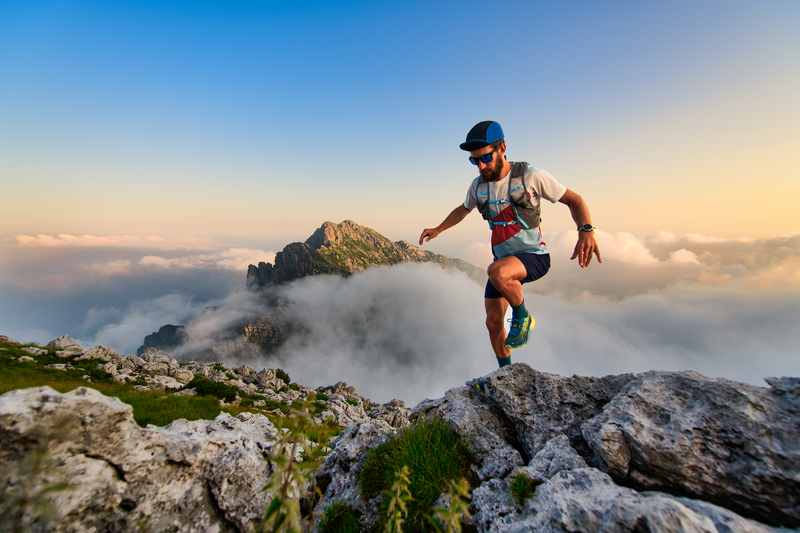
94% of researchers rate our articles as excellent or good
Learn more about the work of our research integrity team to safeguard the quality of each article we publish.
Find out more
ORIGINAL RESEARCH article
Front. Anim. Sci. , 26 April 2022
Sec. Animal Physiology and Management
Volume 3 - 2022 | https://doi.org/10.3389/fanim.2022.876504
This article is part of the Research Topic Biology Meets Technology: Aquatic Animals in Novel and New Aquaculture Production Systems View all 6 articles
A better understanding of recirculating aquaculture system (RAS) biosecurity is crucial for the sustainable and ethical production of Atlantic salmon smolt and post-smolt in these systems. This study described and evaluated the performance of a RAS facility for fish infection research with Atlantic salmon as the main animal model. Fish body weight, length, water quality, and system metrics from five independent experimental trials conducted between September 2020 and July 2021 were used to analyze the variation within and between treatments. Statistical power analysis was performed to determine the minimum number of fish required. The fish parameters variability showed that the inter-class correlation coefficient was on average low (0.1) and that the variation within tanks was larger than the variation between the tanks. The power analysis showed that 15 fish were required to be sampled per tank under these study conditions. Variation of water quality and system management metrics among the five experimental trials was higher compared to the variation within the five experimental trials. Moreover, the variation of the water quality parameters controlled by sensors was relatively low, whereas the parameters depending on biofilter maturation level and performance presented a very high variation. Water exchange rate-dependent quality parameters showed a similar variation value, i.e., nitrate and water turbidity. The established baseline for variability and performance presents an important reference for the design and realization of future experiments in RAS facilities. It is foreseen that the current research facility will develop new knowledge to improve the RAS biosecurity in the Atlantic salmon aquaculture industry.
Atlantic salmon (Salmo salar) is one of the world's major farmed finfish species with an annual production of around 2.4 million tons (FAO, 2020). Its aquaculture production cycle comprises two distinct phases: a land-based smolt production followed by a grow-out phase in sea cages until market size (Bergheim et al., 2009). Recently, the land-based production has been shifting from using traditional flow-through systems toward recirculating aquaculture systems (RASs), and it is estimated that up to 70% of smolts stocked in sea cages in Norway are from RASs (Meriac, 2019). The increased adoption of RASs to produce smolts and post-smolts is partially due to the benefits of a controlled production environment, including reduction of negative environmental impacts, a flexible location, and high biosecurity (Martins et al., 2010; Lazado and Good, 2021).
In theory, biosecurity is tighter in RASs than in other production systems such as flow-through systems, although pathogen breaches are still occurring and causing mass mortality events and high economic losses (Murray et al., 2014). Adversity of pathogens ranging from bacteria, viruses, fungi, and parasites (Noble and Summerfelt, 1996) may infect fish cultured in RASs. In salmonid RAS-based farming, infectious pancreatic necrosis virus (IPNV), the bacterial gill disease agents Flavobacterium spp. and Ca. Branchiomonas cysticola, or enteric red mouth disease agent Yersinia ruckeri are among the pathogens that pose a serious problem (Murray et al., 2014; Dopazo, 2020; Einarsdottir et al., 2020). The infection dynamics in RASs are different from other production systems, as the higher fish densities and nutrient availability (Mota et al., 2015) may enhance pathogen proliferation and infection rates.
The development of disinfection strategies to control pathogen outbreaks in the culture water and water treatment units in RASs without negatively affecting fish health and welfare or the nitrifying bacteria community in the biofilters is challenging. Infectious pathogens in fish research facilities are common (Kent et al., 2009), and several disinfection technologies such as ozone (Powell and Scolding, 2018), UV irradiation (Summerfelt et al., 2009b), or chemicals (e.g., peracetic acid (PAA) based products) added to the water (Good et al., 2020) were evaluated to address this issue. It is important to note that majority of the facilities being used to study diseases in Atlantic salmon under controlled conditions use flow-through systems. However, disinfection strategies applied in flow-through systems are usually not applicable in RASs as they do not consider the water reuse, RAS water chemistry, or the presence of complex equipment. With the current and expected increase in commercial production of Atlantic salmon smolt and post-smolt in RASs, there is an urgent need to improve biosecurity for RAS facilities.
An evaluation of novel aquaculture facilities is crucial to establishing fish and system performance standards and water quality limits. The previous studies evaluated how the theoretical design of a facility and treatment units matched in vivo experimental or grow-out studies on fish and system performance and showed the importance of establishing the water quality limits and system performance in both commercial and research facilities (Vinci et al., 2004; Summerfelt et al., 2009a; Wolters et al., 2009; Terjesen et al., 2013). However, to the best of the authors' knowledge, studies evaluating the equivalence of statistical inference parameters between independent RAS and conventional grow-out studies have not been conducted.
