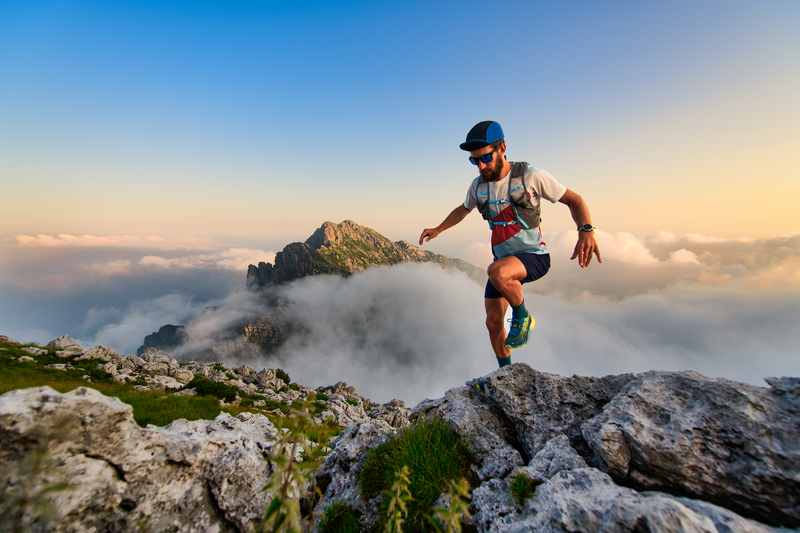
95% of researchers rate our articles as excellent or good
Learn more about the work of our research integrity team to safeguard the quality of each article we publish.
Find out more
REVIEW article
Front. Bioeng. Biotechnol. , 17 February 2022
Sec. Biomaterials
Volume 10 - 2022 | https://doi.org/10.3389/fbioe.2022.853193
This article is part of the Research Topic Functional and Smart Biomaterials: Development and Application in Regenerative Medicine View all 18 articles
Currently, patients with esophageal cancer, especially advanced patients, usually use autologous tissue for esophageal alternative therapy. However, an alternative therapy is often accompanied by serious complications such as ischemia and leakage, which seriously affect the prognosis of patients. Tissue engineering has been widely studied as one of the ideal methods for the treatment of esophageal cancer. In view of the complex multi-layer structure of the natural esophagus, how to use the tissue engineering method to design the scaffold with structure and function matching with the natural tissue is the principle that the tissue engineering method must follow. This article will analyze and summarize the construction methods, with or without cells, and repair effects of single-layer scaffold and multi-layer scaffold. Especially in the repair of full-thickness and circumferential esophageal defects, the flexible design method and the binding force between the layers of the scaffold are very important. In short, esophageal tissue engineering technology has broad prospects and plays a more and more important role in the treatment of esophageal diseases.
Esophageal cancer is the seventh most common cancer in the world and ranks sixth in the world in terms of lethality among all malignant tumors. Of the 500,000 new cases worldwide each year, about half occur in China. It is the fifth most commonly diagnosed cancer and the fourth leading cause of cancer death in China. The fatality rate of esophageal cancer remains high, which seriously affects people’s lives and health (Pennathur et al., 2013; Chen et al., 2016; Chang et al., 2017; Huang et al., 2018; Uhlenhopp et al., 2020). Surgical alternative therapy requires replacement of the stomach, jejunum, colon, and other autologous tissues, but replacement is likely to cause high morbidity and mortality, and at the cost of normal tissue damage, it seriously affects the quality of life (Dua et al., 2016; Arakelian et al., 2018). In recent years, tissue engineering technology has been used to construct bionic esophageal scaffolds, which avoids taking materials from patients, reduces the risk of high-risk surgery and high mortality and morbidity caused by postoperative surgery, and provides a new method for esophageal repair and reconstruction (Zhu et al., 2017). The esophagus is composed of the mucosa, submucosa, muscularis propria, and adventitia. The muscularis propria is a multi-layer structure with an inner ring and an outer longitudinal shape, called the circular muscle and the longitudinal muscle. The cells mainly include mucosal epithelial cells (ECs) and smooth muscle cells (SMCs) (Peirlinck et al., 2018; Blank et al., 2019; Farhat et al., 2021) (Figure 1). Therefore, as an ideal esophageal tissue engineering scaffold and a bionic multi-layer structure of the esophagus, it is endowed with corresponding supporting functions for different parts. How to combine each layer of scaffold effectively is one of the important problems that must be considered when constructing a multi-layer scaffold. In view of this, how to design a bionic multi-layer composite scaffold, which has both multi-layer structure and multi-function and ensures the firm connection between each layer, is a scientific problem of great concern in this field and has important scientific significance and potential application value.
FIGURE 1. Structure of the human esophagus. (A) Position of the esophagus in the human body. (B) Schematic diagram of cross section of the esophagus.
In the research of esophageal tissue engineering, researchers have conducted a large number of innovative research studies on the construction of single-layer scaffolds to repair the mucosal layer or the muscle layer. Single-layer scaffolds are classified according to the choice of materials, mainly including acellular matrix or (and) polymer scaffolds. The acellular matrix includes the small intestinal submucosa (SIS), urinary bladder submucosa (UBS), esophageal mucosa, etc. Polymer materials include polylactide (PLA), poly (l-lactide-co-ε-caprolactone) (PLGA), poly (3-hydroxybutyrate-co-3-hydroxyvalerate) (PHBV), polylactide-poly (ɛ-caprolactone) (PLA-PCL), polyurethane (PU), etc. (Badylak et al., 2000; Nieponice et al., 2014; Kuppan et al., 2016; Tan et al., 2016; Dorati et al., 2017; Kuppan et al., 2017; Luc et al., 2018) (Table 1).
