- 1Department of Bioprocess Engineering, University of Science and Technology (UST) of Korea, Daejeon, South Korea
- 2School of Chemical and Biological Engineering, Seoul National University, Seoul, South Korea
- 3Biotechnology Process Engineering Center, Korea Research Institute of Biosceince and Biotechnology (KRIBB), Daejeon, Chungcheongbuk-do, South Korea
- 4Department of Environmental Engineering, College of Engineering, Ajou University, Suwon, Gyeonggi-do, South Korea
- 5Department of Environmental and Safety Engineering, College of Engineering, Ajou University, Suwon, Gyeonggi-do, South Korea
Introduciton: The α,ω-diamines (NH2-(CH2)n-NH2) and ω -amino fatty acids (NH2-(CH2)n-COOH) have been widely used as building blocks in polymerindustries. Medium- to long-chain (C8 to C18) fatty acid monomers with amino residues are almost exclusively produced via chemical processes that generate hazardous waste and induce severe environmental problems, such as global warming and pollution. Here, we present the construction platformstrains of Yarrowia lipolytica a cheese-ripening yeast, for direct biotransformation of hydrocarbons into medium- to long-chain α,ω-diamines and ωamino fatty acids using metabolic engineering of endogenous fatty acid ω- and β-oxidation pathways and introducing heterologous ω-transaminase in Y. lipolytica.
Methods: We deleted six genes encoding the acyl-CoA oxidase (ACO1–6) and four fatty aldehyde dehydrogenase genes (FALDH1-4), which catalyze fatty acid β-oxidation and downstream oxidation of fatty aldehydes in Y. lipolytica, respectively. The ω-transaminase from Chromobacterium violaceum DSM30191 was introduced into the genome of the ΔPOX ΔFALDH strain under the control of Y. lipolytica-derived EXP1 promoters.
Results and Discussion: The ΔPOX ΔFALDH strains with ω-CvTA successfully accumulated the corresponding C12 αω-diamines into a shaking culture medium with dodecane or dodecanol. In addition, these strains accumulated C12 ω-amino fatty acids from dodecanoic acid. With the commercially available α,ω-diacid bioprocess, this yeast biosynthesis producing medium- and longchain α,ω-diamines and ω-amino fatty acids could complete the yeast platform technology generating all medium- and long-chain aliphatic polyamide monomers, α,ω-biofunctionalized with one or both carboxylic acid and amino residues.
1 Introduction
Plastics are materials that possess a high molecular weight and are composed of repeating organic molecules (monomers). Plastics play essential and ubiquitous roles in modern life. Synthetic plastics are commonly produced via polymerization of petrol-based chemicals; this process consumes approximately 8% of the annual petroleum production (Carpenter 2020).
Polyester (PE) and polyamide (PA) plastics are the two most important classes of commercial plastics, and the monomers of each plastic are joined by an ester (-O-CO-) and an amide (-CO-NH-) linkage, respectively. Both plastics can be synthesized as homopolymers (e.g., PA12 laurolactam, polylactic acid from lactic acid) or copolymers (e.g., PA 12.12 from 1,12-diaminododecane, dodecanedioic acid or polybutylene succinate from succinate and 1,4-butanediol). For PA, the monomers are α,ω-fatty dicarboxylic acids (α,ω-diacids) and α,ω-fatty diamines (α,ω-diamine) or ω-amino fatty acids (ω-AFA), while for PE, the monomers are α,ω-diacids and α,ω-fatty dialcohols (α,ω-diol) or ω-hydroxy fatty acids (ω-HFAs) (Schaffer and Hass 2014). Compared to the short-chain (C2–C6) plastics, medium-chain (C8–C14) repeating monomers exhibit high-performance properties, including flexibility, chemical resistance, low moisture absorption, and resistance to stress cracking (Supplementary Figure S1A) (Albanese et al., 2016). At present, C12 monomers for aliphatic PA and PE are almost exclusively produced via chemical processes, which have several disadvantages, including limitations in the range of products, use of multi-step conversion processes (Supplementary Figure S1B), dependence on non-renewable feedstocks, and generation of hazardous waste and emissions, which induce severe environmental problems, such as global warming and pollution.
Biotechnology offers innovative approaches to overcome the disadvantages involved in the chemical processing of medium-chain polymeric monomers. Yeast biocatalysts can oxidize the terminal (ω) carbons of medium- to long-chain n-alkanes, fatty alcohols, and fatty acids via the ω-oxidation pathway (Craft et al., 2003; Van Bogaert et al., 2011), thereby converting the compounds to their corresponding α,ω-diacids or ω-hydroxy fatty acids (ω-HFAs). The yeasts Candida tropicalis and Y. lipolytica have been used as industrial biocatalysts to produce lipophilic products. Y. lipolytica is classified and genetically recognized as safe (GRAS) and can utilize the ω-oxidation pathway to oxidize hydrophobic substrates, generating corresponding α,ω-bifunctional metabolites (α,ω-diacids, α,ω-diol, and ω-hydroxy fatty acids) that can be used as polymer building blocks (Figure 2A). For plastic monomers with amino residues such as α,ω-diamines and ω-AFA, ω-oxidation reactions are connected to ω-transamination. Several studies have been performed to produce ω-AFAs from fatty acids using Escherichia coli expressing multi-enzyme cascade pathways, including heterologous monooxygenases and ω-transaminase (Ladkau et al., 2016; Ahsan et al., 2018; Ge et al., 2020). Sung et al. (2018) produced ω-AFAs from hydroxy fatty acids or α,ω-diamines from α,ω-diols using Escherichia coli containing heterologous alcohol dehydrogenase and ω-transaminase. Recently, Citoler et al. (2019) reported the biocatalytic amination of fatty acids through a one-pot tandem cascade catalyzed by a carboxylic acid reductase (CAR) and a transaminase (ω-TA). However, the use of yeast in synthesizing medium- to long-chain α,ω-diamines or ω-AFAs from low-cost raw materials has not yet reached its proof-of-concept stage.