The necessary confinement of fish to containment units (tanks, ponds, cages, etc.) means that aquaculture growth trials are especially subjected to large variation, both within tanks (due to factors such as social interactions between fish) and large variations between tanks within treatments (due to environmental nuisance variables such as water quality parameters). This caused statistical challenges in both conventional aquaculture experiments and RAS experiments. In inferential statistics, two types of errors can occur. Type I errors are where a null hypothesis is incorrectly rejected due to chance; conventionally, the probability of this type of error is restricted to 5% by setting alpha (α) at 0.05. Type II errors fail to reject the null hypothesis when in fact there is a true treatment effect. This type of error is conventionally restricted by beta (β) to 20% (Cohen, 1992). Both types of error are costly because they result in incorrect conclusions and thus constitute a waste of limited experimental resources and crucially a waste of experimental fish.
The variation between tanks within treatment is especially poignant in aquaculture studies as these “tank effects” affect all fish within a tank independent of the treatment given and can be so large it is argued that fish within a tank are not statistically independent (Hurlbert, 1984). As a result, the studies analyzed fish as the independent experimental units, which constitutes “pseudo-replication” and grossly inflates Type I errors. The correct analysis is to take the mean of all fish within a tank, called “pooling,” as the response variable in an ANOVA or account for hierarchical data structure using nested ANOVAs for balanced data or mixed model ANOVAs for unbalanced (Ling and Cotter, 2003; Thorarensen et al., 2015). This is especially important in RAS research, as each tank must have its own independent RAS or else the tanks constitute pseudo-replicates and violate the assumption of statistical independence, and data must be analyzed at a mean level of RAS.
Type II errors are equally important in aquaculture studies and related to the statistical power, which should typically be 80%. However, statistical power in aquaculture experiments is rarely considered or reported, and when retrospectively calculated in meta-analyses it is often below 80% (Searcy-Bernal, 1994). According to Thorarensen et al. (2015), statistical power can be increased by (1) increasing the effect size of the treatment, (2) reducing the variation both within tanks and between tanks, (3) increasing the number of tanks, and (4) increasing the number of fish recorded within tanks. Since the number of tanks with an independent RAS will be limited and increasing treatment effect sizes is not always feasible a priori, the statistical power can only be increased by controlling and reducing the between tank environmental nuisance variables such as water quality parameters or increasing the number of fish recorded within tanks. In either case, documentation of the relative sources of variation is needed for planning future experiments.
The main objective of this study was to describe and evaluate a novel RAS facility for fish pathogens research with a focus on Atlantic salmon as an animal model. Specifically, this study characterized and evaluated the system performance during five experimental trials with Atlantic salmon conducted between September 2020 and July 2021. Also, this study estimated the variance between tanks and within tanks from five experiments to calculate the minimum values of fish samples required to achieve a relevant statistical power. Additionally, a disinfection protocol commonly used in salmon farms was employed to test whether it is sufficient to disinfect the system following experimental infection.
The experimental trials were carried out at the fish health laboratory of the Tromsø Aquaculture research station (Havbruksstasjonen i Tromsø AS, Kårvik, Norway) (Figure 1). The aquatic research facility has the Norwegian Food Safety Authority's approval to conduct infection trials in fish using a virus, bacteria, and parasites relevant for Atlantic salmon research (biosafety level 2). The facility wastewater is disinfected using a heat treatment (to 89°C for 15 min.).
The nine individual RAS units are located in one of the infection rooms of the research facility (Figure 2). Each individual RAS contains a cylindroconical experimental tank (V = 0.5 m3) with a dual outlet drain, an emergency oxygen stone, and a sensor for oxygen and temperature (Oxyguard®, Farum, Denmark). The water flow scheme across the different RAS units is shown in Figure 3. Briefly, the water flows out of the fish tank via a bottom center outlet and a sidewall outlet into a microscreen drum filter with a 40-μm screen to remove suspended solids. The backwash discharged from the microscreen is piped to a septic tank and to an underground pipe circuit (500 m long) where water is heated and disinfected (89°C for 15 min.). The microscreen drum filtrate flows into the moving bed bioreactor (MBBR, V = 0.2 m3, 50% filled with bio-media). The bio-media has a specific surface area of 750 m2/m3 (RK BioElements, RK Plast A/S, Skive, Denmark). MBBR media and degasser media are illustrated in Figure 4. The water flows to a pump sump (V = 0.1 m3), where the make-up water is added. Here, it is located a pH probe (K01SVPHD, OxyGuard International A/S, Farum, Denmark) and a water level sensor (KQ6001, ifm electronic gmbh, Essen, Germany). The combined flow is pumped into three water loops. In Loop 1, water (3,000 L/h) is pumped to the top of a degasser column to remove dissolved carbon dioxide (CO2), exits by gravity to a foam fractionator unit, and returns to the MBBR. The backwash foam is discharged to the septic tank pipeline. In Loop 2, water (600 L/h) is pumped to a protein skimmer column where ozone (Ozonizer S 1000, Erwin Sander Elektroapparatebau GmbH, Uetze-Eltze, Germany) is added and exits by the gravity back to the MBBR. In Loop 3, water (1,500 L/h) is pumped to a low-pressure oxygen saturation cone (0.6 bar), where pure oxygen (O2) gas is transferred to the water. Water exiting the oxygen cone flows partially to a chiller and heater unit (TK-1000, TECO®, Ravenna, Italy), to a UV-C unit (40 watt or 140 watt lamp, VGE B.V., Schijndel, the Netherlands), and finally to the fish tank. A vertical pipe with nozzles is used to inject the water back into the fish tank; the nozzles are oriented parallel toward the tank wall. The total RAS water volume is 0.8 m3. During the experiments described in this study, the RAS units were operating without the ozone generator and UV-C units as these units were added to the RAS posteriorly to the experiment's conclusion. The bio-media was pre-acclimatized for ~3–6 weeks using sodium bicarbonate (NaHCO3) and ammonium chloride (NH4Cl) solutions (Permakem AS, Lørenskog, Norway).
