j.biortech.2021.125073
Tan, J. P., Jahim, J. M., Wu, T. Y., Harun, S., Kim, B. H., and Mohammad, A. W. (2014). Insight into biomass as a renewable carbon source for the production of succinic acid and the factors affecting the metabolic flux toward higher succinate yield. Ind. Eng. Chem. Res. 53, 16123–16134. doi:10.1021/ie502178j
Tapin, B., Khanh Ly, B., Canaff, C., Epron, F., Pinel, C., Besson, M., et al. (2020). Characterization by X-ray absorption spectroscopy of bimetallic Re–Pd/TiO2 catalysts efficient for selective aqueous-phase hydrogenation of succinic acid to 1,4-butanediol. Mat. Chem. Phys. 252, 123225. doi:10.1016/j.matchemphys.2020.123225
Thanahiranya, P., Charoensuppanimit, P., Sadhukhan, J., Soottitantawat, A., Arpornwichanop, A., Thongchul, N., et al. (2023). Succinic acid production from glycerol by Actinobacillus succinogenes: techno-economic, environmental, and exergy analyses. J. Clean. Prod. 404, 136927. doi:10.1016/j.jclepro.2023.136927
Thuy, N. T. H., Kongkaew, A., Flood, A., and Boontawan, A. (2017). Fermentation and crystallization of succinic acid from Actinobacillus succinogenes ATCC55618 using fresh cassava root as the main substrate. Bioresour. Technol. 233, 342–352. doi:10.1016/j.biortech.2017.02.114
Tomita, A., Zhang, M., Jin, F., Zhuang, W., Takeda, H., Maruyama, T., et al. (2017). ATP-dependent modulation of MgtE in Mg2+ homeostasis. Nat. Commun. 8, 148. doi:10.1038/s41467-017-00082-w
Valle, A., Haïlaf, A., Ceballos, A., Cantero, D., and Bolivar, J. (2021). Co-overexpression of the malate dehydrogenase (Mdh) and the malic enzyme A (MaeA) in several Escherichia coli mutant backgrounds increases malate redirection towards hydrogen production. Int. J. Hydrogen Energy 46, 15337–15350. doi:10.1016/j.ijhydene.2021.02.100
Vallecilla-Yepez, L., Ramchandran, D., Long, D., Saha, R., and Wilkins, M. R. (2021). Corn fiber as a biomass feedstock for production of succinic acid. Bioresour. Technol. Rep. 16, 100868. doi:10.1016/j.biteb.2021.100868
Vuoristo, K. S., Mars, A. E., Sanders, J. P. M., Eggink, G., and Weusthuis, R. A. (2016). Metabolic engineering of TCA cycle for production of chemicals. Trends Biotechnol. 34, 191–197. doi:10.1016/j.tibtech.2015.11.002
Wang, C., Yan, D., Li, Q., Sun, W., and Xing, J. (2014a). Ionic liquid pretreatment to increase succinic acid production from lignocellulosic biomass. Bioresour. Technol. 172, 283–289. doi:10.1016/j.biortech.2014.09.045
Wang, H., Pan, J., Wang, J., Wang, N., Zhang, J., Li, Q., et al. (2014b). Succinic acid production from xylose mother liquor by recombinant Escherichia coli strain. Biotechnol. Biotechnol. Equip. 28, 1042–1049. doi:10.1080/13102818.2014.952501
Wang, J., Qin, D., Zhang, B., Li, Q., Li, S., Zhou, X., et al. (2015). Fine-tuning of ecaA and pepc gene expression increases succinic acid production in Escherichia coli. Appl. Microbiol. Biotechnol. 99, 8575–8586. doi:10.1007/s00253-015-6734-7
Wang, J., Zhang, B., Zhang, J., Wang, H., Zhao, M., Wang, N., et al. (2014c). Enhanced succinic acid production and magnesium utilization by overexpression of magnesium transporter mgtA in Escherichia coli mutant. Bioresour. Technol. 170, 125–131. doi:10.1016/j.biortech.2014.07.081
Woo, W. X., Nasoha, N. Z. B., Luthfi, A. a.I., Yeap, S. K., Hui, Y. W., Bukhari, N. A., et al. (2023). Bio-based succinic acid production from durian husk: a rising Southeast Asia agricultural waste. Ind. Crop. Prod. 206, 117624. doi:10.1016/j.indcrop.2023.117624