Here, we present the construction of platform strains of Y. lipolytica, a cheese-ripening yeast, for direct biotransformation of hydrocarbons into medium- to long-chain α,ω-fatty diamines [NH2-(CH2)n-NH2] and ω-amino fatty acids [NH2-(CH2)n-COOH], which are building blocks for high-performance polymers (Carpenter 2020), via metabolic engineering of endogenous fatty acid ω- and β-oxidation pathways and introducing heterologous ω-transaminase (ω-TA).
2 Materials and methods
2.1 Yeast strains and plasmids
Y. lipolytica strains, plasmids, and oligonucleotides used in this study are listed in Supplementary Table S1. Genetic engineering was performed according to the standard procedures. All oligonucleotides were synthesized by Cosmogenetech Co., Ltd., (Daejeon, South Korea). Plasmids and DNA fragments were prepared using Qiagen kits (Germany), and other chemicals were purchased from Sigma-Aldrich (United States).
2.2 Genome engineering of Y. lipolytica
Genome modification of Y. lipolytica was based on the homologous recombination and 5-fluoroorotic acid (5-FOA) selection using URA3 as a recyclable selection marker (Figure 1). URA3 was amplified from Y. lipolytica, and pop-out regions were used with glutamate production gene or histidine-related gene from the pGEM T easy cloning vector (Table 1) using the PCR using primers listed in Supplementary Table S2. To construct the gene disruption cassettes, the 5′- and 3′-flanking regions of each gene listed in Supplementary Table S2 were amplified from the genomic DNA of Y. lipolytica through PCR using the XXX_F1/XXX_R1 and XXX_F2/XXX_R2 primers (Supplementary Table S1). These homologous regions for the deletion and pop-out cassettes were obtained using overlap PCR, and the bipartite fragments were transformed into yeast cells by using a one-step transformation method. The 5-FOA selection and uracil marker rescue were performed. The successful disruption of a gene was verified using PCR using the primers XXX_F1/XXX_R1 and XXX_F2/XXX_R2. To introduce ω-transamination into Y. lipolytica, ω-transaminase (ω-TA) from C. violaceum DSM30191 was expressed under the control of Y. lipolytica-derived EXP1 promoters (Blazeck et al., 2011) with or without signal sequences from the ALK1 at the N-terminus for ER membrane anchoring (Iida et al., 1998) (atgtccaacgccctcaacctgtcgctggcgctcggcgtctttctgctagcctactatggcttctccgtgatccagtaccgcatcaaaa cccgcaagctcgaaaagaagtggaagtgtggtaagcccaaggatatttcacgattc). Each ω-TA cassette was integrated into the FALDH4 locus of the engineered Y. lipolytica strain. Genomic DNA from the transformants was extracted using the Gene All DNA extraction kit (Gene all biotechnology, Korea). A Biometra T3000 thermocycler with Prime Star DNA polymerase (Takara, Japan) was used for PCR amplification. Amplified fragments were purified using the QIAgen purification kit (Qiagen, Germany), and DNA fragments were recovered from the agarose gels using the QIAquick gel extraction kit (Qiagen, Germany).
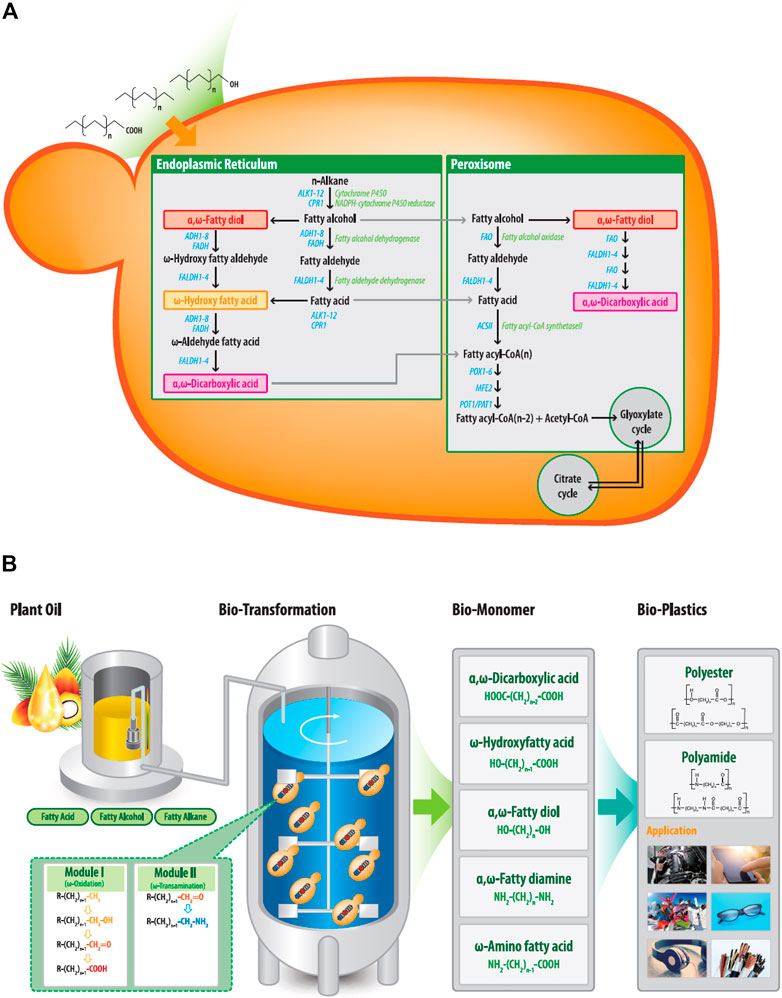
FIGURE 1. (A) Metabolic pathways for hydrophobic substrates, including fatty alkanes, fatty alcohol, and fatty acids, in Y. lipolytica. The substrates were removed in the cell and then converted to their corresponding fatty acids or α,ω-diacids via the ω-oxidation pathway. Fatty acids and α,ω-diacids are then degraded to acetyl-CoA during β-oxidation pathway. (B) Biotechnological synthesis of α,ω-bifunctional polymeric monomers from plant oil-based derivatives. Fatty alkanes, fatty alcohol, and fatty acids are used as feedstock and are converted to corresponding α,ω-bifunctional chemicals through module 1 and/or 2. These final products can be used as building blocks of polyester and polyamide (R: H3C-, HO-, O=, HOOC-).