Figure 2. Detailed view of the RAS units. General view of the RAS unit's facility (A), fish tank view (B), and water treatment units view (C).
All procedures involving fish were authorized by the Norwegian Food Safety Authority (FOTS) under the general husbandry practices, ID 24383, ID 24128, and ID 26793. Fish handling and measurements were preceded by euthanasia with a bath overdose of benzocaine (Benzoak vet, 200 mg/ml, EuroPharma, Leknes, Norway).
Atlantic salmon eyed eggs (AquaGen Atlantic QTL-innOva PRIME, AquaGen AS, Trondheim, Norway) were hatched and fish were raised in a flow-through system (Tromsø Aquaculture Research Station, Kårvik, Norway) at ± 7.5°C under continuous light (LD 24:00) photoperiod until ~10–26 g of body weight.
Fish were fed continuously (~23 h/day) to satiation with commercial diets (1 and 2 mm pellet size, Nutra Olympic, Skretting, Norway) delivered through an automatic belt feeder. In experiment 5, fish were fed an experimental diet formulated for Atlantic salmon parr-smolt (2- and 3-mm pellet size, Nofima AS, Bergen, Norway).
Biological data, water quality, and system management parameters from five independent experimental trials conducted between September 2020 and July 2021 were used to evaluate the RAS units. The designs of these experiments and data collection are briefly described below.
A total of 495 juvenile Atlantic salmon (± 12 g) were randomly distributed among the nine RAS units. The fish were subjected to one of three treatments in triplicate. Treatments consisted of a control group, where fish were not exposed to Y. ruckeri; a single-entry group, where fish were exposed one time to a 24-h culture of Y. ruckeri administered via the make-up water and, a multi-entry group, where fish were exposed to a 24-h culture of Y. ruckeri administered via the makeup water on 3 consecutive days. The experimental period lasted 15 days; the final fish weight was16 g, the biomass was 453 g, and the overall specific growth rate was 1.92%/day. Fish survival and health and welfare were monitored over the experimental period.
A total of 450 juvenile Atlantic salmon (± 12 g), either previously infected with Y. ruckeri or uninfected, were distributed among the nine RAS units using the following infection matrix: Control = 0 infected fish and 50 uninfected fish per RAS unit; low pathogen load = 5 infected fish and 45 uninfected fish per RAS unit and a high pathogen load = 20 infected fish and 30 uninfected fish per RAS unit. All three treatments were run in triplicates. The experimental period lasted for 14 days; the final fish weight was16 g, the biomass was 493 g, and the overall specific growth rate was 2.05%/day. Fish survival and health and welfare were monitored over the experimental period.
A total of 360 juvenile Atlantic salmon (± 15 g) were randomly distributed among the nine RAS units and acclimatized for 1 week. The fish were subjected to three different treatments, with three replicated RAS per treatment. Treatments were a control (0.0 mg/L), low PAA concentration (0.1 mg/L), and a high PAA concentration (1 mg/L). PAA was administered continuously at the pump sump. The experimental trial lasted for 29 days; the final fish weight was 35 g, the biomass was 877 g, and the overall specific growth was of 2.92%/day. At the trial's conclusion, the individual body weight, fork length, and external welfare indicators of fish were registered.
A total of 360 juvenile Atlantic salmon (± 26 g) were randomly distributed among the nine RAS units and acclimatized for 1 week. The fish were subjected to three different treatments, with three replicates per treatment. Treatments were control (no PAA), pulse PAA (1 mg/L every 72 h), and continuous PAA (1 mg/L). The experimental trial lasted for 29 days; the final fish weight was 54 g, the biomass was 1,181 g, and the overall specific growth rate was 2.52%/day. At the trial's conclusion, the individual body weight, fork length, and external welfare indicators of fish were registered.
A total of 990 juvenile Atlantic salmon (± 19 g) were randomly distributed among the nine RAS units and acclimatized for 1.5 weeks. Fish were subjected to three different dietary treatments in triplicates: control diet, low-fat diet (−5% fat), and high-fat diet (+5% fat). The parr were smoltified using a square wave photoperiodic regime consisting of 6 weeks “winter signal” (LD 12:12), followed by 9 weeks of LD 24:0. The experimental trial lasted for 57 days; the final fish weight was 94 g, the biomass was 4,721 g, and the overall specific growth rate was 2.81%/d. At the trial's conclusion, the individual body weight, fork length, external welfare indicators, and external smoltification indicators of fish were registered.
Dissolved oxygen, pH, and temperature were measured weekly using a portable multi-meter (FDO 925 and Sentix 940 sensors, Multi 3630 IDS, Xylem Analytics Germany Sales GmbH & Co., Weilheim, Germany). Carbon dioxide was measured two times a month using a portable meter (Dissolved CO2 sensor, OxyGuard Pacific, OxyGuard International A/S, Farum, Denmark). Turbidity was measured two times a month from water samples using a portable meter (ORION AQ4500, Thermo Scientific®, Thermo Fisher Scientific, Nijkerk, the Netherlands). Ammonium (NH4-N), nitrite (NO2-N), and nitrate (NO3-N) were measured weekly or bi-monthly from water samples using a spectrophotometer (Test Kit 1.14558.001, 1.14776.0001, and 1.14942.0001, Spectroquant®, Merck KGaA, Darmstadt, Germany). Ammonia (NH3-N) concentrations were calculated from the ammonium concentration as a function of pH, temperature, and salinity (Johansson and Wedborg, 1980). All measurements and water samples were obtained from each fish tank. Unless stated otherwise, fish in the RAS units were maintained as follows: dissolved oxygen > 85% saturation, pH 7–7.5, temperature 11.5–12.5°C, salinity < 2 ppt, and photoperiod 24-h light.
The standard routine for cleaning and disinfection of the systems consisted of flushing the system water, wet cleaning the surfaces with a highly alkaline hypochlorite-containing detergent (Enduro Hypo, Lilleborg AS, Oslo, Norway), and ending with a second water flushing.