From the perspective of single-layer scaffold structure, the classification mainly includes acellular matrix, membranes grafted with biomolecules, electrospinning scaffolds, micro-pattern scaffolds, etc. (Hou et al., 2016; Wei et al., 2018; Zhuravleva et al., 2019; Chaitin et al., 2021; Levenson et al., 2021) (Table 2). In order to accurately simulate the inner ring and outer longitudinal structure of the muscle layer, electrospinning scaffolds and micro-pattern scaffolds are mainly used (Gong et al., 2013; Kuppan et al., 2017). Our team has conducted a large number of in vitro and in vivo animal studies on single-layer scaffolds. The original idea was to graft collagen on the surface of polymer materials such as PLGA and poly (l-lactide-co-caprolactone) (PLLC) to improve their biocompatibility (Zhu et al., 2005; Zhu et al., 2006). Then, the polycaprolactone/silk fibroin (PCL/SF) electrospinning scaffold is made by electrospinning technology, and the electrospinning fiber pore size can simulate the structure of the extracellular matrix (ECM) (Lv et al., 2014). At this stage, the micro-pattern membrane technology is used to construct a new type of esophageal bionic scaffold, which has successfully constructed a micro-pattern PU scaffold and double-layer scaffold of the esophageal acellular matrix (Hou et al., 2019; Wang X. et al., 2020).
The abovementioned studies carried out the structural bionic design of single-layer scaffolds from the perspectives of material selection and construction methods and achieved a series of achievements in the damage repair of the esophageal mucosa and muscularis. Studies have shown that if cells are introduced into scaffolds, they will work synergistically with scaffolds in the microenvironment in vivo to further enhance functional repair of tissues. For example, Badylak et al. made artificial defects in the dog’s esophagus and used ECM derived from SIS or UBS to repair the esophageal defect. After 35 days, the scaffold was partially covered by the squamous epithelium, and only scattered skeletal muscle cells surrounded collagen connective tissue (Badylak et al., 2000). Xie et al. proved that, after implantation in dogs, SIS alone is not completely endothelialized. When bone marrow mesenchymal stem cells (MSCs) are combined with SIS, the results show that the defect site is completely endothelialized, the muscle layer is regenerated, and the new microvessels are dense (Tan et al., 2013) (Figure 2).
FIGURE 2. I. Repair of a canine esophageal defect with SIS combined with MSCs. (A) SIS + MSCs. (B) Simple SIS. (C, D) Barium esophagus examination. (E, F) H&E staining of canine esophagus tissue and (G, H) its partial magnification. (I–L) Immunofluorescence staining to detect the expression of living cell marker proteins PKH-26 and α-SMA in canine esophagus tissue (Tan et al., 2013). II. Esophageal muscular acellular matrix repairs porcine esophageal defects: (K) Porcine esophageal muscular acellular matrix. (L) Microscopic perforation treatment. (M, N) Schematic diagram of MSC growth in the acellular matrix scaffold. (O, P) Immunohistochemical staining to detect the expression of actin and desmin in porcine esophagus tissue (Marzaro et al., 2020).
Therefore, researchers have introduced cells, such as ECs, SMCs, and stem cells, into biomimetic single-layer scaffolds to construct tissue engineering scaffolds. The ECs or SMCs are difficult to be widely used due to the large damage to the donor when they are acquired and the limited ability of proliferation and differentiation after cell expansion. On the contrary, stem cells have the advantages of large differentiation potential, strong proliferation ability, convenient and easy acquisition from the body, and the ability to differentiate into specific cells in tissue. So, they are widely used as seed cells in the field of tissue engineering. For example, Ivo et al. combined MSCs and decellularized esophageal muscle tissue to repair the esophagus in situ in pigs, which showed new muscle tissue compared with the decellularized esophageal muscle layer alone (Marzaro et al., 2020) (Figure 2). Aho et al. Using PU material, autologous adipose-derived mesenchymal stem cells were seeded to form a cell-span esophageal implant (CEI). After resection of the patient’s esophageal cancer, in situ repair was performed using CEI and followed by esophagogastroduodenoscopy (EGD). After the patient’s death, histological examination revealed esophageal luminal epithelialization and partial muscle regeneration 7.5 months after scaffold implantation (Aho et al., 2021) (Figure 3).
FIGURE 3. PU combined with MSCs for reconstruction of the human esophagus. (A) EGD image of the esophagus after scaffold placement. (B) EGD image of the esophagus after scaffold deployment. (C) The removed CEI scaffold assembly is adhered to the scaffold. (D) EGD image of esophageal neoplastic tissue. (E) Histological examination of esophageal sections, including H&E staining, Masson staining, and immunohistochemical staining to detect the expression of α-SMA (Aho et al., 2021).
Therefore, single-layer bionic scaffold combined with stem cells to repair the mucosal layer or muscle layer is the current main research direction. On the basis of mucosal layer or muscle layer repair, higher clinical requirements for esophageal repair are proposed, such as full-thickness or circumferential defect, which requires simultaneous repair of the mucosal layer, submucosal layer, and muscle layer of the esophagus. Therefore, it is particularly important to design a multi-layer functional bionic scaffold.