2.3 Biotransformation condition
A single colony of the engineered Y. lipolytica strains was pre-grown in a test tube containing 3 ml yeast extract Bacto-pepton dDextrose (YPD) medium overnight at 30°C with shaking at 200 rpm. One milliliter of pre-cultured broth was inoculated into a 500 ml baffled flask containing 100 ml of growth medium (pH 6.0) containing 50 g/L glucose, 10 g/L yeast extract, 6.7 g/L yeast nitrogen base without amino acids, 5 g/L (NH4)2SO4, and .05 g/L uracil. The cultures were incubated overnight at 30°C with rotation at 200 rpm. When an OD600 of 40–50 was reached, 50 ml of the culture broth was transferred to 250 ml baffled flasks containing 50 ml of the conversion medium (pH 7.6) containing 30 g/L glucose, 3 g/L yeast extract, 6.7 g/L YNB w/o amino acids, 5 g/L (NH4)2SO4, .05 g/L uracil, and 1 g/L substrate (fatty alkane, fatty alcohol, or fatty acid) with .05% Tween 80. This biotransformation was performed for 2 days at 30°C in a shaking incubator (200 rpm). To investigate the effect of multiple gene knock-out on cell growth, Y. lipolytica was incubated in a yeast extract-peptone medium (YPD) containing 10 g/L yeast extract and 20 g/L Bacto-peptone in sterile 24-well PS plates (Falcon, Corning, NY). Each well contained 400 µl of YP media with 2 µl of the pre-cultured Y. lipolytica. The Y. lipolytica culturing was done in 20 ml of YPD medium in 250 ml baffled flask incubated overnight at 30°C. The plate was incubated on a shaking plate reader (Tecan, Männedorf, Switzerland) at 30°C at 200 RPM, and the turbidity of each well was measured every 15 min (no sampling of the culture).
2.4 Analytical methods
The biomass was measured using a UV spectrophotometer (Uvikon XL, Secomam, France) at 600 nm absorbance. To analyze the subcellular localization of ω-CvTAER and ω-CvTAcytosol introduced into Y. lipolytica, cells were prepared, and fluorescence microscopy observations were performed. PDI monoclonal antibody (MA1-10032) was used as the primary antibody for ω-CvTA (Abfrontier company, Seoul, Republic of Korea) for the ER marker, and the goat anti-mouse IgG1 (H&L; Alexa Fluor® 488) was used as the secondary antibody. Substrates and products were identified and quantified using gas chromatography-mass spectrometry (GC-MS) (Agilent GC/MSD 5975 coupled with an Agilent 7890A) equipped with a quadrupole mass selective detector on electroionization (EI) operated at 70 eV. An Agilent HP-5MS column (30 m length, .25 mm inner diameter, .25 μm film thickness) was used at a 10:1 split ratio, helium was used as a carrier gas, and the oven temperature was 150°C–172°C. The substrates and products were extracted and prepared for GC-MS analysis according to the corresponding methods shown in Supplementary Figure S2. Briefly, extraction was performed from acid-or base-treated culture broth using an equivalent volume of diethyl ether or chloroform. Methanol dissolved in 2.2% hydrochloric acid and 10% toluene was added to the reacted samples (1:1, v/v) and the mixture was incubated for 1 h at 100°C. After heating, the mixture was acidified with H2SO4 to convert the ionized ω-amino fatty acid to ω-amino fatty acid and directly extracted with diethyl ether. For sialylation, N,O-bis(trimethylsilyl)trifluoroacetamide was mixed with the extracted samples (1:1, v/v) and the mixture was incubated for 20 min at 60°C. Tetradecane was used as an internal standard.
3 Results
3.1 Genome engineering of Y. lipolytica
Multiple genes are involved in each step of ω- and β-oxidation (Supplementary Table S2). Therefore, genome-wide modifications are required to engineer a strain of Y. lipolytica suitable to produce α,ω-bifunctional monomers with amino residues, aliphatic polymeric monomers with amino residues, and hydrophobic substrates. In Y. lipolytica, non-homologous end-joining (NHEJ) is used more than homologous recombination (HR) for the repair of double-strand breaks in DNA (Krtezschmar et al., 2013; Verbeke et al., 2013). To improve HR efficiency, we used the strains with the disrupted KU70 responsible for NHEJ-based double-strand break repair, and then we performed a bipartite PCR-based gene-targeting method using URA3 as a recyclable selection marker (Figure 2). The bipartite deletion cassettes used to remove target genes (Supplementary Table S1) were specifically inserted into sites in the genome via URA3 split-marker recombination. The sites of integration were confirmed by PCR, which was followed by pop-out of URA3 via selection of the 5′-FOA plate. This strategy allowed for the deletion of several genes and the construction of various strains possessing ω-/β-oxidation pathways (Table 1).
