- 1Department of Biochemistry and Molecular Biology, Faculty of Chemistry, Complutense University of Madrid, Madrid, Spain
- 2The Peter Gorer Department of Immunobiology, King's College London, London, United Kingdom
- 3The Francis Crick Institute, London, United Kingdom
The “epithelial barrier hypothesis” states that a barrier dysfunction can result in allergy development due to tolerance breakdown. This barrier alteration may come from the direct contact of epithelial and immune cells with the allergens, and indirectly, through deleterious effects caused by environmental changes triggered by industrialization, pollution, and changes in the lifestyle. Apart from their protective role, epithelial cells can respond to external factors secreting IL-25 IL-33, and TSLP, provoking the activation of ILC2 cells and a Th2-biased response. Several environmental agents that influence epithelial barrier function, such as allergenic proteases, food additives or certain xenobiotics are reviewed in this paper. In addition, dietary factors that influence the allergenic response in a positive or negative way will be also described here. Finally, we discuss how the gut microbiota, its composition, and microbe-derived metabolites, such as short-chain fatty acids, alter not only the gut but also the integrity of distant epithelial barriers, focusing this review on the gut-lung axis.
Allergy: a pandemic of the 21st century
Allergic diseases are characterized by immunological alterations that drive to hyperresponsiveness mainly against protein components called allergens. These molecules are present in a plethora of natural sources (house dust mites, molds, pollens, bee or wasp venoms, vegetable and animal foods, etc.), and lead to many different inflammatory diseases such as asthma, allergic rhinitis, food allergy (FA) or atopic dermatitis (AD) (1).
Worldwide prevalence of allergic diseases has dramatically increased during the last decades (2–5). Asthma, food allergy, and atopic dermatitis are considered the most relevant allergic diseases, and the connections to each other are still under research. Asthma is a chronic disease where the allergen avoidance is often difficult. Proper diagnosis and follow-up are required, especially in patients displaying severe symptoms, whose main treatment implies the administration of corticosteroids, adjusting doses to the minimum effective ones and/or controlling disease exacerbations (6).
Food allergies affect around 5%–8% of global population, and is increasing every year, causing deaths, alteration of life quality and health cost (7, 8). Nowadays, food allergy development is mainly explained by the “dual allergen exposure hypothesis”, which addresses that food allergen exposure through inflamed skin before exposure to the alimentary tract might lead to the development of FA (9). Accordingly, avoidance of allergenic foods during pregnancy until the first year of the child's life has been widely recommended from decades (10). However, more recent studies suggest that this practice induces an increase on IgE sensitization (11–13). Conversely, the introduction of food allergens during early life could even induce immune tolerance, as concluded by the PreventADALL study that found that exposure to allergenic foods from 3 months of age reduced FA at 36 months in a general population (14). So far, management of food allergies follows two major strategies: focusing the initial symptoms or long-term treatments (15). One of the main strategies against FAs is the avoidance of allergen ingestion or contact; however, around 11% of allergic reactions are caused due to non-accidental contacts (7). A correct diagnosis of FA is key for the avoidance of allergic reactions; nowadays, the gold standard for food allergy diagnosis is the Oral Food Challenge, but other methods are also used, including blood IgE level measurements, Skin Prick Tests, physical examination, and anamnesis (16, 17). Most common allergic components of the diet are cow's milk, tree nuts, peanut, shellfish and vegetables, as determined by the US National Health and Nutrition Examination Survey (NHANES) (18).
Atopic dermatitis is the main inflammatory skin disease, whose prevalence reaches 20% in children and 3% in adults (19) producing several alterations of life quality. AD is driven by various pathophysiological mechanisms, including genetic factors, and is related to other diseases such as asthma, allergic rhinitis, and food allergy (20–22). In fact, AD is considered by several authors as the first cause that could trigger subsequent allergenic processes. The mechanisms of AD are multifactorial, involving barrier dysfunction and Th2 response, with a main role of IL-4 and IL-13, but also IL-31, IL-17, IL-22 and TSLP (23). Two types of AD have been described: the extrinsic form, with an IgE-mediation; and the intrinsic form, not IgE mediated (19). AD patients often manifest eczema with excoriation and serous exudation, and the treatment is based on corticosteroids, cyclosporine for dupilumab for patients that do not respond to topical therapies (24).
Mechanistically, allergic reaction development starts with the recognition of allergens by the immune system. Under certain conditions, this recognition triggers a reaction called sensitization phase, that primes naïve B cells into differentiating plasma cells, that finally produce specific IgE against the allergen. As stated by “the atopic march theory”, skin barrier impairment during infancy and the development of AD acts as key drivers for further development of asthma or FAs due to cytokine release and defective barrier function (25). Even though not shared by all the allergic patients, a common feature is the disruption of an epithelial barrier during allergy development, mainly those from skin, gut, and lungs, matching the three more prevalent allergic diseases. The epithelial barriers constitute the main surfaces of contact between the inside of the body and the outside world and are strongly involved in the outcome of the immune responses, playing an active role in the sensitization phase during allergy development. In addition, other components in close contact with these barriers, such as the microbiome, have been proven to play key roles in epithelia homeostasis whereas dysbiosis may trigger a tolerance breakdown.