A second disinfection protocol was tested to specifically eliminate pathogens and it was done two times, at the end of Experiments 1 and 2. The protocol consisted of filling the RAS units with fresh water and starting the circulating pumps, so the water circulated through all units. Then, a sulfuric acid solution (Cid non-P, DeLaval N.V., Gent, Belgium) was added until the water pH was < 4 and the system was run for 24 h. After this period, the water was removed, and new freshwater was added. Then, sodium hydroxide pellets (≥ 97% NaOH, Emplura® Merck KGaA, Darmstadt, Germany) were added until the pH was > 12, and the RAS units were left running for 48 h. After this disinfection procedure, swabs were collected from (1) fish tank walls, (2) fish tank inlet pipes, (3) fish tank outlet pipes, and (4) foam fractionator walls and were kept in Eppendorf tubes previously filled with RNAlater® solution (Invitrogen, Thermo Fisher Scientific Baltics UAB, Vilnius, Lithuania) and stored at 4°C until assayed. The swab samples were sent to an external service laboratory (PHARMAQ Analytiq AS, Bergen, Norway) for the detection of Y. ruckeri by real-time RT-PCR.
Statistical analysis was conducted on growth data (fish body weight and fork length) collected at the end of the experiments. For each of the data sets, a nested ANOVA (also called hierarchical ANOVA) was run using linear mixed effect models with restricted maximum likelihood in the “nlme” package (Pinheiro et al., 2017) of R statistical software (R Core Team, 2019) and had the following form:
where yijk is the measurement of the kth fish, within the jth tank and ith treatment. Treat is the fixed effect of the ith treatment (i = 3 levels across datasets), Treati(Tankj) is the random effect of tanks nested within treatments, which was assumed to be normally distributed (ND) ~ (0,I), and eijk is the random residual error term assumed to be ND ~ (0,I).
The intra-class correlation coefficient for replicate tanks within treatment was calculated as (ICC) = /( + ). The effect size f was calculated as f = where μD is the difference between effects in Treati. The realized power was calculated using the wp.crt3arm function of the R package “WebPower” (Zhang and Mai, 2019). A power analysis was conducted for the standard system setup of nine tanks with three treatments assigned three replicate tanks each for a range of fish per tank from 1 to 60. The sensitivity of the power analysis was evaluated using a range of ICC for tanks nested in treatments set to 0, 0.1, and 0.2, and the three standardized effect sizes (small = 0.2, medium = 0.5, and large = 0.8) as defined by (Cohen, 1988).
Table 1 lists fish body weight and fork length figures derived from the five experimental trials at the terminal sampling. The inter-class correlation coefficient (ICC) was 0.1 on average, ranging from 0.0 to 0.4. Overall, the variation within tanks (CVe) was larger than the variation between tanks (CV): 36 vs. 11% for weight and 6 vs. 2% for length, respectively, CVe and CV. The largest difference was observed for the weight, where the CVe values ranged from 22 to 97%, whereas the CV values ranged from 5 to 20%. The average realized power of the five experiments was 59% for weight and 47% for length. The realized power for weight presented the highest variation among experiments, ranging from 21 to 95%.
The statistical power vs. number of fish sampled for three treatment effect sizes (small = 0.2, medium = 0.5, and large = 0.8) (Cohen, 1988) at three ICC (0.0, 0.1, and 0.2) is shown in Figures 5A–C. Here, it is observable that: (1) statistical power decreases with ICC increase, (2) statistical power increases with treatment effect increase, and (3) statistical power increases with the increase of the number of fish sampled per tank. Assuming the maximum available number of tanks at the fish health laboratory RAS facility, i.e., nine with three tanks per treatment, to achieve a power of 80% (a conventional choice), a medium to large treatment effect (0.5–0.8), an ICC of 0, and a minimum of 15 fish sampled per tank are necessary.
Figure 5. Graph of the statistical power vs. number of fish sampled for three treatment effect sizes (small = 0.2, medium = 0.5 and large = 0.8). (A–C) Three ICC values, 0.1, 0.2, and 0.2, respectively. Data for this study experimental units, i.e., nine fish tanks with three tanks per treatment.
Average water quality parameters from the five experimental trials are summarized in Table 2.
The CVExp. values (among the five experimental trials) were on average 243.7% higher compared to CVTreat. values (within the five experimental trials). The CVExp. values of the water quality parameters controlled by sensors such as dissolved oxygen, pH, and temperature were relatively low 1.7–3.6%, whereas parameters depending on biofilter maturation level and performance such as NH4-N/NH3-N and NO2-N presented a very high CVExp. 146.4–202.6% among the five experimental trials. The water quality parameters where concentration depended on the water dilution level showing similar CVExp. values, i.e., 104.6 (NO3-N) and 108.6% (turbidity), to the water exchange rate value of 99.3%. System management metrics (Table 2) showed a high CVExp. (20.3–115.8%) with parameters ranging from 0.6 to 5.9 kg/m3 for maximum fish density, 2.0 and 13.0 h for system mean hydraulic retention time, or 687–1,579 L/kg feed for average water exchange rate. The CVTreat. values for the parameters analyzed showed the same trend as described above for the CVExp. values but at a lower magnitude.
The real-time RT-PCR results from all 18 swabs were negative for the presence of Y. ruckeri.
In this study, data from five independent experimental trials were used to establish a variation baseline for fish performance, water quality, and system management metrics of a novel and unique research facility for pathogen research in RAS. A statistical power analysis model was developed for different experimental scenarios and can be used as a tool to reduce and refine the number of animals for experimental use. Moreover, the design of the nine single RAS units was described in detail to facilitate the planning and design of future experiments to address biosecurity challenges in the Atlantic salmon RAS industry.