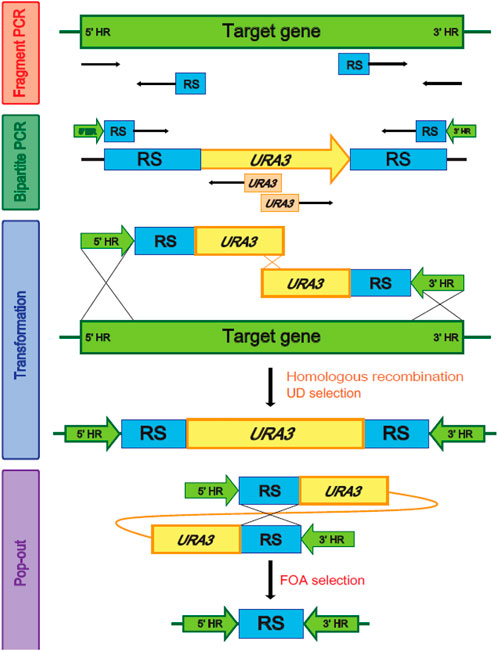
FIGURE 2. Homologous recombination and the 5-FOA (5-Fluoroorotic acid) selection using URA3 as a recyclable selection marker. HR, Homologous region, RS, Repeated sequence.
3.2 Y. lipolytica platform strains for α,ω-bifunctional monomers with amino residue
To biosynthesize α,ω-bifunctional monomers with amino residues in Y. lipolytica, ω-oxidation was combined with ω-transamination reactions. Thus, we intended to oxidize the terminal end of the hydrophobic substrate into an aldehyde group by ω-oxidation and convert the aldehyde group into an amino group by ω-transaminase (ω-TA), which catalyzes the amination between the ω-positioned ketone and amine groups. However, as shown in Figure 1A, ω-oxidation pathway in Y. lipolytica oxidizes hydrocarbons substrates such as alkanes, fatty alcohols and acids to their corresponding terminal carboxy function (Craft et al., 2003). Fatty acid synthesized by the ω-oxidation pathway or introduced as a substrate can be further oxidized through the same ω-oxidation pathway to the corresponding α,ω-diacids. Finally, both fatty acids and α,ω-diacids are metabolized through the β-oxidation pathway to yield energy, CO2, and water (Poirier et al., 2006).
To block β-oxidation, we consecutively deleted six genes (POX1-6) encoding the acyl-CoA oxidase. The ΔPOX strain did not grow on fatty alkanes, fatty alcohols or acids as sole carbon sources (Supplementary Figure S3). To block the oxidizing aldehyde group into the carboxylic group in ω-oxidation, we eliminated four fatty aldehyde dehydrogenase genes (FALDH1-4) and inserted ω-CvTA (Klatte and Wendisch 2015) from Chromobacterium violaceum DSM30191 into the genome of the ΔPOX ΔFALDH strain (Figure 3A). The ω-CvTA was placed under the control of Y. lipolytica-derived EXP1 promoters (Blazeck et al., 2011), and its expression in two forms was designed with or without the ER targeting leader sequence (ERTLS) (Iida et al., 1998) from ALK1 at its N-terminus. Confocal microscopy confirmed that two forms of ω-CvTA were successfully expressed in Y. lipolytica; and one form (ω-CvTA with ERTLS, ω-CvTAER) was exclusively localized to the ER and the other (ω-TA without ERTLS, ω-CvTAcytosol) to the cytosol (Figure 3B).
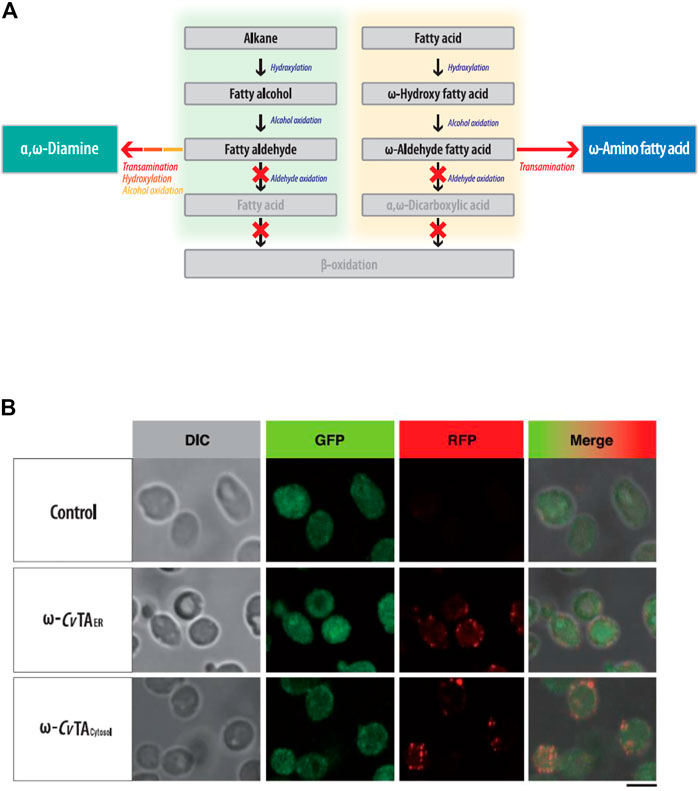
FIGURE 3. (A) Metabolic engineering of Y. lipolytica for the biotransformation of hydrocarbons (fatty alkane, alcohol, and acids) into the fatty polymeric monomers with amino residue. (B) Subcellular localization of ω-CvTAER and ω-CvTAcytosol introduced into Y. lipolytica. Bar is 5 μm-size.
3.3 The effect of multiple gene knock-out on cell growth
The effect of multiple gene knock-out on cell growth was investigated by monitoring the growth of each mutant using a plate reader. Time-profiles of the monitored cell growth (Figure 4) were fitted to the Gompertz model (Zwietering et al., 1990) by least squares regression, which could determine the maximum specific growth rate (μmax) and lag time (λ) of each mutant. Compared to Pol1g strain, all mutant showed the lower μmax, representing a theoretical maximum growth rate based on the inflection point of the curve, and more increased λ, the adaptation time. However, a remarkable toxic effect on all mutants was not observed.