The most common manifestation of allergic responses to food involves the gut, but it is increasingly evident that gastrointestinal allergy to ingested foods often precedes or coexists with respiratory tract symptoms, rhinorrhea, sneezing, coughing, and wheezing. Asthmatics and patients with allergic rhinitis are often affected by gastrointestinal disorders (26). In addition, it has been shown that exposure to aeroallergens (e.g., pollen derived allergens such as profilin) not only have a significant effect on the development of allergic diseases in the lung but can also induce sensitization towards specific foods components (27). The opposite direction has been also shown in mice, as experimental gastrointestinal allergy to egg ovalbumin (OVA) enhances pulmonary responses to OVA but also to an unrelated allergen as house dust mite (28). As an opposite effect, evidence from mice has shown that dietary fiber, metabolized by a “healthy” gut microbiota, exerts a beneficial effect in the immunological environment in the lung protecting against the development of allergy and asthma (29, 30). Intestinal microbiota is of special interest as producer of fiber-derived short-chain fatty acids (SCFAs) that present beneficial effects not only in the gut but also in distant organs. Besides, SCFAs have a powerful anti-inflammatory effect and enhance epithelial function (31, 32). Taking all these considerations into account and in view of the highly interesting crosstalk between the gut microbiota and the lung epithelia in the context of allergy, we focused this review firstly on factors that modulate the integrity of epithelial barriers to focus finally on the role of gut derived SCFAs in the regulation of lung epithelia homeostasis.
Epithelial barriers play a key role in allergy development
The different epithelial barriers of the human body constitute the first line of defense against harming agents, pathogens, or even allergens. Growing evidence supports the “epithelial barrier hypothesis”, which states that epithelial barrier integrity and function is key to maintain the homeostasis of the organism and that a dysfunctional barrier may underlie allergic and other inflammatory diseases and explain their increase in prevalence (33–35).
Even though each epithelia have a different role and morphology, they share many physiological and structural/mechanical features. The apical junction complexes (AJCs) are one of the main components maintaining the integrity of epithelial barriers as they regulate cell-cell adhesion, cell polarity, and paracellular permeability of exogenous elements. These complexes are composed of two main structures, the tight junctions (TJs) and the adherens junctions (AJs) (36–38). TJs are formed by extracellular domains of transmembrane proteins (such as occludin and claudin protein families) that form strong links between them and connect with actin and tubulin cytoskeleton via scaffold proteins such as zonula occludens-1 (ZO-1) (39). TJ formation is regulated by the expression and phosphorylation of their components or the expression of disruptor proteins (e.g., Claudin 2) (40). Allergen tolerance is directly related to TJs integrity: IL-17 and IL-22 induce ZO-1 and claudin expression; but, in atopic individuals, this route is impaired because of the presence of Th2 cytokines facilitating the entrance of allergens (41, 42) (Figure 1).
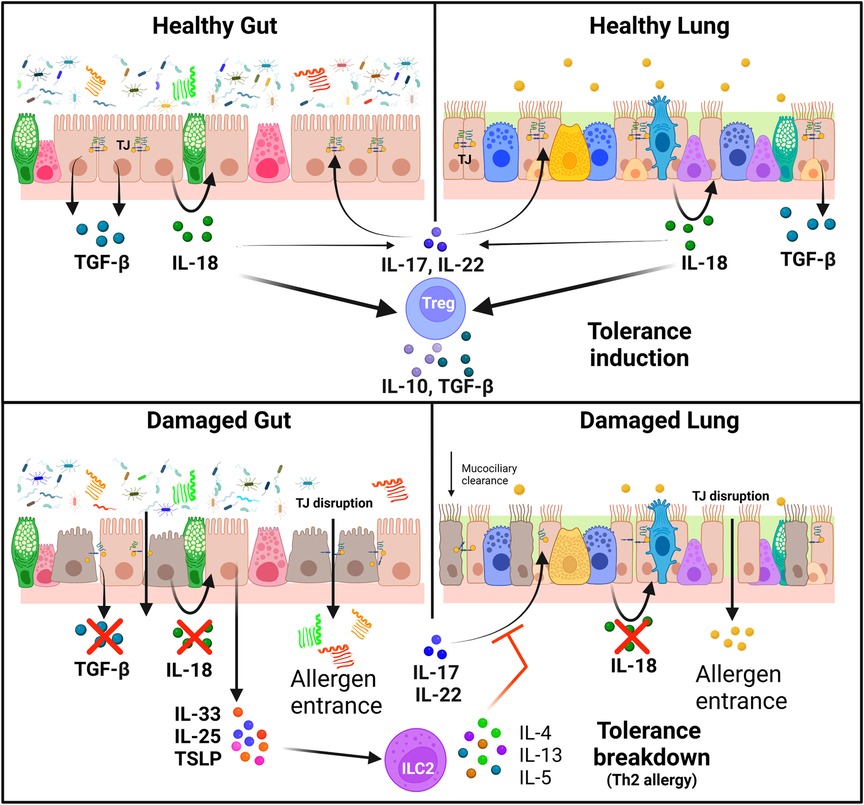
Figure 1. Immunoregulation of tolerance induction and breakdown in healthy and damaged epithelia. Tight junctions are essential for the maintenance of healthy gut and lung epithelial barriers. IL-17 and IL-22 induce the expression of TJ proteins, but this effect is impaired in the presence of Th2 cytokines. Healthy epithelial cells release IL-18 and TGF-β in response to allergens, which enhance the production of IL-17 and IL-22 and stimulate Treg cells to release IL-10 and TGF-β, leading to tolerance induction. On the other hand, epithelial cells in damaged barriers secrete alarmins (IL-25, IL-33 and TSLP) which trigger tolerance breakdown with the activation of a Th2 response in ILC2 cells with the release of IL4, IL-5 and IL-13 (Created with BioRender.com).
Epithelial cells have indeed an immunologic function: they are the first sensors of external damage, producing and secreting alarmins IL-25, IL-33 and thymic stromal lymphopoietin (TSLP), triggering the activation of type 2 innate lymphoid cells (ILC2) Th2 responses (33, 43). This activation is key for sensitization and in the physiopathology of allergic diseases, triggering epithelial damage and an increase in its permeability. ILC2 cells regulate and crosstalk with naïve T cells, showing an important role for specific CD4+ T cell production by secreting IL-5 and IL-13. They participate on IL-4 mediated Th2 differentiation, and promote mast cell sensibility to degranulation, but also inhibit Treg induction (44). They also have a higher cytokine production rate in comparison to Th2 when activated and have the capacity of antigen presentation via MHCII (45).