The current RAS facility reuses water and this feature opens a new pathogen research area: how to eliminate pathogens in water. Ozone is a powerful oxidant that has been used to improve water quality and reduce pathogens (Powell and Scolding, 2018). Several studies have addressed the effect of ozone on Atlantic salmon health in RASs (Davidson et al., 2021; Lazado et al., 2021) establishing ozone thresholds (Stiller et al., 2020). Ultraviolet (UV) irradiation is also being widely applied within aquaculture systems as water treatment (Summerfelt, 2003). This study evaluated the efficacy of a chemical disinfection method that combined a low-high pH cycle to eliminate pathogens, in this specific case the bacterium Y. ruckeri. The results from this study confirmed the effectiveness of this method to eliminate Y. ruckeri and recommends the use of this protocol between trials when needed. However, fewer studies had addressed the efficacy of these disinfection technologies in a RAS stocked with infected fish, which is now possible with the current facility.
The evaluation and description of novel research facilities are important for effective resource utilization, high-quality scientific output, and to raise awareness about the research facility for national and transnational collaboration. For example, Wolters et al. (2009) evaluated the fish culture systems, water quality, and fish loading of a RAS for Atlantic salmon at the National Cold Water Marine Aquaculture Center (Maine, USA). These authors described the water treatment components, water process flow, and water quality limits for the target species, information that can be used for other research and industry users to develop and refine their own production systems. Another study by Summerfelt et al. (2009a) evaluated the partial water reused systems for Atlantic salmon smolt production and described among other parameters, the diurnal and fish tank fluctuations of different water quality parameters, such as dissolved oxygen, temperature, and water velocity. Data on parameter variation within RASs are very relevant as it helps to design and dimension new RAS facilities. The research facilities are required to go even further, as apart from the requirements of optimal fish performance and water quality, they need to supply reliable and statistically powerful data. For example, Terjesen et al. (2013) described a facility for research studies on the requirements of fish in RAS environments, which among other features included multiple fish tanks connected to a single water treatment, resulting in the replicated unit being the fish tank. From a statistical perspective, this design is a pseudo-replication (Hurlbert, 1984); however, the design of this facility follows the standards of commercial RAS facilities (Vinci et al., 2004; Summerfelt et al., 2009a; Wolters et al., 2009) but in an experimental scale. It has the advantage of providing the same water quality across all experimental tanks, which is relevant when the research study object is the fish and its physiological requirements in a RAS environment. In contrast, the research facility from this study comprises nine identical single tank RASs, and, therefore, the replicated unit is the whole RAS. The design of the current systems has the advantage that each RAS unit is considered an independent replicate, thus addressing issues on pseudo-replication which is considerable in a number of RAS-related research. The latter can be defined as the use of inferential statistics to test treatment effects with data from experiments where either treatments are not replicated (though samples may be) or replicates are not statistically independent (Hurlbert, 1984).
One way to estimate the statistical independence of tanks is to partition variation into between tank variation within treatments and within tank variation. In general, the ratio of between-tank variance within treatments to total variance is expressed as the Intraclass correlation coefficient (ICC) and is a good indication of the statistical independence of tanks. If ICC = 0, the replicates are statistically independent, if the ICC = 1, the replicates are completely dependent, and differences are completely due to “tank effects.” In this study, the ICC was on average low (0.1), indicating low good reproducibility of replicates within the treatment. The between-tank and within-tank variance can be also examined separately. We evaluated five RAS experimental trials, and we found the within-tank variation (CVe) to be consistently higher than the between-tank variation CVB for weight CVe (36%) and CV (6%,) and for length CVe (11%) and CV (2%). These values are comparable to a previous study that evaluated the bodyweight of Atlantic salmon from 11 conventional flow-through growth trials and found a wide range of CV values (Ling and Cotter, 2003); on average, the variation within tanks (CVe) was larger than the variation between tanks (CV): 28 and 3%, respectively. Experimental fish in the five RAS trials of this study were exclusively juvenile Atlantic salmon parr, and some of the variations can be explained by a bimodal size-frequency distribution commonly observed in populations of Atlantic salmon during early life stages (Thorpe, 1977). Thorarensen et al. (2015) similarly reported CVe ranging from 30.6% and a lower CV ranging from 4.5% for body weight across trials involving Atlantic halibut (Hippoglossus hippoglossus), turbot (Scophthalmus maximus), Shire tilapia (Oreochromis shiranus), Arctic charr (Salvelinus alpinus), and Atlantic cod (Gadus morhua). Overall, the relatively low CV reported in this study is a good indication that the order of magnitude of the tank/RAS variance is low. Consequently, the research facility shows great promise for the replicability of the experimental conditions, which is especially important for complex biological and technological environments such as RAS, with the added benefit that the RAS units are independent and do not violate statistical assumptions.
Water quality criteria for aquaculture systems typically consider temperature, dissolved oxygen, pH, and total ammonia nitrogen. However, in RAS, we may need to consider those parameters together with parameters related to water reuse and biofiltration metabolites, such as turbidity, nitrite, and nitrate (Colt, 2006; Mota et al., 2014, 2015; Aslam et al., 2019). Differences in these water quality parameters will contribute to the between-tank variation if they are not controlled. Moreover, different fish species and life stages have different water quality optima (Thorarensen and Farrell, 2011; Mota et al., 2019). Water quality fluctuations can occur within the day and different units of RAS, depending on numerous factors such as feeding regime (continuous vs. pulse), level of automation or sensors, and system management. For example, a feed load leads to the increase of several metabolites in the RAS water such as CO2, TAN, nitrite, nitrate, and a decrease in the pH (Terjesen et al., 2013). In this study, the variation of controlled variables by sensors such as dissolved oxygen, pH, and temperature showed a relatively low variation both within (1.0–1.6%) and among (1.7–3.6%) the different experiments. This result illustrates the increasing importance of sensors and automation in RAS and aquaculture in general to obtain stable and standard rearing conditions. In contrast, the water quality parameters that depend on the biofilter maturation and performance such as NH4-N/NH3-N and NO2-N presented a very high variation both within (45.4–60.0%) and among (146.4–202.6%) the different experiments. The slow development of nitrifying bacteria makes the biofilter less efficient during the activation period, causing reduced water quality due to increased concentrations of ammonia and nitrite, which can be harmful for the fish or inhibit further microbial biofilter development (Roalkvam et al., 2021). Several factors can impact the development of the microbial communities such as temperature and salinity of the biofilter, and this is an area of current research in RAS (Kinyage and Pedersen, 2016; Navada et al., 2019). Another factor to consider is the start-up of the nitrifying biofilter, a process that can take several months and be especially critical in facilities that need to disinfect the bio-media between experimental trials, like the current facility. A solution to accelerate the start-up of the biofilter can be the use of commercially available inoculums (Ruiz et al., 2020) or mature bio-media kept frozen. Moreover, water exchange rate-dependent parameters, such as nitrate (NO3-N), and dissolved solids (turbidity) showed a relatively low variation within individual experiments but high variation among the 5 experiments.