Researchers have studied full-thickness or circumferential defects by designing lamellar or tubular bionic scaffolds. According to the research methods, it is mainly divided into two categories: scaffolds and scaffolds/cells composite. The scaffolds were prepared by the one-step method or multi-step method. The one-step method is to mix the scaffold material into a whole through melting, electrostatic spinning, temperature-induced sedimentation, etc., and different components complement each other and work in synergy. For example, Tan et al. melted PCL/PLA and stretched it into a directional spinning tubular structure (Tan et al., 2016). The multi-step method is based on the perspective of esophageal structure bionics, combining different layers of scaffold materials through a certain link method. Joshua et al. prepared the silk fibroin double-layer scaffold by solution pouring (Gundogdu et al., 2021). Rossella et al. used electrospinning and temperature-induced sedimentation to construct two double-layer scaffolds (Pisani et al., 2020). Saverio et al. designed a PU electrospinning three-layer scaffold (inner and outer layer pore diameter>10 μm; middle layer <10 μm) (Soliman et al., 2019) (Table 3).
Through the abovementioned research and analysis, it can be seen that the design of multi-layer scaffolds is the guiding ideology of bionics, but these scaffolds still cannot completely induce the structural growth of tissues. Therefore, researchers have constructed scaffolds with cells to enhance the repair function of tissue. Natural materials such as esophageal acellular matrix, SIS, and collagen scaffold are compounded with cells.
Guillaume et al. designed the esophageal mucosal acellular matrix/omentum double-layer scaffold, in which MSCs were cultured on the acellular matrix, and the omentum re-matured in pigs. As a result, it was found that 3 months after the esophageal replacement surgery, a new epithelium and muscle regeneration were visible (Levenson et al., 2021) (Figure 4). Paola et al. used the method of organoid culture to construct a multi-layer esophageal scaffold with cells in vitro. The researchers re-seeded ECs on the acellular matrix of the rat esophageal mucosa and allowed the cells to grow in the lumen of the acellular scaffold to construct the esophageal mucosal layer and co-cultured human or mouse fibroblasts and mouse neural crest cells in vitro. The muscle layer is constructed and then implanted into the rat omentum for in vivo culture to promote angiogenesis and build a multi-layer esophageal structure together with the mucosal layer. This kind of esophageal tissue composed of cells is more complete than the commonly used acellular matrix and other natural materials, but it needs to be verified by animal experiments to prove its positive significance (Urbani et al., 2018) (Figure 4).
FIGURE 4. Porcine esophageal acellular matrix and omentum construct a double-layer scaffold to repair esophageal defects. (A) Acellular matrix. (B) Decellularized matrix composite omentum. (C) Omentum maturation in pigs. (D) H&E staining of pig esophagus sections. (E) Immunohistochemical staining to detect the expression of desmin in porcine esophagus tissue (Levenson et al., 2021). II. The PLGA/PCL electrospinning scaffold was prepared by the one-step method combined with ECs and myocytes to repair esophageal defects: (F) Cells were cultured on the inner and outer surfaces of the scaffold. (G) The scaffold is a patch to repair esophageal injury in rats. (H, I) H&E staining of rat esophagus sections (Jensen et al., 2015). III. PU electrospun scaffolds combined with mucosal cells to construct composite scaffolds involved in porcine esophagus reconstruction: (J) Schematic diagram of electrospinning. (K) Composite scaffold for in situ replacement of the esophagus. (L, M) H&E staining of porcine esophagus sections. (N, O) Immunofluorescence staining was used to detect the expression of α-SMA in porcine esophagus tissue (Barron et al., 2018).
In addition to natural materials, synthetic polymer materials such as PLGA, PCL, and PU have also been studied in combination with cells. For example, Christine et al. prepared a PLGA/PCL electrospun tubular esophageal scaffold, the inner cavity of the scaffold was compounded with autologous ECs, and the outer side was compounded with autologous SMCs. The composite scaffolds containing cells were cultured in an in vitro bioreactor for a period of time and then implanted into the mouse esophagus in situ, the esophagus is still viable after 2 weeks, and the cells maintain the phenotype (Jensen et al., 2015) (Figure 4). Dennis et al. combined porcine esophageal mucosal cells and electrospun PU scaffold into a tubular scaffold and implanted it into the whole-peripheral defect of the porcine esophagus, and the results showed that the mucosal layer, submucosa, and muscle layer of the esophagus regenerate simultaneously and have abundant blood vessels (Barron et al., 2018) (Figure 4).
Different from the traditional scaffolds, 3D printing scaffolds have many advantages, such as the flexibility of preparation methods, the customization of irregular tissue damage parts, and the ability to prepare scaffolds with very complex structures (Memic et al., 2017; Matai et al., 2020; Wan et al., 2020; Barros et al., 2021; Wu et al., 2021; Yang et al., 2021). The 3D printing scaffolds have been studied in esophageal repair. For example, Chung et al. used a 3D melt extrusion method to construct a polycaprolactone (PCL) 3D printing scaffold, seeded MSCs on the scaffold to participate in esophageal reconstruction, cells grew along the direction of the scaffold, and implanted it in the defect of the rat esophagus. The results show that the new tissue repaired by the 3D printing scaffold is similar to natural tissue and has obvious advantages compared with electrospun PU scaffolds (Figure 5) (Park et al., 2021). Although 3D printing scaffolds have many advantages, this method also has its own limitations, such as lack of diversity of bio-ink, harsh printing conditions (high temperature or UV curing), and expensive equipment for printing cells.