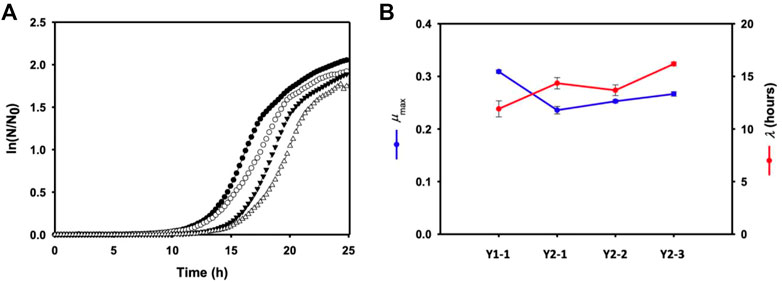
FIGURE 4. (A) Cell growth of each mutant (closed circular: Y1-1, open circular: Y2-1, closed inverted triangle: Y1-1, and open inverted trianlge: Y2-3) in a yeast extract-peptone medium containing 10 g/L yeast extract and 20 g/L Bacto-peptone in sterile 24-well PS plates. (B) the maximum specific growth rate (μmax) and lag time (λ) of each mutant calculated by the Gompertz model. Error bars represent the standard error of the mean of at least three independent replicates.
3.4 Production of α,ω-bifunctional monomers with amino residue in Y. lipolytica
The ΔPOXΔFALDH strains with ω-CvTAER or ω-CvTAcytosol successfully accumulated the corresponding 1,12-diaminododecanes into the culture medium in a shaking flask culture with 1 g/L of dodecane or dodecanol as C12 hydrocarbon substrates (Figures 5A, B, Supplementary Figure S4), but the strain with ω-CvTAER produced more than 2-fold of 1,12-diaminododecanes (8.32 ± .70 mg/L, from dodecane as a substrate and 5.81 ± .40 mg/L from dodecanol) than those with ω-CvTAcytosol (3.62 ± .46 mg/L and 2.43 ± .49 mg/L from dodecane and dodecanol, respectively). To examine whether the strains could convert FAs to the corresponding ω-AFAs, these strains were cultivated in a shaking flask with 1 g/L of dodecanoic acid. These strains successfully accumulated 12-aminododecanoic acids in the culture medium (Figure 5C, Supplementary Figure S5). Similar to 1,12-diaminododecanes, the strain with ω-CvTAER also produced more than 2-folds of 12-aminododecanoic acids (7.94 ± .54 mg/L) than those with ω-CvTAcytosol (1.91 ± .65 mg/L). Surprisingly, 12-aminododecanoic acids were produced from dodecane (1.91 ± .18 mg/L for ω-CvTAER and .77 ± .28 mg/L for ω-CvTAcytosol) and dodecanol (1.11 ± .58 mg/L for ω-CvTAER and .48 ± .16 mg/L for ω-CvTAcytosol) (Figures 4A, B), which may be resulted from the overoxidation of some cytochrome P450s as described previously (Lu et al., 2010). These strains also produced 1,12-dodecanedioic acids and 12-hydroxydodecanoic acids as by-products, and their amounts were similar to those in the ΔPOX strains.
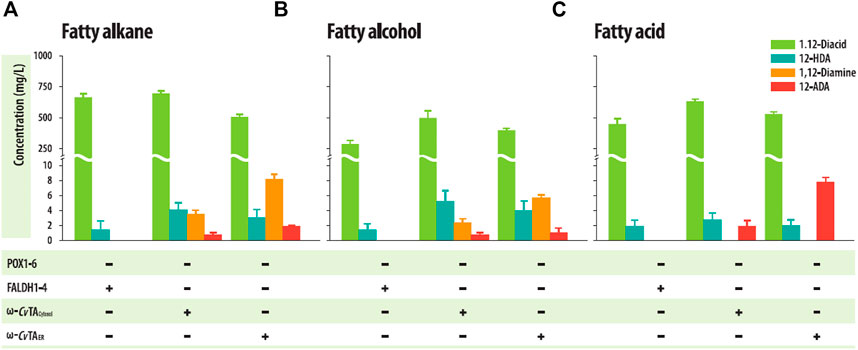
FIGURE 5. Production of α,ω-diaminododecane and ω-amino dodecanoic acid from (A) dodecane, (B) dodecanol, and (C) dodecanoic acid by engineered Y. lipolytica strains in shake flask cultures. Error bars represent the standard error of the mean of at least three independent replicates.
When substrates were expanded to an odd number (C11) or long chain (C16), both ΔPOX and ΔFALDH strains with ω-CvTAER or ω-CvTAcytosol also produced the corresponding ω-AFAs (1.91 ± .18 mgC11 ω-AFAs/L and .77 ± .28 mgC16 ω-AFAs/L for ω-CvTAER and 1.91 ± .18 mgC11 ω-AFAs/L and .77 ± .28 mgC16 ω-AFAs/L for ω-CvTAcytosol) (Figure 6A). To increase the production of αω-FDs and ω-AFAs with amino residues, we engineered Y. lipolytica strain by knocking out fatty alcohol oxidase and increasing the copy numbers of ω-CvTA. Interestingly, only FAO1-deleted strains produced 1,12-diols, indicating that FAO1 plays an important role in the degradation of alcohol groups, which increased the production of α,ω-diamines and ω-amino fatty acids (Figure 6B). These findings were similar with previous studies (Gatter et al., 2014; Iwama et al., 2015).