Thus, these epithelial cell-derived cytokines can be considered as novel targets for allergy treatment (46). Currently, an anti-TSLP monoclonal antibody has been approved in the US for the treatment of uncontrolled severe asthma (47), and an anti-IL-33 is being proved in clinical trials against AD (48) and peanut allergy (49). Moreover, intestinal epithelial cells (IECs) express MHCI/II, thus having the possibility to induce specific antigen immune responses towards allergenic components, and these cells secrete exosomes containing these MHCII-peptide complexes mediating indirect presentation of allergens that prime for an immunogenic rather than tolerogenic response (50, 51).
Healthy epithelia have also a regulatory function: they sense the environment and activate different routes of homeostatic responses via toll-like receptors (TLR), protease-activated receptors (PAR) and nucleotide-binding leucine-rich repeat-containing receptors (NLR) (52–54). The gut epithelia also secrete antimicrobial peptides (AMPs) that play an important role in maintaining tolerance to gut microbiota, protecting against enteric infections, and thus maintaining a healthy microbiome. In addition, some AMPs present anti-inflammatory and immunostimulatory properties. For example, human α/β-defensins and cathelicidin regulate the intestinal microbiota, limiting invasion of the epithelia and acting against gram-positive commensals, restricting enterohemorrhagic E. coli infections; lactoferrin and hepticidin control free iron required for bacterial growth and lysozyme enzymatically degrades the peptidoglycan of gram-positive bacteria inducing their lysis (55, 56).
Inflammatory responses also alter intracellular signaling routes that are intrinsically linked to the maintenance of the barrier integrity (57) (Figure 1). Classic Th2 cytokines IL-4 and IL-13 contribute to TJ instability in skin and the lungs (58). Moreover, IL-4, IL-5, and IL-13 signaling cascades can be triggered after the cell response to barrier-disrupting noxious stimuli (33). In addition, other inflammation-derived molecules, such as IL-6 (57) or histamine (59), are known to induce epithelial barrier permeability.
Environmental factors promote allergic diseases by disrupting epithelial barriers
Western lifestyle, diet, and environment account among the more commonly described factors contributing to the development of the allergic diseases. Humans are daily exposed to a plethora of different chemical and biological agents, overall known as the exposome (60). Human exposome includes compounds related with pollution (61, 62), hygiene-derived products such as laundry detergents (63), house dust mites (64), natural toxins (e.g., the mycotoxin deoxynivanelol) (65), and food additives (e.g., food emulsifiers) (66), which are mostly harmful for highly exposed body cell surfaces like the epithelial barriers. Several in vitro studies show that when the human epithelial barrier is exposed to these compounds, cell functions are altered (67, 68), and allergenic responses could be enhanced (69–74). Moreover, an adverse outcome pathway (AOP) describing the covalent binding of electrophilic chemicals to keratinocyte proteins leading to skin sensitization has been described (https://aopwiki.org/aops/40). This AOP is quite interesting as the covalent binding of electrophiles could potentially affect proteins from epithelial cells form other organs triggering their activation, leading to further activation of dendritic cells and T-cells and sensitization towards these electrophilic compounds.
Diverse environmental proteases have been described as allergenic initiators triggering further immune responses. Among them, it is important to mention those from house mite feces (whose presence in the exposome is directly related with western lifestyle), such as Der p 1 (75), and fungal proteases, such as Asp f 13 (76). The activity of these proteases disrupts the human TJs and increases the epithelial barrier permeability, facilitating the passage of diverse allergens according to in vitro studies (74, 77), and the stimulation of the innate immune system (78, 79). Their effect is regulated by the redox microenvironment of the bronchial lumen, as it has been described that Der p 1 activity is enhanced by glutathione-S-transferase-pi, a detox enzyme secreted by the bronchial epithelium, and by the presence of the antioxidant glutathione, both of which are highly abundant in the human epithelial lung fluid (80). This effect is stronger on damaged or inflamed epithelium (as occurs in asthmatic patients), in which anti-protease and mucociliary clearance are impaired (81). Protease inhalation is also related to FA, as a higher response to food allergens and an associated gut epithelium disfunction has been described in mice after intranasal exposure to dust mite extracts (82).
Environmental proteases as Der p 1 not only affect human bronchial epithelium, but also alter the integrity of intestinal epithelium as they are present in the diet together with food derived allergens with protease activity (e.g., Act d 1, a Cys-protease from kiwifruit) (83, 84). Der p 1 has been detected on human intestinal biopsies, where it not only disrupts the epithelial barrier integrity but also reduces the expression of TJ proteins and mucus barrier and induces a pro-inflammatory response with increased cytokine release (85, 86). Apart from barrier disruption, it is worth mentioning that alternative mechanisms could be acting at promoting allergic sensitization triggered by airborne proteases. In this sense, and although much less explored, Der p 1 cleavage of other secondary allergens have been described to generate small and more permeable allergen-derived peptides, which indeed preserve IgG/IgE reactivity and activation of basophils from allergic patients (77).
Several other exposome components of the urban environment produce or synergistically enhance epithelial damage, as has been shown in asthma and allergic rhinitis patients (87). On the inhaled group of toxic agents, it is important to mention traffic and industry related contamination (NO2, O3, particles in suspension), whose levels have been related geographically with a higher rate of infant asthma (88). Maternal exposure to NO2 causes an impairment in Th1/Th2 balance in newborn mice, leading to enhanced sensitivity to allergens and increased airway hyperresponsiveness (89). Increased Th2 response and accumulation of ILC2 cells was observed in a diesel exhaust-enhanced allergic mice model (90). Ozone and NO2 have, as well, a pro-inflammatory effect upon bronchial epithelial cells, promoting the release of cytokines and chemokines, such as IL-33, IL-25 and TSLP, in both normal and asthmatic patients (91, 92).