FIGURE 5. 3D printed PCL scaffold and electrospun PU scaffold combined with MSCs to repair the esophageal defect. (A) Schematic diagram of the 3D printed PCL scaffold and electrospun PU scaffold. (B,C) The live/dead cell assay on the scaffold surface was studied. (D–F) H&E staining of rat esophagus sections. (G–I) Masson staining of rat esophagus sections. (J–L) Elastic fiber staining of rat esophagus sections (Park et al., 2021).
The mixed use of polymer synthetic materials and natural materials has gradually become the focus of research. For example, Jonathan et al. used electrospinning technology to make PLGA fiber layers on the SIS acellular matrix to form a double-layer esophageal scaffold, the results showed that human esophageal smooth muscle cell culture experiments and subcutaneous embedding presented good biocompatibility (Syed et al., 2019), but further research is needed for in vivo repair.
Our group’s previous study used micro-pattern technology to construct a three-layer scaffold, which corresponds to the inner ring muscle (S1), outer longitudinal muscle (S2), and mucosal layer (S3) of the esophagus. After inoculating MSCs on the composite scaffold, it was implanted into the esophageal defect. The results showed that there was new esophageal tissue, including the muscle layer and mucosal layer. However, the PU material can still be found in the tissue 180 days after implantation, which may affect the speed of muscle regeneration (Wang X. et al., 2020) (Figure 6).
FIGURE 6. Multi-layer esophageal scaffold combined with stem cells to repair esophageal defect in rabbits. (A) Schematic diagram of the three-layer scaffold; S1, S2, and S3, respectively, represent the inner ring muscle, outer longitudinal muscle, and mucosal layer of the esophagus. (B–D) H&E staining of rabbit esophagus sections at 180 days (E–G) Western blot evaluated the expression of α-SMA (S1, S2) and CK-14 (S3), respectively, in rabbit esophageal defect. (H–J) Quantitative calculation of (E–G) using ImageJ software (n = 3). *p < 0.05 (Wang X. et al., 2020).
In conclusion, although studies on the repair of the full-thickness or circumferential defect of the esophagus have achieved many tentative results, the following key issues still need to be further developed and improved, in order to obtain a bionic scaffold that is closer to the natural esophageal structure and function, so as to be used in the clinical treatment of esophageal cancer patients as soon as possible and benefit mankind.
At present, there are two main construction methods of esophageal scaffold, one-step construction and multi-step construction. The advantage of one-step construction is that it is relatively simple, only need one or more types of biomaterials are required, and generally, the binding force between the support layers is strong and stable. However, the disadvantage is that the material and function are relatively single, so it is very difficult to accurately simulate the multi-layer structure of the esophagus and give specific functions to each layer of the scaffold. Since the multi-layer scaffold prepared by the multi-step construction method is flexible, it can provide different materials (natural materials, synthetic materials, or both) and cells (ECs, SMCs, or stem cells) for each layer of the scaffold, so as to more accurately mimic the structure and function of the esophagus. Therefore, it is the current development direction to prepare an accurate bionic multi-layer esophageal scaffold by multi-step construction.
For multi-layer scaffolds prepared by multi-step construction, the firmness between the scaffolds is another key issue. If the adhesion between scaffolds is poor, the multi-layer scaffolds will fall off or shift, which will seriously affect the repair effect of the esophagus. The connection modes between the layers of multi-layer scaffolds include the solution casting method (Gundogdu et al., 2021), temperature-induced precipitation method (Dorati et al., 2017), solvent volatilization method of electrospinning (Chung et al., 2015), and glue bonding (Deng et al., 2019). The first three methods are not universally applicable because solutions or solvents may dissolve the active components such as protein, growth factor in the scaffold, and too high or low temperature is not conducive to the introduction of proteins and cells into the scaffold. Glue bonding does not affect the design of each layer of the scaffold, as long as the scaffold prepared separately is combined, which is a simple combination method with universal applicability. Generally, it is relatively easy for the glue to adhere to objects in a dry environment, but it remains a great challenge for repair in a wet environment (exudate or blood at the injury) or dynamic adhesion (human movement).
At present, the tissue glue used in the clinical treatment of esophageal anastomotic fistula is mainly cyanoacrylate (superglue, highly toxic, and rarely used) and fibrin glue (fibrin glue, frequently used, but with low adhesion ability) (Rao et al., 2018). The adhesion strength and adhesion energy of fibrin glue are about 10 kPa and 10 Jm−2, respectively (Deng et al., 2019). As the esophagus is a soft tissue with peristalsis and swallowing functions, higher requirements are put forward for the glue used to bond the multi-layer esophageal scaffold (>>10 kPa and >>10 Jm−2). New adhesives, such as nano-clay/multi-walled carbon nanotubes/isopropylacrylamide hydrogel (adhesive strength 7 kPa) (Deng et al., 2019), sodium p-styrene sulfonate/chloromethane quaternized dimethylaminoethyl acrylate hydrogel (adhesive strength 25 kPa, adhesive energy 50 Jm-2) (Rao et al., 2018), chitosan/double-bonded phenylalanine hydrogel (adhesive strength 14 kPa) (Sharma et al., 2019), aldehyde functionalized hyaluronic acid/3,3′-dithiobis (propionyl hydrazide) hydrogel (adhesive strength 120 kPa) (Sigen et al., 2021), polyethylene glycol/lysozyme hydrogel (adhesive strength 32 kPa) (Tan et al., 2019), folic acid/polydimethyl diallyl ammonium chloride hydrogel (adhesive strength 150 kPa) (Gao et al., 2021), and hyaluronic acid/catechol/horseradish peroxidase hydrogels (17 kPa) (Wang D. et al., 2020) (Figure 7), are used. The hydrogels mentioned above can only meet one of the requirements of adhesion or cytocompatibility. Therefore, it is the research direction of adhesiveness of hydrogel to satisfy high adhesion and biocompatibility in a complex environment.