The power of a statistical test null hypothesis (H0) is the probability that the H0 will be rejected when it is false, in other words failing to detect a true treatment effect (Cohen, 1992). The retrospective statistical power across growth trials in the present study was on average 0.59 for weight and 0.47 for length. These values are in line with the studies reported by Thorarensen et al. (2015), which had an average statistical power of 54% ranging from 12 to 100% across numerous aquaculture species and support the notion that achieving a statistical power of 80% is challenging in aquaculture trials. An important consideration in interpreting the current power analyses is that not all of the 5 trials aimed at testing treatment effects on weight and length. Rather, we conducted this exercise to document the performance of the RAS facility for a prior power calculation for future trials using this facility. The results from this study show that it is possible to combine both by assuming the maximum available number of tanks at the fish health laboratory RAS facility, i.e., nine with three tanks per treatment, to achieve a power of 80% (a conventional choice), and a medium to large treatment effect (0.5–0.8) an ICC of 0 is necessary and a minimum of 15 fish sampled per tank. Other options available to increase the statistical power involve (i) increasing the treatment effect, (ii) sampling a larger number of fish per tank, (iii) reducing the within-tank variation either through reducing measurement error or through narrowing the range of sizes of fish entered the trial, and (iv) controlling factors that contribute to between-tank variation.
Animal-based research must balance safeguarding animal welfare while enabling high-quality science (Clark, 2018). The 3Rs of Replacement, Reduction, and Refinement are nowadays fundamental principles in any experimental design and application to the Animal Welfare Committee. For example, the Norwegian Food Safety Authority (Mattilsynet) requires approaching the 3Rs when developing an experimental study, including a justification of the number of animals used preferably using a statistical method such as power analysis. The results obtained in this study showed sampling a minimum of 15 animals is required per RAS to achieve a robust power design for growth in the RAS facility evaluated. Nevertheless, one may need to be aware that sampling more or less fish than those reported here can be necessary, depending on the variation associated with the research question, expected effect sizes, and response variables of interest.
This study describes and evaluates a RAS facility specifically designed to conduct research on Atlantic salmon pathogen infection dynamics and respective disinfection strategies. A number of four major objectives were taken into account when designing and building this facility: (1) to establish challenge models with pathogens using in vivo fish, (2) to offer flexibility on disinfection technologies including ozonation, UV irradiation, and water chemicals, (3) to produce industry-relevant fish performance and water quality within optimal levels for Atlantic salmon, and (4) to have identical and independent replicated RAS units to support robust experimental designs and statistical data analysis. The data from the five trials analyzed indicate that the facility successfully delivers the expected results, and it is expected to be useful for developing new knowledge to improve the RAS biosecurity in the Atlantic salmon aquaculture industry.
The original contributions presented in the study are included in the article/supplementary material, further inquiries can be directed to the corresponding author.
The animal study was reviewed and approved by Norwegian Food Safety Authority (FOTS) under general husbandry practices, ID 24383, ID 24128, and ID 26793.
VM, GV, GD, and CL prepared the original project concept and formulated the study design and project objectives. VM, AS, and GD performed the data interpretation. GD performed the statistical analysis. VM wrote the first manuscript draft with input from all co-authors. All authors contributed to the article and approved the submitted version.
This work was funded by The Research Council of Norway, Basic funding Nofima AS, project no. 13310 RASLab in focus: infrastructure audit and establishment of SOPs for future research, The Research Council of Norway, Young Research Talent Program, project no. 302767 Water disinfection strategies to improve Atlantic salmon parr production, and The Research Council of Norway, Strategic Institute Research Nofima AS, project no. 12878 Atlantic salmon biology in focus: for a robust fish in a shifting aquaculture industry.
The authors declare that the research was conducted in the absence of any commercial or financial relationships that could be construed as a potential conflict of interest.
All claims expressed in this article are solely those of the authors and do not necessarily represent those of their affiliated organizations, or those of the publisher, the editors and the reviewers. Any product that may be evaluated in this article, or claim that may be made by its manufacturer, is not guaranteed or endorsed by the publisher.
The authors would like to thank the staff at the Tromsø Aquaculture Research Station (Havbruksstasjonen i Tromsø AS) for technical support during the installation of the RAS units and during the experimental work, in special to Rune Olsen, Thomas Fagerheim, Daniel Johnsen, Morten Marienborg and Astrid-Elisabeth Chr. Hanssen. To thank the staff of Nofima AS for the support during the acquisition of the RAS units, in special to Arne Mikal Arnesen, Hilde Toften, Gunn Berit Olsson, Kari Grønlien, Lill-Heidi Johansen, and Roy-Inge Hansen. The authors would also like to thank the master students Maia Eggen, Kari Justad, Danilo Carletto, and Lena Aas for their assistance during water and fish sampling and data collection and analysis. The authors appreciate the assistance of AquaBioTech Group (Mosta, Malta) for supplying the RAS units, ZEBCARE (Nederweert, the Netherlands) for supplying the UVC and ozone generator units and Science Crunchers (Lisbon, Portugal) for RAS units sketches.