Tobacco smoke exposure also exacerbates asthma and rhinitis symptoms and decreases muco-ciliary clearance (93). Moreover, there is a strong epidemiological link between pre- or postnatal passive cigarette smoke exposure and the prevalence of asthma in children (94, 95). Recent studies have spotlighted the effect of cigarette smoke on the pulmonary epithelium, directly disrupting TJs and modulating TJ protein expression and aggravating OVA-induced inflammation in asthmatic mice models (96).
Cigarette smoke exposure also modifies epigenetic marks involved in immune response, such as increasing methylation of GC isles in genes like IL-10 in human samples (97) and promote Th2 responses, e.g., by decreasing gene methylation of IL-4, IL-13 or increasing FOXP3 methylation after house dust mite challenge (44).
Other components from the exposome can also alter the pulmonary epithelium and facilitate the induction of respiratory allergic diseases. On this idea, it has been reported that inhalation of airborne microplastics causes pulmonary inflammatory cell infiltration, bronchoalveolar macrophage aggregation and increased levels of tumor necrosis factor-α (TNF-α) in both healthy and asthmatic mice (98), and that viral infections during infancy and childhood predispose to later asthma development (99, 100).
Diet modulates epithelial integrity: protective and harmful food factors
Perinatal and early age nutrition, and the correct time of active introduction of dietary components is key to avoid FAs and the development of tolerance. The actual tendency is to potentiate this development by introducing probiotics and lactose in child formulas (101). As described above, a damaged gut epithelium is related to the development not only of FAs but also of other types of allergies. In fact, there is increasing evidence of the presence of ingested noxious agents in the exposome that contribute to this damage. During the early life introduction of different foods, especially when breastfeeding is being retired (or has been absent), the child is lacking the protective compounds supplied by mother's milk. In this context, some foods and dietary components, especially from fish and vegetable meals, have been proven to possess a protective effect against gut epithelium damage and even in distant epithelia such as the lung, either directly or after intestinal microbiome processing (102–104).
Even though some diet components and contaminants may alter the epithelial barriers, there are several other diet components that present protective effects against allergic diseases by reinforcing the epithelial barrier (Figure 2). EAACI 2020 guidelines point towards the beneficial effect of breastfeeding by preventing the development of food allergy in the first two years of life, or even against asthma and allergic rhinitis (105, 106). Maternal milk contains a milieu of beneficial components; however, their levels vary between individuals, especially when comparing atopic mothers with non-atopic mothers. Maternal secreted IgA is directly able to raise the infant immune system as it protects from respiratory and digestive tract infections, thus preserving epithelial integrity. Lower levels of IgA in milk are related to higher risk of cow milk allergy (107). Soluble CD14, that is produced by mammary epithelial cells, is another powerful immunomodulatory molecule; it mediates secretion of innate immune response molecules such as IL-8, TNF-α, and epithelial neutrophil activator-78 by CD14-negative intestinal epithelial cells exposed to lipopolysaccharide (LPS) or bacteria (108). CD14 acts as a co-receptor for TLR4 and enhances the recognition of bacterial LPS by the immune system, especially at low LPS levels (109). This CD14-TLR4 interaction plays a key role in immune tolerance development during early life, as evidenced in the correlation between low CD14 levels in breast milk with higher risk of AD (110) and sensitization to egg white (111). Breast milk also contains TGF-β, a powerful anti-inflammatory cytokine that is also involved in gut epithelial integrity, IL-10, an inducer of antigen-specific tolerogenic Treg and Breg cells, and IL-6, a pro-inflammatory cytokine that stimulates Th-17 responses in the presence of IL-10 and TGF-β (112, 113). These molecules are absent or undetectable in substitutive formula for breastmilk.
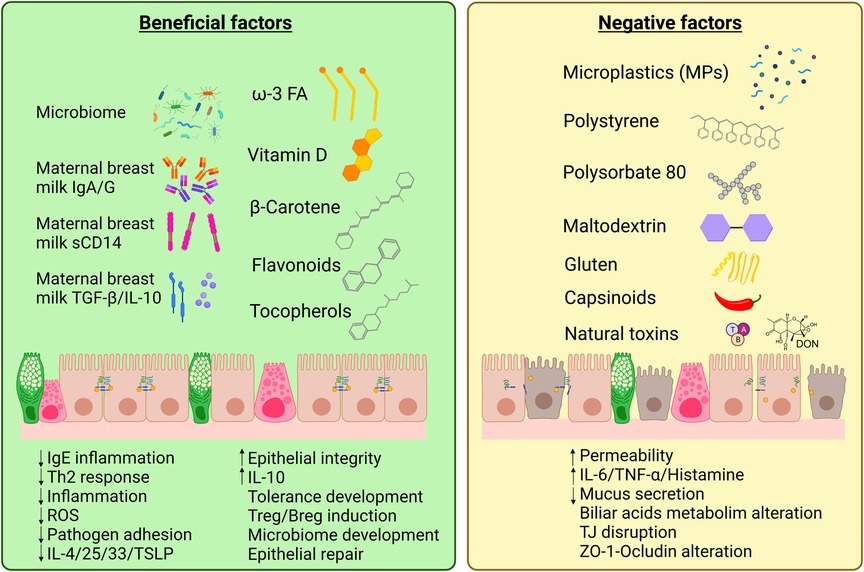
Figure 2. Diet components that present beneficial or negative effects on the intestinal epithelial barrier. An equilibrated diet and maternal breast milk contains a wide variety of beneficial factors that enhance intestinal epithelium integrity and repair. However, western lifestyle and pollution have introduced new agents in our diet that, together with natural compounds as gluten, capsinoids or natural toxins [e.g., mycotoxins as deoxynivanelol (DON)], can be irritant or present negative effects on gut epithelial cells increasing barrier permeability and potentially triggering allergic responses (Created with BioRender.com).