FIGURE 7. Polyethylene glycol/lysozyme hydrogel adheres to the defect site of the left ventricular wall in rabbits. (A–F) Hydrogel participates in the process of sealing the left ventricular defect (Tan et al., 2019). II. Adhesion experiments of aldehyde-functionalized hyaluronic acid/3,3′-dithiobis (propionyl hydrazide) hydrogels. (G,J) Hydrogels adhered to various substrate surfaces (Sigen et al., 2021).
Compared with traditional methods, esophageal tissue engineering technology has become a promising alternative method for the treatment of esophageal injury. The multi-layer complex structure of the esophagus should be considered in the repair of the full-thickness or circumferential defect of the esophagus, and how to obtain an ingenious design and retain the bionic structure and bionic function are the research direction. To solve these problems, the multi-step method is more favorable for the preparation of scaffolds; for example, glue bonding and 3D printing methods are two of the flexible styles to fabricate bionic scaffolds. It is believed that more and more perfect scaffolds will emerge in the near future and achieve more effective repair effects.
RH conceived the initial idea and the conceptualization. RX drafted the manuscript. XF, SW, YW, YZ, LZ, LS, and QP participated in data collection. RH, JZ, XC, RZ, LY, and YZ revised the manuscript. All authors contributed to the article and approved the submitted version.
This work was supported by the National Natural Science Foundation of China (No. 32000942), the Natural Science Foundation of Zhejiang (No. LY20C100001), the Natural Science Foundation of Ningbo (Nos. 2021J071 and 202003N4238), the Major Project of 2025 Sci and Tech Innovation of Ningbo (Nos. 2018B10052 and 2020Z096), the State Key Laboratory of Fluid Power and Mechatronic Systems (No. GZKF-202024), and the General scientific Research Project of Zhejiang Education Department (No. Y202044155).
The authors declare that the research was conducted in the absence of any commercial or financial relationships that could be construed as a potential conflict of interest.
All claims expressed in this article are solely those of the authors and do not necessarily represent those of their affiliated organizations, or those of the publisher, the editors, and the reviewers. Any product that may be evaluated in this article, or claim that may be made by its manufacturer, is not guaranteed or endorsed by the publisher.
The authors would like to thank the Core Facilities, Ningbo University School of Medicine, for the technical support.
A, S., Xu, Q., Johnson, M., Creagh-Flynn, J., Venet, M., Zhou, D., et al. (2021). An Injectable Multi-Responsive Hydrogel as Self-Healable and On-Demand Dissolution Tissue Adhesive. Appl. Mater. Today 22, 100967. doi:10.1016/j.apmt.2021.100967
Aho, J. M., La Francesca, S., Olson, S. D., Triolo, F., Bouchard, J., Mondano, L., et al. (2021). First-in-Human Segmental Esophageal Reconstruction Using a Bioengineered Mesenchymal Stromal Cell-Seeded Implant. JTO Clin. Res. Rep. 2 (9), 100216. doi:10.1016/j.jtocrr.2021.100216
Arakelian, L., Kanai, N., Dua, K., Durand, M., Cattan, P., and Ohki, T. (2018). Esophageal Tissue Engineering: from Bench to Bedside. Ann. N.Y. Acad. Sci. 1434 (1), 156–163. doi:10.1111/nyas.13951
Badylak, S., Meurling, S., Chen, M., Spievack, A., and Simmons-Byrd, A. (2000). Resorbable Bioscaffold for Esophageal Repair in a Dog Model. J. Pediatr. Surg. 35 (7), 1097–1103. doi:10.1053/jpsu.2000.7834
Barron, M. R., Blanco, E. W., Aho, J. M., Chakroff, J., Johnson, J., Cassivi, S. D., et al. (2018). Full-thickness Oesophageal Regeneration in Pig Using a Polyurethane Mucosal Cell Seeded Graft. J. Tissue Eng. Regen. Med. 12 (1), 175–185. doi:10.1002/term.2386
Barros, A. S., Costa, A., and Sarmento, B. (2021). Building Three-Dimensional Lung Models for Studying Pharmacokinetics of Inhaled Drugs. Adv. Drug Deliv. Rev. 170, 386–395. doi:10.1016/j.addr.2020.09.008
Blank, R. S., Collins, S. R., Huffmyer, J. L., and Jaeger, J. M. (2019). “Anesthesia for Esophageal Surgery,” in Principles and Practice of Anesthesia for Thoracic Surgery. Editor P. Slinger (Cham: Springer International Publishing), 609–649. doi:10.1007/978-3-030-00859-8_38