Xi, Y., Ye, L., and Yu, H. (2022). Enhanced thermal and alkaline stability of L-lysine decarboxylase CadA by combining directed evolution and computation-guided virtual screening. Bioresour. Bioprocess. 9, 24. doi:10.1186/s40643-022-00510-w
Xu, C., Alam, M. A., Wang, Z., Peng, Y., Xie, C., Gong, W., et al. (2021). Co-fermentation of succinic acid and ethanol from sugarcane bagasse based on full hexose and pentose utilization and carbon dioxide reduction. Bioresour. Technol. 339, 125578. doi:10.1016/j.biortech.2021.125578
Xu, H., Zhou, Z., Wang, C., Chen, Z., and Cai, H. (2016). Enhanced succinic acid production in Corynebacterium glutamicum with increasing the available NADH supply and glucose consumption rate by decreasing H+-ATPase activity. Biotechnol. Lett. 38, 1181–1186. doi:10.1007/s10529-016-2093-4
Xu, P., Li, J., Qian, J., Wang, B., Liu, J., Xu, R., et al. (2023). Recent advances in CO2 fixation by microalgae and its potential contribution to carbon neutrality. Chemosphere 319, 137987. doi:10.1016/j.chemosphere.2023.137987
Xu, S., Hao, N., Xu, L., Liu, Z., Yan, M., Li, Y., et al. (2015). Series fermentation production of ornithine and succinic acid from cane molasses by Corynebacterium glutamicum. Biochem. Eng. J. 99, 177–182. doi:10.1016/j.bej.2015.03.017
Yan, D., Wang, C., Zhou, J., Liu, Y., Yang, M., and Xing, J. (2014). Construction of reductive pathway in Saccharomyces cerevisiae for effective succinic acid fermentation at low pH value. Bioresour. Technol. 156, 232–239. doi:10.1016/j.biortech.2014.01.053
Yang, G., Tan, H., Li, S., Zhang, M., Che, J., Li, K., et al. (2020). Application of engineered yeast strain fermentation for oligogalacturonides production from pectin-rich waste biomass. Bioresour. Technol. 300, 122645. doi:10.1016/j.biortech.2019.122645
Yang, X., Wang, H., Li, C., and Lin, C. S. K. (2017). Restoring of glucose metabolism of engineered Yarrowia lipolytica for succinic acid production via a simple and efficient adaptive evolution strategy. J. Agric. Food Chem. 65, 4133–4139. doi:10.1021/acs.jafc.7b00519
Yang, Y., Xu, W., Wang, Y., Shen, J., Wang, Y., Geng, Z., et al. (2022). Progress of CCUS technology in the iron and steel industry and the suggestion of the integrated application schemes for China. Chem. Eng. J. 450, 138438. doi:10.1016/j.cej.2022.138438
Yang, Z., Wu, W., Zhao, Q., Angelidaki, I., Arhin, S. G., Hua, D., et al. (2024). Enhanced direct gaseous CO2 fixation into higher bio-succinic acid production and selectivity. J. Environ. Sci. 143, 164–175. doi:10.1016/j.jes.2023.05.035
Yao Zhang, X. Q., Chen, C., Yu, Z., and Hong, H. (2020). Recent progress in microbial production of succinic acid. CIESC J. 71, 1964–1975. doi:10.11949/0438-1157.20191430
Yin, Y., and Wang, J. (2022). Production of medium-chain fatty acids by co-fermentation of antibiotic fermentation residue with fallen Ginkgo leaves. Bioresour. Technol. 360, 127607. doi:10.1016/j.biortech.2022.127607
Yu, J., Zhu, L.-W., Xia, S.-T., Li, H.-M., Tang, Y., Liang, X.-H., et al. (2016). Combinatorial optimization of CO2 transport and fixation to improve succinate production by promoter engineering. Biotechnol. Bioeng. 113, 1531–1541. doi:10.1002/bit.25927
Zaidi, S., Srivastava, N., and Kumar Khare, S. (2022). Microbial carbonic anhydrase mediated carbon capture, sequestration and utilization: a sustainable approach to delivering bio-renewables. Bioresour. Technol. 365, 128174. doi:10.1016/j.biortech.2022.128174