Early sensitization to food allergens during the breastfeeding, or even in the amniotic fluid, explain reactions to a food with which there has been no previous contact. In fact, the presence of major food allergens in breast milk and amniotic fluid has been shown in the range of nanograms per milliliter, as described for OVA, β-lactoglobulin from cow and sheep's milk, peanut proteins Ara h 1/2/6, among others from fruit, mustard, wheat or fish (114–116). The kinetics of antigen shedding and clearance strongly depend on the mother's diet, but varies with individuals, the way of antigen consumption (i.e., raw or cooked), or with the interaction of other milk components (115). Specific IgG from an allergen-exposed non-atopic mother is related to the onset of antigen-specific tolerance in the infant due to the formation of IgG-antigen immune complexes in breast milk that potentiate specific Treg cell development (117, 118).
Human breast milk also contains a lipid fraction, which is responsible for many beneficial properties. Special attention has been paid to polyunsaturated fatty acids (PUFAs), mainly ω-3 fatty acids like eicosapentanoic (EPA) and docosahexanoic (DHA) acids, that depend directly on the mother's diet (e.g., fish oil supplementation) and are currently under study due to the correlation of breast milk lipids and the risk of allergic disease (119, 120). Oligosaccharides from breast milk are additional agents that potentiate epithelial barrier function and protect against pathogen adhesion. Moreover, they contribute to the early life microbiota development (as will be discussed later in this review) (121).
In addition to their presence in breast milk, ω-3 long-chain PUFAs (DHA and EPA) are present in various food sources and can be incorporated in the routine diet. As described above, they play a key role in immune system development and in the establishment of tolerance by suppressing inflammation (122). These two PUFAs, together with ω-6 arachidonic acid (AA), are widely present in immune cell membranes. Free AA is oxidated via cyclooxygenase (COX) and lipoxygenase (LOX) pathways, releasing prostaglandins and leukotrienes. The role of AA-derived metabolites in allergic disease is still controversial. In one hand, they enhance inflammation, histamine production, white cell infiltration in epithelia and suppress Th1 inflammation; on the other hand, prostaglandin E2 is reduced in severe anaphylactic patients' sera (123). DHA and EPA suppress AA-mediated inflammation as their oxidation produces anti-inflammatory molecules. In fact, both PUFAs from fish oil supplements have been reported to ameliorate allergic responses in children with risk of developing allergy (124). Novel roles for these acids have been also described in in vitro porcine and human models, as they interact with PPAR-α and PPAR-γ in intestinal epithelial cells, enhancing epithelial cell function (125), protecting against exogenous damage (126) and decreasing cytokine-mediated permeability (127). High DHA diet al.so enhances repair of dust-exposed pulmonary epithelium in mice (128).
Vitamin D is a fat-soluble sterol whose deficiency has been related to immune deficiencies and is also involved in the regulation of gut microbiota. Humans can synthesize it after sunlight exposure (which is reduced by western lifestyle) or obtain it from animal- and plant-derived food. Vitamin D contributes to intestinal homeostasis inducing the expression of the antimicrobial peptide cathelicidin in IECs (129) and is essential to maintain the integrity of the gut mucosal barrier by enhancement of intercellular junctions that control mucosal permeability and reduction of pro-inflammatory cytokines such as IL-8; therefore, its deficiency is related to a leaky gut (130, 131). It also has a potent tolerogenic effect towards the immune system, inducing IL-10 secretion and contributing to Treg differentiation (132).
Nevertheless, there are discrepancies as to the potential benefits of supplementing diet with vitamin D regarding the prevention of allergic diseases; these beneficial effects are not clear in FAs and AD (133) and only some studies detect a decrease in the incidence of asthma and rhinitis (134). Prenatal administration to pregnant woman with asthma risky child induces a slight descent on the 3-year incidence of asthma and rhinitis, but did not influence the 6-year incidence (135). Vitamin D supplementation, combined with immunotherapy, enhances FoxP3 expression on specific therapy towards AD according to human in vivo studies (136); however, no symptomatologic differences were detected compared to patients treated with immunotherapy alone. On a cohort of Icelander children that received (or not) vitamin D supplementation, this administration correlated with a 6-year lower risk of allergic sensitization (137). But vitamin D administration is inefficient on asthmatic patients in which the vitamin blood levels are already low (138). This may correlate with an increase in oxidative stress in the gut due to pollutants and increased luminal levels of oxidized vitamin D metabolites that do not bind to the vitamin D receptors (139). Low vitamin D blood levels can be also due to malabsorption of the vitamin, as has been reported in several cases of food allergies (140). Thus, vitamin D diet supplementation may not be effective in these patients. In summary, authors conclude that enough vitamin D administration is key for tolerance development (specially on Nordic countries with limited sunlight), but the beneficial effect is still controversial.
Dietary antioxidants have been associated with a protective effect against allergic diseases (141). Diverse vitamins and vegetable-derived compounds have been proven to exert beneficial effects on human health, given the fact that oxidative stress impairs the gut metabolism of Vitamin D (139). Liposoluble vitamins β-carotene (pro-vitamin A) and tocopherol (vitamin E), obtained only from fresh vegetables and nuts, have also proven their protective effects against allergy due to their strong antioxidant properties. Vitamin E impairs Th2 inflammation on mice models, inhibiting eosinophil and neutrophil activation and the production of oxygen reactive species (142). Intranasal administration of tocopherol is a current approach to ameliorate symptoms in allergic rhinitis patients, but oral supplementation is not proven to be efficient in AD, FA or asthma treatment (143).