Chaitin, H., Lu, M. L., Wallace, M. B., and Kang, Y. (2021). Development of a Decellularized Porcine Esophageal Matrix for Potential Applications in Cancer Modeling. Cells 10 (5), 1055. doi:10.3390/cells10051055
Chang, J., Tan, W., Ling, Z., Xi, R., Shao, M., Chen, M., et al. (2017). Genomic Analysis of Oesophageal Squamous-Cell Carcinoma Identifies Alcohol Drinking-Related Mutation Signature and Genomic Alterations. Nat. Commun. 8, 11. doi:10.1038/ncomms15290
Chen, W., Zheng, R., Baade, P. D., Zhang, S., Zeng, H., Bray, F., et al. (2016). Cancer Statistics in China, 2015. CA: A Cancer J. Clinicians 66 (2), 115–132. doi:10.3322/caac.21338
Chung, E.-J., Ju, H. W., Park, H. J., and Park, C. H. (2015). Three-layered Scaffolds for Artificial Esophagus Using Poly(ɛ-Caprolactone) Nanofibers and Silk Fibroin: An Experimental Study in a Rat Model. J. Biomed. Mater. Res. 103 (6), 2057–2065. doi:10.1002/jbm.a.35347
Deng, Z., Hu, T., Lei, Q., He, J., Ma, P. X., and Guo, B. (2019). Stimuli-Responsive Conductive Nanocomposite Hydrogels with High Stretchability, Self-Healing, Adhesiveness, and 3D Printability for Human Motion Sensing. ACS Appl. Mater. Inter. 11 (7), 6796–6808. doi:10.1021/acsami.8b20178
Dorati, R., De Trizio, A., Marconi, S., Ferrara, A., Auricchio, F., Genta, I., et al. (2017). Design of a Bioabsorbable Multilayered Patch for Esophagus Tissue Engineering. Macromol. Biosci. 17 (6), 1600426. doi:10.1002/mabi.201600426
Dua, K. S., Hogan, W. J., Aadam, A. A., and Gasparri, M. (2016). In-vivo Oesophageal Regeneration in a Human Being by Use of a Non-biological Scaffold and Extracellular Matrix. The Lancet 388 (10039), 55–61. doi:10.1016/s0140-6736(15)01036-3
Farhat, W., Chatelain, F., Marret, A., Faivre, L., Arakelian, L., Cattan, P., et al. (2021). Trends in 3D Bioprinting for Esophageal Tissue Repair and Reconstruction. Biomaterials 267, 120465. doi:10.1016/j.biomaterials.2020.120465
Gao, S., Wang, W., Wu, T., Jiang, S., Qi, J., Zhu, Z., et al. (2021). Folic Acid-Based Coacervate Leading to a Double-Sided Tape for Adhesion of Diverse Wet and Dry Substrates. ACS Appl. Mater. Inter. 13 (29), 34843–34850. doi:10.1021/acsami.1c06844
Gong, C., Hou, L., Zhu, Y., Lv, J., Liu, Y., and Luo, L. (2013). In Vitro Constitution of Esophageal Muscle Tissue with Endocyclic and Exolongitudinal Patterns. ACS Appl. Mater. Inter. 5 (14), 6549–6555. doi:10.1021/am401115z
Gundogdu, G., Morhardt, D., Cristofaro, V., Algarrahi, K., Yang, X., Costa, K., et al. (2021). Evaluation of Bilayer Silk Fibroin Grafts for Tubular Esophagoplasty in a Porcine Defect Model. Tissue Eng. A 27 (1-2), 103–116. doi:10.1089/ten.tea.2020.0061
Hou, L., Gong, C., and Zhu, Y. (2016). In Vitro construction and In Vivo Regeneration of Esophageal Bilamellar Muscle Tissue. J. Biomater. Appl. 30 (9), 1373–1384. doi:10.1177/0885328215627585
Hou, R., Wang, X., Wei, Q., Feng, P., Mou, X., Zhu, Y., et al. (2019). Biological Properties of a Bionic Scaffold for Esophageal Tissue Engineering Research. Colloids Surf. B: Biointerfaces 179, 208–217. doi:10.1016/j.colsurfb.2019.03.072
Huang, X., Zhou, X., Hu, Q., Sun, B., Deng, M., Qi, X., et al. (2018). Advances in Esophageal Cancer: A New Perspective on Pathogenesis Associated with Long Non-coding RNAs. Cancer Lett. 413, 94–101. doi:10.1016/j.canlet.2017.10.046
Jensen, T., Blanchette, A., Vadasz, S., Dave, A., Canfarotta, M., Sayej, W. N., et al. (2015). Biomimetic and Synthetic Esophageal Tissue Engineering. Biomaterials 57, 133–141. doi:10.1016/j.biomaterials.2015.04.004
Kuppan, P., Sethuraman, S., and Krishnan, U. M. (2016). Fabrication and Investigation of Nanofibrous Matrices as Esophageal Tissue Scaffolds Using Human Non-keratinized, Stratified, Squamous Epithelial Cells. RSC Adv. 6 (31), 26461–26473. doi:10.1039/c5ra24303c
Kuppan, P., Sethuraman, S., and Krishnan, U. M. (2017). In Vitro Co-culture of Epithelial Cells and Smooth Muscle Cells on Aligned Nanofibrous Scaffolds. Mater. Sci. Eng. C 81, 191–205. doi:10.1016/j.msec.2017.07.050
Levenson, G., Berger, A., Demma, J., Perrod, G., Domet, T., Arakelian, L., et al. (2022). Circumferential Esophageal Replacement by a Decellularized Esophageal Matrix in a Porcine Model. Surgery 171, 384–392. doi:10.1016/j.surg.2021.07.009