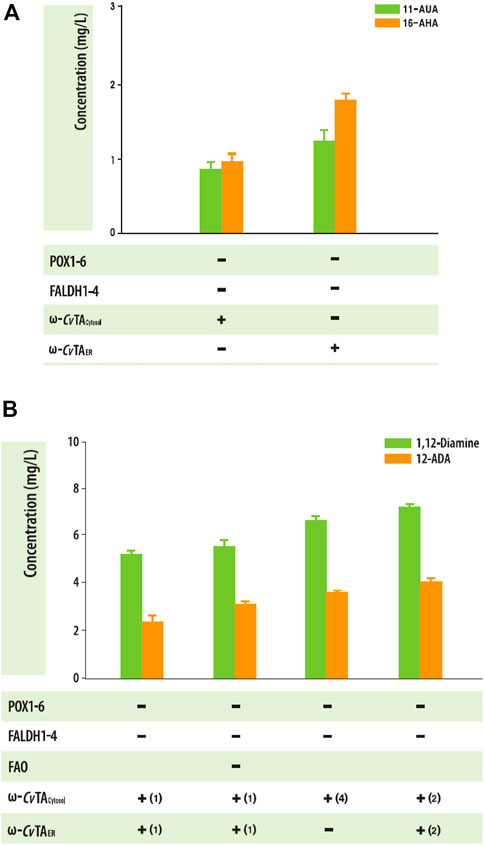
FIGURE 6. (A) Biotransformation of C11 and C16 fatty acids into the corresponding ω-amino fatty acids in the engineered Y. lipolytica strains. (B) Effect of ΔFAO1 and copy numbers of ω-TA on the production of amine-compound production in engineered Y. lipolytica. Error bars represent the standard error of the mean of at least three independent replicates.
4 Discussion
Biotransformation of hydrocarbons into polymeric monomers with amino residues has mainly been attempted using whole-cell systems of E. coli by introducing heterologous CYP genes. However, attempts encountered some problems, such as CYP expression and hydrophobic substrate uptake in E. coli-based whole-cell biotransformation. In particular, the correct expression of CYP in E. coli requires a heme precursor such as expensive δ-ala-leuvenic acid (Wu et al., 2006; Ichinose and Wariishi 2013). The n-alkane-assimilating yeasts with an endogenous CYP belonging to the CYP52 family and a reductase provide an advantageous approach for ω-oxidation of hydrocarbons. The diploid yeast C. tropicalis produces 174 g/L C14 diacid from methyl ester C14 fatty acid and 153 g/L of C13 α,ω-diacids from tridecane (Picataggio et al., 1992). Cathay Biotechnologies Inc. (China) used C. tropicalis to industrially produce C11–C16 α and ω-diacids from petroleum-based n-paraffin. Lu et al. (2010) reported high-level production of ω-hydroxy fatty acids using C. tropicalis through a modification of the α- and β-oxidation pathways.
In this study, we needed genome-wide modification and thus selected an alternative n-alkane-assimilating haploid Y. lipolytica as a starting strain since C. tropicalis is an obligate diploid (Seevai et al., 2013). We successfully generated α,ω-diamines and ω-amino fatty acids from hydrocarbons by oxidizing the terminal of the hydrophobic substrate into an aldehyde group via ω-oxidation and converting an aldehyde group into an amino group by ω-transaminase, which catalyzes the amination between the ω-positioned ketone and amine group. To the best of our knowledge, this study is the first to establish a Y. lipolytica-based biocatalytic system for the production of α,ω-diamines or ω-amino fatty acids from n-alkanes, fatty alcohols and acids. Although whole-cell catalytic systems using E. coli has been recently developed (Yoo et al., 2022), the systems have the critical disadvantages including CYP expression and hydrophobic substrate uptakes. Firstly, CYP expression in E. coli requires a heme precursor, which supplements culture medium with δ-ala-leuvenic acid, an expensive commodity (Wu et al., 2006; Cheesman et al., 2013; Ichinose and Wariishi, 2013; Ichinose et al., 2015). Furthermore, E. coli shows limited uptake of hydrophobic substrates, which can lower the productivity.
In conclusion, with the commercially available α,ω-diacid bioprocess, this yeast biosynthesis that produces medium- and long-chain α,ω-diamines and ω-amino fatty acids could complete the yeast platform technology generating all medium- and long-chain aliphatic polyamide monomers, α,ω-biofunctionalized with one or both of carboxylic acid or amino residues (Figure 7). Therefore, although the use of plant oils has been focused on manufacturing biodiesel, this study will contribute in advancing the inexpensive plant oil-derived fatty acids or alcohols as substrates while producing high-value products [for example, from dodencanoic acid or dodecanol (US$ 1–1.5/kg) to 1,12-dodecanedioc acid, 1,12-dodecanediamine, and 1,12-dodecanediol (above US$ 7/kg)]. In this study, however, the low production of α,ω-diamines and ω-amino fatty acids was limited due to over-oxidized by-products (1,12-diacids and 12-hydroxy fatty acids). In our future studies, we will conduct the engineering of the CYP protein to reduce over-oxidation activity and develop fermentation and purification processes for α,ω-diamines and ω-amino fatty acids.
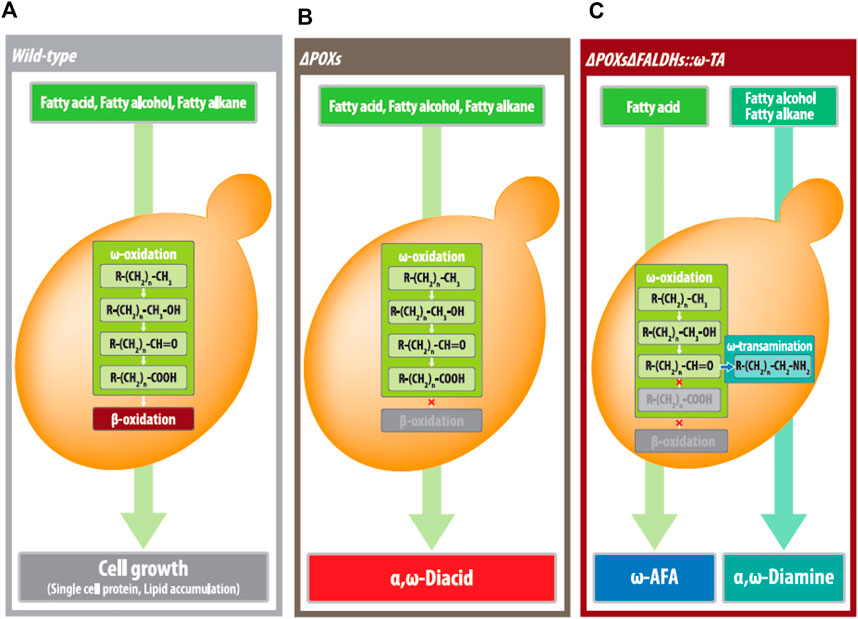
FIGURE 7. Yeast biocatalytic platforms for biotransfomation of MCLC hydrophobic substrates to industrial applicable products including (A) single cell protein or lipid by wild-type strain, (B) α,ω-diacids (fatty alkanes, fatty alcohols, and fatty acids as substrates), by the ΔPOXs strains, (C) ω-amino fatty acids (for fatty acids as substrates), and α,ω-diamines (for fatty alkane and fatty alcohol as substrates) by the ΔPOXs ΔFALDHs strains expressing ω-TA.