Plant flavonoids like kaempferol or quercetin are also known as inflammation suppressors with increasing relevance in the field of allergy. Both reduce IgE-induced inflammation in human IECs (144) and kaempferol mitigates inflammation in asthma models when orally administrated to OVA-sensitized mice (145). In vivo studies showed that quercetin ameliorates mice epithelial asthmatic response inhibiting the secretion of IL-4, IL-25, IL-33 and TSLP and leads to lower mast cell infiltration and endothelial smooth muscle thickness (146).
Diet can also contain harmful components or additives that may impair the intestinal epithelial barrier function and thus foster inflammatory diseases, such as inflammatory bowel disease, obesity and celiac disease (33, 147), as well as FA, where the allergens can cross the leaky epithelial barrier and reach immune cells (148) (Figure 2). Along with lifestyle changes and pollution increase, dietary changes have occurred specially in urban environments, with reduced fresh food intake and increased processed and junk foods.
Food additives, mainly dietary surfactants, are on the focus due to their damaging properties for the epithelial barrier showing toxic and gut permeabilizing effects (149). For example, the food emulsifier Polysorbate-80 has been reported to increase the permeability to food allergens in rodents and human IECs (150–152), as well as presenting effects on distant tissues, being able to alter the systemic metabolism leading to glucose intolerance and mitochondrial dysfunction in the skeletal muscle in mice (153). Maltodextrin, an alimentary thickener widely used in infant formula, induces reticular stress, inflammation, and mucin deficit on the intestinal epithelium (154). In addition to food additives, other diet components can also alter the epithelial barrier status. For example, gliadin can alter the interactions between occludins and ZO-1 on humans (155), or hot spices components (such as capsianosides and terpene glycosides) alter TJ integrity and the paracellular flux by affecting the actin cytoskeleton (156, 157).
Because of environmental contamination, and due to the high recalcitrance of many synthetic plastics that result in their long persistence in the environment, small plastic particles (microplastics or MPs) have been incorporated to our diets. These MPs accumulate in the marine ecosystem, being ingested by invertebrates and fish, and can also leak into soil and accumulate in plants, thus entering the trophic chain being an increasing matter of concern in human health (158, 159). As an example, polystyrene MP have been shown to affect directly epithelial permeability and alter biliary acid metabolism in mice (160). Other studies have related the ingestion of these MPs with mucus secretion, glucose and lipid metabolism, and microbiota alterations (161). Furthermore, polypropylene MPs have been associated with immune system alterations (162), inducing an increase in the secretion of pro-inflammatory cytokines (e.g., IL-6 and TNF-α) and histamine in human and mice cells in vitro (163). Interestingly, most of the damage caused by MPs on the intestinal barrier seem to be more directly dependent on the particle size and not so much on their composition (163, 164). Altogether, these data point towards a potential contribution of MPs to allergic diseases development due to the deleterious effect on the gut epithelium and their pro-inflammatory potential (68, 165); nevertheless, further research is required to clarify MP effects in human health.
Gut microbiome as a key regulator of the intestinal epithelial barrier status and immune response
It is increasingly evident that dysbiosis of the human commensal gut bacteria triggered by western lifestyle may contribute to food allergy (166–169). In vitro/ex vivo studies showed that gut microbiome is composed not only by bacteria, but also by other microorganisms including fungi, archaea, virus and protozoans (170). Among healthy individuals, the microbiota composition is quite similar, despite minor variations based on own individual diet and lifestyle (Figure 3). The main bacterial phyla present in the human healthy gut are Firmicutes (classes Clostridia and Lactobacillus), Bacteroidetes (class Bacteroidales), Actinobacteria (class Bifidobacteriaceae), and Pseudomonadota/Proteobacteria (class Enterobacterales) (Figure 3) (171). However, important differences in microbiome species have been described in diseases such as inflammatory bowel disease (37, 170), celiac disease (172) or food allergies (173, 174), among others.
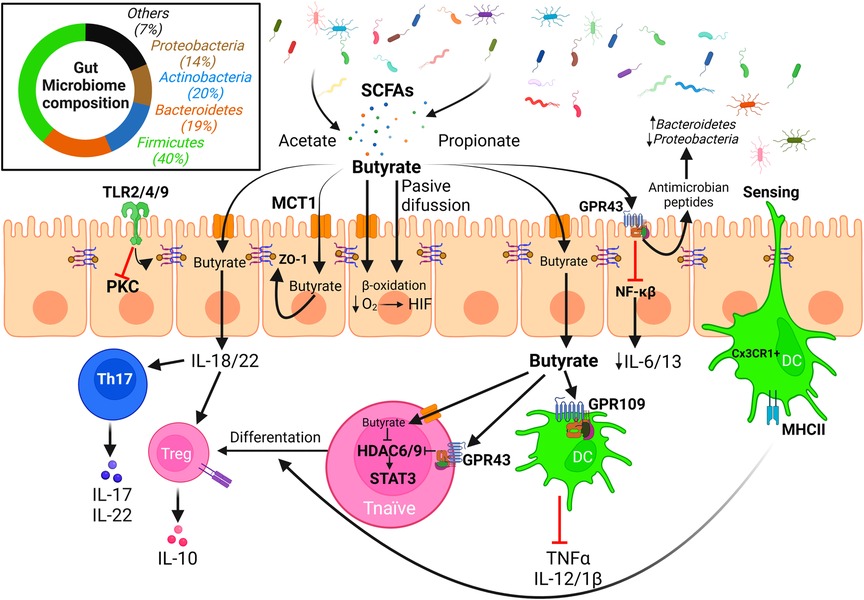
Figure 3. Effect of microbiota derived SCFAs on the integrity of the intestinal epithelial barrier. The intestinal microbiota is mainly composed of bacteria from the phyla Firmicutes/Bacillota, Actinobacteria, Bacteroidetes, and Proteobacteria/Pseudomonadota among other minor phyla. Diet fiber fermentation by gut microbiota generates SCFAs that may enter the epithelial cells either through passive diffusion or via monocarboxylate transporters, or well interact with GPCRs (e.g., GPR43) present in the apical membrane. Butyrate is the main fuel and carbon source for colonocytes, but it can be exported through the basolateral membranes and interact with immune cells altering T cells differentiation and cytokine release patterns, promoting the integrity of the epithelial barrier. Biological effects of butyrate are mainly related to its activity as inhibitor of HDACs, effect that is enhanced via interaction with GPR43 that induces a down-regulation of HDAC expression (Created with BioRender.com).