Luc, G., Charles, G., Gronnier, C., Cabau, M., Kalisky, C., Meulle, M., et al. (2018). Decellularized and Matured Esophageal Scaffold for Circumferential Esophagus Replacement: Proof of Concept in a Pig Model. Biomaterials 175, 1–18. doi:10.1016/j.biomaterials.2018.05.023
Lv, J., Chen, L., Zhu, Y., Hou, L., and Liu, Y. (2014). Promoting Epithelium Regeneration for Esophageal Tissue Engineering through Basement Membrane Reconstitution. ACS Appl. Mater. Inter. 6 (7), 4954–4964. doi:10.1021/am4059809
Marzaro, M., Algeri, M., Tomao, L., Tedesco, S., Caldaro, T., Balassone, V., et al. (2020). Successful Muscle Regeneration by a Homologous Microperforated Scaffold Seeded with Autologous Mesenchymal Stromal Cells in a Porcine Esophageal Substitution Model. Therap Adv. Gastroenterol. 13, 175628482092322. doi:10.1177/1756284820923220
Matai, I., Kaur, G., Seyedsalehi, A., McClinton, A., and Laurencin, C. T. (2020). Progress in 3D Bioprinting Technology for Tissue/organ Regenerative Engineering. Biomaterials 226, 119536. doi:10.1016/j.biomaterials.2019.119536
Memic, A., Navaei, A., Mirani, B., Cordova, J. A. V., Aldhahri, M., Dolatshahi-Pirouz, A., et al. (2017). Bioprinting Technologies for Disease Modeling. Biotechnol. Lett. 39 (9), 1279–1290. doi:10.1007/s10529-017-2360-z
Nieponice, A., Ciotola, F. F., Nachman, F., Jobe, B. A., Hoppo, T., Londono, R., et al. (2014). Patch Esophagoplasty: Esophageal Reconstruction Using Biologic Scaffolds. Ann. Thorac. Surg. 97 (1), 283–288. doi:10.1016/j.athoracsur.2013.08.011
Park, H., Kim, I. G., Wu, Y., Cho, H., Shin, J. W., Park, S. A., et al. (2021). Experimental Investigation of Esophageal Reconstruction with Electrospun Polyurethane Nanofiber and 3D Printing Polycaprolactone Scaffolds Using a Rat Model. Head & Neck 43 (3), 833–848. doi:10.1002/hed.26540
Peirlinck, M., Debusschere, N., Iannaccone, F., Siersema, P. D., Verhegghe, B., Segers, P., et al. (2018). An In Silico Biomechanical Analysis of the Stent-Esophagus Interaction. Biomech. Model. Mechanobiol 17 (1), 111–131. doi:10.1007/s10237-017-0948-9
Pennathur, A., Gibson, M. K., Jobe, B. A., and Luketich, J. D. (2013). Oesophageal Carcinoma. The Lancet 381 (9864), 400–412. doi:10.1016/s0140-6736(12)60643-6
Pisani, S., Croce, S., Chiesa, E., Dorati, R., Lenta, E., Genta, I., et al. (2020). Tissue Engineered Esophageal Patch by Mesenchymal Stromal Cells: Optimization of Electrospun Patch Engineering. Ijms 21 (5), 1764. doi:10.3390/ijms21051764
Rao, P., Sun, T. L., Chen, L., Takahashi, R., Shinohara, G., Guo, H., et al. (2018). Tough Hydrogels with Fast, Strong, and Reversible Underwater Adhesion Based on a Multiscale Design. Adv. Mater. 30 (32), 1801884. doi:10.1002/adma.201801884
Sharma, S., Kumar, R., Kumari, P., Kharwar, R. N., Yadav, A. K., and Saripella, S. (2019). Mechanically Magnified Chitosan-Based Hydrogel as Tissue Adhesive and Antimicrobial Candidate. Int. J. Biol. Macromolecules 125, 109–115. doi:10.1016/j.ijbiomac.2018.12.018
Soliman, S., Laurent, J., Kalenjian, L., Burnette, K., Hedberg, B., and La Francesca, S. (2019). A Multilayer Scaffold Design with Spatial Arrangement of Cells to Modulate Esophageal Tissue Growth. J. Biomed. Mater. Res. 107 (2), 324–331. doi:10.1002/jbm.b.34124
Syed, O., Kim, J.-H., Keskin-Erdogan, Z., Day, R. M., El-Fiqi, A., Kim, H.-W., et al. (2019). SIS/aligned Fibre Scaffold Designed to Meet Layered Oesophageal Tissue Complexity and Properties. Acta Biomater. 99, 181–195. doi:10.1016/j.actbio.2019.08.015
Tan, B., Wei, R.-Q., Tan, M.-Y., Luo, J.-C., Deng, L., Chen, X.-H., et al. (2013). Tissue Engineered Esophagus by Mesenchymal Stem Cell Seeding for Esophageal Repair in a Canine Model. J. Surg. Res. 182 (1), 40–48. doi:10.1016/j.jss.2012.07.054
Tan, H., Jin, D., Qu, X., Liu, H., Chen, X., Yin, M., et al. (2019). A PEG-Lysozyme Hydrogel Harvests Multiple Functions as a Fit-To-Shape Tissue Sealant for Internal-Use of Body. Biomaterials 192, 392–404. doi:10.1016/j.biomaterials.2018.10.047
Tan, Y. J., Yeong, W. Y., Tan, X., An, J., Chian, K. S., and Leong, K. F. (2016). Characterization, Mechanical Behavior and In Vitro Evaluation of a Melt-Drawn Scaffold for Esophageal Tissue Engineering. J. Mech. Behav. Biomed. Mater. 57, 246–259. doi:10.1016/j.jmbbm.2015.12.015