Aslam, S. N., Navada, S., Bye, G. R., Mota, V. C., Terjesen, B. F., and Mikkelsen, Ø. (2019). Effect of CO2 on elemental concentrations in recirculating aquaculture system tanks. Aquaculture 511, 734254. doi: 10.1016/j.aquaculture.2019.734254
Bergheim, A., Drengstig, A., Ulgenes, Y., and Fivelstad, S. (2009). Production of Atlantic salmon smolts in Europe—current characteristics and future trends. Aquacult. Eng. 41, 46–52. doi: 10.1016/j.aquaeng.2009.04.004
Clark, J. M. (2018). The 3Rs in research: a contemporary approach to replacement, reduction and refinement. Br. J. Nutr. 120, S1–S7. doi: 10.1017/S0007114517002227
Cohen, J. (1992). Statistical power analysis. Curr. Direct. Psychol. Sci. 1, 98–101. doi: 10.1111/1467-8721.ep10768783
Colt, J. (2006). Water quality requirements for reuse systems. Aquacult. Eng. 34, 143–156. doi: 10.1016/j.aquaeng.2005.08.011
Davidson, J., Summerfelt, S., Espmark, Å. M. O., Mota, V. C., Marancik, D., Earley, R. L., et al. (2021). Effects of ozone on post-smolt Atlantic salmon (Salmo salar) performance, health, and maturation in freshwater recirculation aquaculture systems. Aquaculture 533, 736208. doi: 10.1016/j.aquaculture.2020.736208
Dopazo, C. P. (2020). The infectious pancreatic necrosis virus (IPNV) and its virulence determinants: what is known and what should be known. Pathogens 9, 94. doi: 10.3390/pathogens9020094
Einarsdottir, T., Guttormsdottir, G., Connaghan, D., and Hjartardottir, S. (2020). Longitudinal survey of flavobacterium species in Icelandic salmonid fish farms. Dis. Aquat. Organ. 141, 15–24. doi: 10.3354/dao03508
Good, C., Davidson, J., Straus, D. L., Harper, S., Marancik, D., Welch, T., et al. (2020). Assessing peracetic acid for controlling post-vaccination Saprolegnia spp.-associated mortality in juvenile Atlantic salmon Salmo salar in freshwater recirculation aquaculture systems. Aquacult. Res. 51, 2624–2627. doi: 10.1111/are.14567
Hurlbert, S. H. (1984). Pseudoreplication and the design of ecological field experiments. Ecol. Monogr. 54, 187–211. doi: 10.2307/1942661
Johansson, O., and Wedborg, M. (1980). The ammonia-ammonium equilibrium in seawater at temperatures between 5 25 C. J. Solut. Chem. 9, 37–44. doi: 10.1007/BF00650135
Kent, M. L., Feist, S. W., Harper, C., Hoogstraten-Miller, S., Mac Law, J., Sanchez-Morgado, J. M., et al. (2009). Recommendations for control of pathogens and infectious diseases in fish research facilities. Compar. Biochem. Physiol. Part C Toxicol. Pharmacol. 149, 240–248. doi: 10.1016/j.cbpc.2008.08.001
Kinyage, J. P. H., and Pedersen, L.-F. (2016). Impact of temperature on ammonium and nitrite removal rates in RAS moving bed biofilters. Aquacult. Eng. 75, 51–55. doi: 10.1016/j.aquaeng.2016.10.006
Lazado, C. C., and Good, C. (2021). Survey findings of disinfection strategies at selected Norwegian and North American land-based RAS facilities: a comparative insight. Aquaculture 532, 736038. doi: 10.1016/j.aquaculture.2020.736038
Lazado, C. C., Stiller, K. T., Reiten, B.-K. M., Osório, J., Kolarevic, J., and Johansen, L.-H. (2021). Consequences of continuous ozonation on the health and welfare of Atlantic salmon post-smolts in a brackish water recirculating aquaculture system. Aquat. Toxicol. 238, 105935. doi: 10.1016/j.aquatox.2021.105935
Ling, E. N., and Cotter, D. (2003). Statistical power in comparative aquaculture studies. Aquaculture 224, 159–168. doi: 10.1016/S0044-8486(03)00225-4
Martins, C. I. M., Eding, E. H., Verdegem, M. C. J., Heinsbroek, L. T. N., Schneider, O., Blancheton, J. P., et al. (2010). New developments in recirculating aquaculture systems in Europe: A perspective on environmental sustainability. Aquacult. Eng. 43, 83–93. doi: 10.1016/j.aquaeng.2010.09.002
Meriac, A. (2019). Smolt Production and the Potential for Solid Waste Collection in Norway. Tromsø: Nofima AS.
Mota, V. C., Limbu, P., Martins, C. I., Eding, E. H., and Verreth, J. A. (2015). The effect of nearly-closed RAS on the feed intake and growth of Nile tilapia (Oreochromis niloticus), African catfish (Clarias gariepinus) and European eel (Anguilla anguilla). Aquacult. Eng. 68, 1–5. doi: 10.1016/j.aquaeng.2015.06.002
Mota, V. C., Martins, C. I., Eding, E. H., Canário, A. V., and Verreth, J. A. (2014). Steroids accumulate in the rearing water of commercial recirculating aquaculture systems. Aquacult. Eng. 62, 9–16. doi: 10.1016/j.aquaeng.2014.07.004
Mota, V. C., Nilsen, T. O., Gerwins, J., Gallo, M., Ytteborg, E., Baeverfjord, G., et al. (2019). The effects of carbon dioxide on growth performance, welfare, and health of Atlantic salmon post-smolt (Salmo salar) in recirculating aquaculture systems. Aquaculture 498, 578–586. doi: 10.1016/j.aquaculture.2018.08.075
Murray, F., Bostock, J., and Fletcher, D. (2014). Review of Recirculation Aquaculture System Technologies and Their Commercial Application. Glasgow: Stirling Aquaculture.
Navada, S., Vadstein, O., Tveten, A.-K., Verstege, G. C., Terjesen, B. F., Mota, V. C., et al. (2019). Influence of rate of salinity increase on nitrifying biofilms. Journal of Clean. Product. 238, 117835. doi: 10.1016/j.jclepro.2019.117835
Noble, A. C., and Summerfelt, S. T. (1996). Diseases encountered in rainbow trout cultured in recirculating systems. Ann. Rev. Fish Dis. 6, 65–92. doi: 10.1016/S0959-8030(96)90006-X
Pinheiro, J., Bates, D., Debroy, S., Sarkar, D., Heisterkamp, S., Van Willigen, B., et al. (2017). “Package ‘nlme'. Linear and Nonlinear Mixed Effects Models. Version 3.1”. Technical Report.