Data availability statement
The original contributions presented in the study are included in the article/Supplementary Material, further inquiries can be directed to the corresponding authors.
Author contributions
H-WL, B-GK, K-YC, and JA designed the study. GP, YK, MJ, HP, and WJ conducted bioconversion experiments and analyzed the products. K-YC and JA wrote and revised the manuscript.
Funding
This work was supported by the Industrial Strategic Technology Development Program (Grant Nos. 2002734 and 20012501) funded by the Ministry of Trade, Industry and Energy (MOTIE, South Korea) and was also supported by the Basic Core Technology Development Program (Grant No. 2022M3J4A1091445) of the National Research Foundation (NRF) funded by the Ministry of Science and ICT (MSIT, South Korea).
Conflict of interest
The authors declare that the research was conducted in the absence of any commercial or financial relationships that could be construed as a potential conflict of interest.
Publisher’s note
All claims expressed in this article are solely those of the authors and do not necessarily represent those of their affiliated organizations, or those of the publisher, the editors and the reviewers. Any product that may be evaluated in this article, or claim that may be made by its manufacturer, is not guaranteed or endorsed by the publisher.
Supplementary material
The Supplementary Material for this article can be found online at: https://www.frontiersin.org/articles/10.3389/fbioe.2022.825576/full#supplementary-material
References
Ahsan, M. M., Jeon, H., Nadarajan, S. P., Chung, T., Yoo, H-W., Kim, B-G., et al. (2018). Biosynthesis of the nylon 12 monomer, ω-aminododecanoic acid with novel CYP153A, AlkJ, and ω-TA enzymes. Biotechnol. J. 13, e1700562. doi:10.1002/biot.201700562
Albanese, M., Boyenval, J., Marchese, P., Sullalti, S., and Celli, A. (2016). The aliphatic counterpart of PET, PPT and PBT aromatic polyesters: Effect of the molecular structure on thermo-mechanical properties. AIMS Mol. Sci. 3, 32–51. doi:10.3934/molsci.2016.1.32
Blazeck, J., Liu, L., Redden, H., and Apler, H. (2011). Tuning gene expression in Yarrowia lipolytica by a hybrid promoter approach. Appl. Environ. Microbiol. 77, 7905–7914. doi:10.1128/AEM.05763-11
Carpenter, S. (2020). Why the oil industry’s $400 billion bet on plastics could backfire. New Jersey, US: Forbes.
Cheesman, M. J., Traylor, M. J., Hilton, M. E., Richards, K. E., Taylor, M. C., Daborn, P. J., et al. (2013). Soluble and membrane-bound Drosophila melanogaster CYP6G1 expressed in Escherichia coli: Purification, activity, and binding properties toward multiple pesticides. Insect biochem. Mol. Biol. 43, 455–465. doi:10.1016/j.ibmb.2013.02.003
Citoler, J., Derrington, S. R., Galman, J. L., Bevinakatti, H., and Turner, N. J. (2019). A biocatalytic cascade for the conversion of fatty acids to fatty amines. Green Chem. 21, 4932–4935. doi:10.1039/c9gc02260k
Craft, D. L., Madduri, K. M., Eshoo, M., and Ron Wilson, C. (2003). Identification and characterization of the CYP52 family of Candida tropicalis ATCC 20336, important for the conversion of fatty acids and alkanes to α, ω-dicarboxylic acids. Appl. Environ. Microbiol. 69, 5983–5991. doi:10.1128/AEM.69.10.5983-5991.2003
Gatter, M., Förster, A., Bär, K., Winter, M., Otto, C., Petzsch, P., et al. (2014). A newly identified fatty alcohol oxidase gene is mainly responsible for the oxidation of long-chain ω-hydroxy fatty acids in Yarrowia lipolytica. FEMS Yeast Res. 14, 858–872. doi:10.1111/1567-1364.12176
Ge, J., Yang, X., Yu, H., and Ye, L. (2020). High-yield whole cell biosynthesis of Nylon 12 monomer with self-sufficient supply of multiple cofactors. Metab. Eng. 62, 172–185. doi:10.1016/j.ymben.2020.09.006
Ichinose, H., Hatakeyama, M., and Yamauchi, Y. (2015). Sequence modifications and heterologous expression of eukaryotic cytochromes P450 in Escherichia coli. J. Biosci. Bioeng. 120, 268–274. doi:10.1016/j.jbiosc.2015.01.019
Ichinose, H., and Wariishi, H. (2013). High-level heterologous expression of fungal cytochrome P450s in Escherichia coli. Biochem. Biophys. Res. Commun. 438, 289–294. doi:10.1016/j.bbrc.2013.07.057
Iida, T., Ohta, A., and Takagi, M. (1998). Cloning and characterization of an n-alkane-inducible cytochrome P450 gene essential for n-decane assimilation by Yarrowia lipolytica. Yeast 14, 1387–1397. doi:10.1002/(sici)1097-0061(199811)14:15<1387::aid-yea333>3.0.co;2-m