The development and maintenance of a healthy gut microbiome is key to human health status as it contributes to many physiological functions: it impairs pathogen colonization, promotes gut epithelial and mucosal integrity, produces necessary vitamins B12 and K, and has a potent immunomodulatory role (175). Even though the question of what a healthy microbiome is remains unsolved, some species have been related to human microbial health, specifically with allergic diseases. Bacteroides fragillis and Bacteroides stercosis are specially relevant species, even though they are only moderately abundant in the human gut, as they modulate the production of key metabolites that influence the composition of the microbiota (176). The biodiversity hypothesis states that microbiota species enrichment promotes immune tolerance, whereas low microbiome species diversity is associated with allergic diseases (177). However, the increase in certain bacterial populations, such as Clostridium or Firmicutes, are associated with an enhanced allergic sensitization risk in young children according to various cohort studies (178–180).
Homeostatic and beneficial properties of commensal bacteria are mediated by small metabolites that can cross epithelial barriers and exert their effects locally or in distant epithelia as the lung or the skin after entering the bloodstream, inducing cytokine release. Short-chain fatty acids (SCFAs), particularly butyrate, propionate and acetate, are among the main products of dietary fiber fermentation, with a total concentration in the human intestinal lumen decreasing from 70 to 140 mM in the proximal colon to 20–70 mM in the distant colon (181). These SCFAs are key players in the microbiome-immune system crosstalk (Figure 3) (182). They present beneficial effects regarding FAs; among them, they modulate gut epithelial cell function and barrier integrity (31, 32).
Butyrate is used as a preferential carbon and energy source by human IECs, being consumed by the β-oxidative pathway and shifting energy metabolism (183). Butyrate metabolism decreases available intracellular O2, leading to hypoxia induced factor (HIF) activation, which enhances gut integrity and is necessary for butyrate-mediated intestinal barrier restoration (184, 185). SCFAs are recognized by specific G protein-coupled receptors (GPCRs), such as GPR109 or GPR43, present on the surface of intestinal and immune cells. This interaction generates anti-sensitization responses on the epithelial barrier and induce Treg cell differentiation in the colon (186–189). Depletion of these receptors provoke a disruption of the gut epithelial barrier on mouse models, increasing allergen permeability and inducing specific IgE reactions (190). GPR43 is expressed on a higher rate on epithelial cells rather than dendritic cells (DCs), whose main receptor is GPR109. GPR43 activation in IECs induces antimicrobial peptide production, which regulates microbiota composition by enhancing Bacteroidetes population and decreasing Proteobacteria proportion (191). GPR43−/− mice had this effect reversed when butyrate was added to drinking water, accompanied by an increase of Clostridium and Proteobacteria (190, 191). GPR43 has a maximal effective concentration threshold in human epithelial cells of around 0.5 mM, and its activation triggers downstream signaling that provokes cAMP decrease and increases cytoplasmic calcium concentration (192). This receptor induces also an alternative anti-inflammatory signaling mediated by β-arrestin-2, by inhibition of the NF-κB pathway, and down-regulation of pro-inflammatory cytokines IL-6 and IL-1β (193).
In vivo experiments revealed that high fiber diets, that increase butyrate production in the gut, enhance the expression of cellular adhesion proteins such as occludin and ZO-1, and induce IL-22 expression, thus contributing to the integrity of the gut mucosal barrier while reducing the concentration of pro-inflammatory IL-21 (189). SCFAs, mainly butyrate, induce the production of IL-18 in IECs (194), an additional key cytokine for the maintenance of barrier homeostasis (195). TSLP, that polarizes dendritic cells (DCs) to adopt a CD103-(+) Th2 phenotype, is enhanced in GPR43−/− mice, but paradoxically not altered when GPR109 is absent (190). In addition, SCFAs restore Treg numbers in mice devoid of a gut microbiota and regulate Treg function via GPR43 inducing a reduced expression of histones deacetylases (HDACs) 6 and 9 (196).
Bacterial-derived SFCAs, mainly butyrate, also regulate the epigenetic status of the human epithelium by inhibition of HDAC activities, specifically zinc-dependent class Ia and IIa HDACs (197). Butyrate enters epithelial and immune cells via passive diffusion or through different monocarboxylate transporters as MCT1 or a butyrate/bicarbonate antiporter (183). This leads to HDAC inhibition (together with the above mentioned down-regulation of the expression of some HDACs via GPR43), causing an opening of the chromatin and facilitating the binding of STAT3, NF-κB or Foxp3, important factors on the development and regulation of immune cells (193). In vitro studies demonstrated that this provokes a repressed production of pro-inflammatory molecules TNFα, IL-12, IL-1β, and NO on immune cells, while upregulating anti-inflammatory IL-10 production on mononuclear cells and neutrophils (193). Once butyrate enters the cell, it also has the capability of activating PPAR-γ (198), promoting epithelial barrier integrity (199).