Powell, A., and Scolding, J. W. (2018). Direct application of ozone in aquaculture systems. Rev. Aquacult. 10, 424–238. doi: 10.1111/raq.12169
R Core Team (2019). R: A Language and Environment for Statistical Computing (Version 3.6. 1)[Computer software]. R Foundation for Statistical Computing.
Roalkvam, I., Drønen, K., Dahle, H., and Wergeland, H. I. (2021). A case study of biofilter activation and microbial nitrification in a marine recirculation aquaculture system for rearing Atlantic salmon (Salmo salar L.). Aquacult. Res. 52, 94–104. doi: 10.1111/are.14872
Ruiz, P., Vidal, J. M., Sepúlveda, D., Torres, C., Villouta, G., Carrasco, C., et al. (2020). Overview and future perspectives of nitrifying bacteria on biofilters for recirculating aquaculture systems. Reviews in Aquaculture 12, 1478–1494. doi: 10.1111/raq.12392
Searcy-Bernal, R. (1994). Statistical power and aquacultural research. Aquaculture 127, 371–388. doi: 10.1016/0044-8486(94)90239-9
Stiller, K. T., Kolarevic, J., Lazado, C. C., Gerwins, J., Good, C., Summerfelt, S. T., et al. (2020). The effects of ozone on Atlantic salmon post-smolt in brackish water—Establishing welfare indicators and thresholds. Int. J. Mol. Sci. 21, 5109. doi: 10.3390/ijms21145109
Summerfelt, S., Sharrer, M., Gearheart, M., Gillette, K., and Vinci, B. (2009a). Evaluation of partial water reuse systems used for Atlantic salmon smolt production at the white river national fish hatchery. Aquacult. Eng. 41, 78–84. doi: 10.1016/j.aquaeng.2009.06.003
Summerfelt, S. T. (2003). Ozonation and UV irradiation—an introduction and examples of current applications. Aquacult. Eng. 28, 21–36. doi: 10.1016/S0144-8609(02)00069-9
Summerfelt, S. T., Sharrer, M. J., Tsukuda, S. M., and Gearheart, M. (2009b). Process requirements for achieving full-flow disinfection of recirculating water using ozonation and UV irradiation. Aquacult. Eng. 40, 17–27. doi: 10.1016/j.aquaeng.2008.10.002
Terjesen, B. F., Summerfelt, S. T., Nerland, S., Ulgenes, Y., Fjæra, S. O., Reiten, B. K. M., et al. (2013). Design, dimensioning, and performance of a research facility for studies on the requirements of fish in RAS environments. Aquacult. Eng. 54, 49–63. doi: 10.1016/j.aquaeng.2012.11.002
Thorarensen, H., and Farrell, A. P. (2011). The biological requirements for post-smolt Atlantic salmon in closed-containment systems. Aquaculture 312, 1–14. doi: 10.1016/j.aquaculture.2010.11.043
Thorarensen, H., Kubiriza, G. K., and Imsland, A. K. (2015). Experimental design and statistical analyses of fish growth studies. Aquaculture 448, 483–490. doi: 10.1016/j.aquaculture.2015.05.018
Thorpe, J. (1977). Bimodal distribution of length of juvenile Atlantic salmon (Salmo salar L.) under artificial rearing conditions. J. Fish Biol. 11, 175–184. doi: 10.1111/j.1095-8649.1977.tb04111.x
Vinci, B. J., Summerfelt, S. T., Creaser, D. A., and Gillette, K. (2004). Design of partial water reuse systems at white river NFH for the production of Atlantic salmon smolt for restoration stocking. Aquacult. Eng. 32, 225–243. doi: 10.1016/j.aquaeng.2004.07.003
Wolters, W., Masters, A., Vinci, B., and Summerfelt, S. (2009). Design, loading, and water quality in recirculating systems for Atlantic salmon (Salmo salar) at the USDA ARS national cold water marine aquaculture center (Franklin, Maine). Aquacult. Eng. 41, 60–70. doi: 10.1016/j.aquaeng.2009.06.011
Zhang, H., and Mai, Y. (2019). WebPower: Basic and Advanced Statistical Power Analysis. Analysis. R package version 0.6. Available online at: https://CRAN.R-project.org/package=WebPower
Keywords: recirculating aquaculture system (RAS), fish health, pathogens, infection challenge models, biosecurity, power analysis and sample size-analysis
Citation: Mota VC, Striberny A, Verstege GC, Difford GF and Lazado CC (2022) Evaluation of a Recirculating Aquaculture System Research Facility Designed to Address Current Knowledge Needs in Atlantic Salmon Production. Front. Anim. Sci. 3:876504. doi: 10.3389/fanim.2022.876504
Received: 15 February 2022; Accepted: 14 March 2022;
Published: 26 April 2022.
Edited by:
Amira Leila Dib, Constantine 1 University, AlgeriaReviewed by:
Weinan Zhou, University of Illinois at Urbana-Champaign, United StatesCopyright © 2022 Mota, Striberny, Verstege, Difford and Lazado. This is an open-access article distributed under the terms of the Creative Commons Attribution License (CC BY). The use, distribution or reproduction in other forums is permitted, provided the original author(s) and the copyright owner(s) are credited and that the original publication in this journal is cited, in accordance with accepted academic practice. No use, distribution or reproduction is permitted which does not comply with these terms.
*Correspondence: Vasco C. Mota, dmFzY28ubW90YUBub2ZpbWEubm8=
Disclaimer: All claims expressed in this article are solely those of the authors and do not necessarily represent those of their affiliated organizations, or those of the publisher, the editors and the reviewers. Any product that may be evaluated in this article or claim that may be made by its manufacturer is not guaranteed or endorsed by the publisher.
Research integrity at Frontiers
Learn more about the work of our research integrity team to safeguard the quality of each article we publish.