Iwama, R., Koboyashi, S., Ohta, A., Horiuchi, H., and Fukuda, R. (2015). Alcohol dehydrogenases and an alcohol oxidase involved in the assimilation of exogenous fatty alcohols in Yarrowia lipolytica. FEMS Yeast Res. 15, fov014. doi:10.1093/femsyr/fov014
Klatte, S., and Wendisch, V. F. (2015). Role of L-alanine for redox self-sufficient amination of alcohols. Microb. Cell Fact. 14, 9. doi:10.1186/s12934-014-0189-x
Kretzschmar, A., Otto, C., Holz, M., Werner, S., Hübner, L., and Barth, G. (2013). Increased homologous integration frequency in Yarrowia lipolytica strains defective in non-homologous end-joining. Curr. Genet. 59, 63–72. doi:10.1007/s00294-013-0389-7
Ladkau, N., Assmann, M., Schrewe, M., Julsing, M. K., Schmid, A., and Bühler, B. (2016). Efficient production of the Nylon 12 monomer ω-aminododecanoic acid methyl ester from renewable dodecanoic acid methyl ester with engineered Escherichia coli. Metab. Eng. 36, 1–9. doi:10.1016/j.ymben.2016.02.011
Lu, W., Ness, J. E., Xie, W., Zhang, X., Minshull, J., and Gross, R. A. (2010). Biosynthesis of monomers for plastics from renewable oils. J. Am. Chem. Soc. 132, 15451–15455. doi:10.1021/ja107707v
Picataggio, S., Rohrer, T., Deanda, K., Lanning, D., Reynolds, R., Mielenz, J., et al. (1992). Metabolic engineering of Candida tropicalis for the production of long-chain dicarboxylic acids. Biotechnol. (N Y). 10, 894–898. doi:10.1038/nbt0892-894
Poirier, Y., Antonenkov, V. D., Glumoff, T., and Kalervo Hiltunen, J. (2006). Peroxisomal beta-oxidation--a metabolic pathway with multiple functions. Biochim. Biophys. Acta. 1763, 1413–1426. doi:10.1016/j.bbamcr.2006.08.034
Schaffer, S., and Hass, T. (2014). Biocatalytic and fermentative production of α, ω-bifunctional polymer precursors. Org. Process Res. Dev. 18, 752–766. doi:10.1021/op5000418
Seervai, R. N. H., Jones, S. K., Hirakawa, M. P., Porman, A. M., and Bennett, R. J. (2013). Parasexuality and ploidy change in Candida tropicalis. Eukaryot. Cell 12 (12), 1629–1640. doi:10.1128/EC.00128-13
Sung, S., Jeon, H., Sarak, S., Ahsan, M. M., Patil, M. D., Kroutil, W., et al. (2018). Parallel anti-sense two-step cascade for alcohol amination leading to ω-amino fatty acids and α, ω-diamines. Green Chem. 20, 4591–4595. doi:10.1039/C8GC02122H
Van Bogaert, I. N., Groeneboer, S., Saerens, K., and Soetaert, W. (2011). The role of cytochrome P450 monooxygenases in microbial fatty acid metabolism. FEBS J. 278, 206–221. doi:10.1111/j.1742-4658.2010.07949.x
Verbeke, J., Beopoulos, A., and Nicaud, J-M. (2013). Efficient homologous recombination with short length flanking fragments in Ku70 deficient Yarrowia lipolytica strains. Biotechnol. Lett. 35, 571–576. doi:10.1007/s10529-012-1107-0
Wu, Z-L., Sohl, C. D., Shimada, T., and Guengerich, F. P. (2006). Recombinant enzymes overexpressed in bacteria show broad catalytic specificity of human cytochrome P450 2W1 and limited activity of human cytochrome P450 2S1. Mol. Pharmacol. 69, 2007–2014. doi:10.1124/mol.106.023648
Yoo, H. W., Jung, H., Sarak, S., Kim, Y. C., Park, B. G., Kim, B. G., et al. (2022). Multi-enzymatic cascade reactions with Escherichia coli-based modules for synthesizing various bioplastic monomers from fatty acid methyl esters. Green Chem. 24, 2222–2231. doi:10.1039/d1gc04532f
Keywords: α,ω-diamine, ω-amino fatty acids, ω-oxidation, ω-transamination, Yarrowia lipolytica, metabolic engineering
Citation: Park G, Kim YC, Jang M, Park H, Lee H-W, Jeon W, Kim B-G, Choi K-Y and Ahn J (2023) Biosynthesis of aliphatic plastic monomers with amino residues in Yarrowia lipolytica. Front. Bioeng. Biotechnol. 10:825576. doi: 10.3389/fbioe.2022.825576
Received: 30 November 2021; Accepted: 15 December 2022;
Published: 11 January 2023.
Edited by:
Wenming Zhang, Nanjing Tech University, ChinaReviewed by:
Zongbao K. Zhao, Dalian Institute of Chemical Physics (CAS), ChinaChen-Guang Liu, Shanghai Jiao Tong University, China
Copyright © 2023 Park, Kim, Jang, Park, Lee, Jeon, Kim, Choi and Ahn. This is an open-access article distributed under the terms of the Creative Commons Attribution License (CC BY). The use, distribution or reproduction in other forums is permitted, provided the original author(s) and the copyright owner(s) are credited and that the original publication in this journal is cited, in accordance with accepted academic practice. No use, distribution or reproduction is permitted which does not comply with these terms.
*Correspondence: Kwon-Young Choi, a3ljaG9pQGFqb3UuYWMua3I=; Jungoh Ahn, YWhuam9Aa3JpYmIub3Iua3I=
†These authors have contributed equally to this study