Zerva, A., Pentari, C., Ferousi, C., Nikolaivits, E., Karnaouri, A., and Topakas, E. (2021). Recent advances on key enzymatic activities for the utilisation of lignocellulosic biomass. Bioresour. Technol. 342, 126058. doi:10.1016/j.biortech.2021.126058
Zhang, F., Li, J., Liu, H., Liang, Q., and Qi, Q. (2016). ATP-based ratio regulation of glucose and xylose improved succinate production. PLOS ONE 11, e0157775. doi:10.1371/journal.pone.0157775
Zhang, J., Li, K., Liu, S., Huang, S., and Xu, C. (2022). Alkaline hydrogen peroxide pretreatment combined with bio-additives to boost high-solids enzymatic hydrolysis of sugarcane bagasse for succinic acid processing. Bioresour. Technol. 345, 126550. doi:10.1016/j.biortech.2021.126550
Zhang, Q., Cheng, C.-L., Nagarajan, D., Chang, J.-S., Hu, J., Lee, D.-J., et al. (2017). Carbon capture and utilization of fermentation CO2: integrated ethanol fermentation and succinic acid production as an efficient platform. Appl. Energy 206, 364–371. doi:10.1016/j.apenergy.2017.08.193
Zhang, X., Jantama, K., Moore, J. C., Jarboe, L. R., Shanmugam, K. T., and Ingram, L. O. (2009). Metabolic evolution of energy-conserving pathways for succinate production in Escherichia coli. PNAS 106, 20180–20185. doi:10.1073/pnas.0905396106
Zhao, Y., Cao, W., Wang, Z., Zhang, B., Chen, K., and Ouyang, P. (2016). Enhanced succinic acid production from corncob hydrolysate by microbial electrolysis cells. Bioresour. Technol. 202, 152–157. doi:10.1016/j.biortech.2015.12.002
Zhu, L. W., Xia, S. T., Wei, L. N., Li, H. M., Yuan, Z. P., and Tang, Y. J. (2016). Enhancing succinic acid biosynthesis in Escherichia coli by engineering its global transcription factor, catabolite repressor/activator (Cra). Sci. Rep. 6, 36526. doi:10.1038/srep36526
Zhu, X., Tan, Z., Xu, H., Chen, J., Tang, J., and Zhang, X. (2014). Metabolic evolution of two reducing equivalent-conserving pathways for high-yield succinate production in Escherichia coli. Metab. Eng. 24, 87–96. doi:10.1016/j.ymben.2014.05.003
Keywords: succinic acid, CO2 sequestration, waste biomass, Bioelectrochemical, Bioreactors, high-throughput screening
Citation: Lin F, Li W, Wang D, Hu G, Qin Z, Xia X, Hu L, Liu X and Luo R (2024) Advances in succinic acid production: the enhancement of CO2 fixation for the carbon sequestration benefits. Front. Bioeng. Biotechnol. 12:1392414. doi: 10.3389/fbioe.2024.1392414
Received: 27 February 2024; Accepted: 18 March 2024;
Published: 28 March 2024.
Edited by:
Rongzhen Zhang, Jiangnan University, ChinaReviewed by:
Xiaofeng Yang, South China University of Technology, ChinaSha Li, Nanjing Tech University, China
Carol Sze Ki Lin, City University of Hong Kong, Hong Kong SAR, China
Yong Fan, Chinese Academy of Sciences (CAS), China
Copyright © 2024 Lin, Li, Wang, Hu, Qin, Xia, Hu, Liu and Luo. This is an open-access article distributed under the terms of the Creative Commons Attribution License (CC BY). The use, distribution or reproduction in other forums is permitted, provided the original author(s) and the copyright owner(s) are credited and that the original publication in this journal is cited, in accordance with accepted academic practice. No use, distribution or reproduction is permitted which does not comply with these terms.
*Correspondence: Dan Wang, dwang@cqu.edu.cn
†These authors have contributed equally to this work and share first authorship