Uhlenhopp, D. J., Then, E. O., Sunkara, T., and Gaduputi, V. (2020). Epidemiology of Esophageal Cancer: Update in Global Trends, Etiology and Risk Factors. Clin. J. Gastroenterol. 13 (6), 1010–1021. doi:10.1007/s12328-020-01237-x
Urbani, L., Camilli, C., Phylactopoulos, D.-E., Crowley, C., Natarajan, D., Scottoni, F., et al. (2018). Multi-stage Bioengineering of a Layered Oesophagus with In Vitro Expanded Muscle and Epithelial Adult Progenitors. Nat. Commun. 9, 16. doi:10.1038/s41467-018-06385-w
Wan, Z., Zhang, P., Liu, Y., Lv, L., and Zhou, Y. (2020). Four-dimensional Bioprinting: Current Developments and Applications in Bone Tissue Engineering. Acta Biomater. 101, 26–42. doi:10.1016/j.actbio.2019.10.038
Wang, D., Xu, P., Wang, S., Li, W., and Liu, W. (2020a). Rapidly Curable Hyaluronic Acid-Catechol Hydrogels Inspired by Scallops as Tissue Adhesives for Hemostasis and Wound Healing. Eur. Polym. J. 134, 109763. doi:10.1016/j.eurpolymj.2020.109763
Wang, X., Jin, J., Hou, R., Zhou, M., Mou, X., Xu, K., et al. (2020b). Differentiation of bMSCs on Biocompatible, Biodegradable, and Biomimetic Scaffolds for Largely Defected Tissue Repair. ACS Appl. Bio Mater. 3 (1), 735–746. doi:10.1021/acsabm.9b01063
Wei, Q., Jin, J., Wang, X., Shen, Q., Zhou, M., Bu, S., et al. (2018). The Growth and Pluripotency of Mesenchymal Stem Cell on the Biodegradable Polyurethane Synthesized with Ferric Catalyst. J. Biomater. Sci. Polym. Edition 29 (10), 1095–1108. doi:10.1080/09205063.2018.1426424
Wu, Y., Kennedy, P., Bonazza, N., Yu, Y., Dhawan, A., and Ozbolat, I. (2021). Three-Dimensional Bioprinting of Articular Cartilage: A Systematic Review. Cartilage 12 (1), 76–92. doi:10.1177/1947603518809410
Yang, Y., Yang, G., Liu, X., Xu, Y., Zhao, S., Zhang, W., et al. (2021). Construction of Lung Tumor Model for Drug Screening Based on 3D Bio-Printing Technology. J Biomater. Tissue Eng. 11 (7), 1213–1226. doi:10.1166/jbt.2021.2706
Zhu, Y., Chan-Park, M. B., and Sin Chian, K. (2005). The Growth Improvement of Porcine Esophageal Smooth Muscle Cells on Collagen-Grafted Poly(DL-Lactide-Co-Glycolide) Membrane. J. Biomed. Mater. Res. 75B (1), 193–199. doi:10.1002/jbm.b.30305
Zhu, Y., Chian, K. S., Chan-Park, M. B., Mhaisalkar, P. S., and Ratner, B. D. (2006). Protein Bonding on Biodegradable Poly(L-Lactide-Co-Caprolactone) Membrane for Esophageal Tissue Engineering. Biomaterials 27 (1), 68–78. doi:10.1016/j.biomaterials.2005.05.069
Zhu, Y., Yang, K., Cheng, R., Xiang, Y., Yuan, T., Cheng, Y., et al. (2017). The Current Status of Biodegradable Stent to Treat Benign Luminal Disease. Mater. Today 20 (9), 516–529. doi:10.1016/j.mattod.2017.05.002
Keywords: esophageal repair, tissue engineering, single-layer scaffold, multi-layer scaffold, stem cells
Citation: Xu R, Fang X, Wu S, Wang Y, Zhong Y, Hou R, Zhang L, Shao L, Pang Q, Zhang J, Cui X, Zuo R, Yao L and Zhu Y (2022) Development and Prospect of Esophageal Tissue Engineering. Front. Bioeng. Biotechnol. 10:853193. doi: 10.3389/fbioe.2022.853193
Received: 12 January 2022; Accepted: 31 January 2022;
Published: 17 February 2022.
Edited by:
Guicai Li, Nantong University, ChinaReviewed by:
Yifeng Lei, Wuhan University, ChinaCopyright © 2022 Xu, Fang, Wu, Wang, Zhong, Hou, Zhang, Shao, Pang, Zhang, Cui, Zuo, Yao and Zhu. This is an open-access article distributed under the terms of the Creative Commons Attribution License (CC BY). The use, distribution or reproduction in other forums is permitted, provided the original author(s) and the copyright owner(s) are credited and that the original publication in this journal is cited, in accordance with accepted academic practice. No use, distribution or reproduction is permitted which does not comply with these terms.
*Correspondence: Ruixia Hou, aG91cngxMzBAMTYzLmNvbQ==
Disclaimer: All claims expressed in this article are solely those of the authors and do not necessarily represent those of their affiliated organizations, or those of the publisher, the editors and the reviewers. Any product that may be evaluated in this article or claim that may be made by its manufacturer is not guaranteed or endorsed by the publisher.
Research integrity at Frontiers
Learn more about the work of our research integrity team to safeguard the quality of each article we publish.