Other SCFAs such as acetate and propionate have also some effects on immune responses, increasing the expression of IFN-β in the lungs via GPR43 activation (200) or inducing CD69 expression on basophils and IL-13 production (201), but their role in allergy seems to be less relevant than that of butyrate.
The microbiome, either through its metabolites or through direct interaction with cells, influences immune responses, with the epithelial barrier as central element of transduction. SCFAs induce different cytokine secretion by gut epithelia, as we have previously reviewed, which modulate the immune system response. Human IECs express on their surface TLR2/4/9, that can recognize exogenous elements present on the lumen. Activation of TLR2 enhances ZO-1 expression in human IECs via activation of PKC, whereas TLR4 activation reduces this expression (202). IECs can also produce and secrete several types of cytokines under different stimuli. For example, butyrate induces in vivo IL-18 secretion by mice IECs modulating Th17 and Treg cells, controlling their differentiation (203). In response to allergen exposure, IECs can produce IL-25, IL-33 and TSLP and signal to ILC2 cells, that respond secreting Th2 cytokines (IL-4, IL5, IL-13), and IL-9 (204–206). These cytokines have the ability of inducing differentiation of progenitor cells to secretory cells, perpetuating allergic inflammation (207). Together with IL-9, IECs can also secrete eotaxin-1 that recruit eosinophils to the gut when an allergen is present (208). Otherwise, IL-22 secreted from immune cells, such as Th1/17, affects the state of the epithelial barrier, inducing cell survival via secretion of antimicrobial peptides from the Reg family (RegIIIβ and RegIIIγ) in colonic epithelial cells (209).
The immune system has also mechanisms to directly sense the microbiome and antigens which are present in the lumen. CX3CR1+ DCs generate protrusions that reach the lumen, crossing epithelial cell contacts, and capture antigens directly without epithelial processing preserving the integrity of the epithelial barrier (210, 211). These DCs express CD11c and CD103, via TGF-β and retinoic acid produced by IECs, promote Treg differentiation (212), and induce integrin α4β7 expression which is involved in gut homing of Treg cells (213, 214).
Microbial and dietary regulation of the pulmonary epithelium: the gut-lung axis
Despite their manifest anatomical distance, alteration of the human microbiome resident in the gut has been linked to inflammatory conditions on the airways mainly via SCFAs (acetate, propionate and butyrate) derived from bacterial fermentation of diet fiber (215), contributing to the definition of a novel player in allergic diseases: the gut-lung axis (216). SCFAs and small bacterial metabolites have a positive effect as well in different epithelia along the body, contributing significantly to the skin barrier function (defining the gut-skin axis) by modulating keratinocyte metabolism in fiber-fed mice models (217) and even to the esophageal barrier function in vitro (218).
There is increasing evidence of the influence of intestinal microbiota metabolites in the maintenance of lung epithelium homeostasis and integrity. Cohort studies have shown a systematic decrease in SCFA-producing Bifidobacteria in long-term asthmatic patients, spotlighting the relevance of Bifidobacterium adolescentis and Bifidobacterium breve as inflammation suppressors (219, 220). In a similar way, a decrease of Akkermnansia and Faecalibacteria species in the human gut lumen has been related in vivo to the development of asthma and atopy in parallel with an alteration on the fiber fermentation and SCFAs production (221). These results are also supported by the European PASTURE/EFRAIM study group, that has reported that high concentrations of fecal butyrate and propionate in children at the age of one year had significantly less atopic sensitization and were less susceptible to develop asthma between 3 and 6 years, together with a reduced risk of food allergy and allergic rhinitis (222). SCFAs have a protective role against allergic sensitization in the lungs, as they are able to downregulate hematopoiesis during Th2 allergic airway inflammation (30), enhancing macrophage and DC progenitors, that later infiltrate in the lungs and mature to CD11b+ DCs which are unable to present allergens (223, 224). In vivo studies with butyrate oral administration demonstrated an attenuation of OVA-induced asthma and lung infiltration of eosinophils into lungs on mice (222). Similar results were observed with high fiber fed mice challenged with house-dust mite (29).
On the contrary, some bacterial species from the genus Clostridium have been typically associated with pro-inflammatory roles (e.g., pathogenic Clostridia or Clostridium neonatale) and they are increased in stool samples from asthmatic children related to controls (225). However, Clostridium butyricum has been recently associated with tolerance development (226).
Even though SCFAs play a key role in tolerance to aeroallergens and pulmonary inflammatory status, lung microbiome cannot produce them, being specifically secreted by some populations of the gut microbiome establishing a unique relationship between diet, gut microbiome, and respiratory allergy. Microbiome-produced or diet-derived butyrate enters the bloodstream crossing the intestinal epithelial barrier via apical surface MCT1 or SMCT1 transporter proteins in the epithelial cells (227); although butyrate is used by colonocytes as main energy and carbon source, it can still be exported through the basolateral membranes via MCT3–5 (228) reaching the bloodstream (Figure 4). Propionate and butyrate are metabolized in the liver, but they can still reach peripheral organs, such as the lungs or the bone marrow, where concentration of these SCFAs is directly correlated with fiber consumption (229). High-fiber diet is known to cause a 20-fold increase in butyrate concentration in portal blood and a 6-fold increase in arterial blood in porcine models (230), and 100-fold in venous blood in murine models (231). Butyrate and propionate concentration in human plasma is around 1–10 μM (229); however, there are no clear studies on the effect of diet or microbiota in SCFA concentrations in human peripheral blood due to limited accessibility of blood samples and the requirement of complex quantification techniques, such as mass spectrometry or heavy isotope labeling. In any case, new and simpler methods for determining SCFAs in human blood are currently under development with potential application in this field (232).
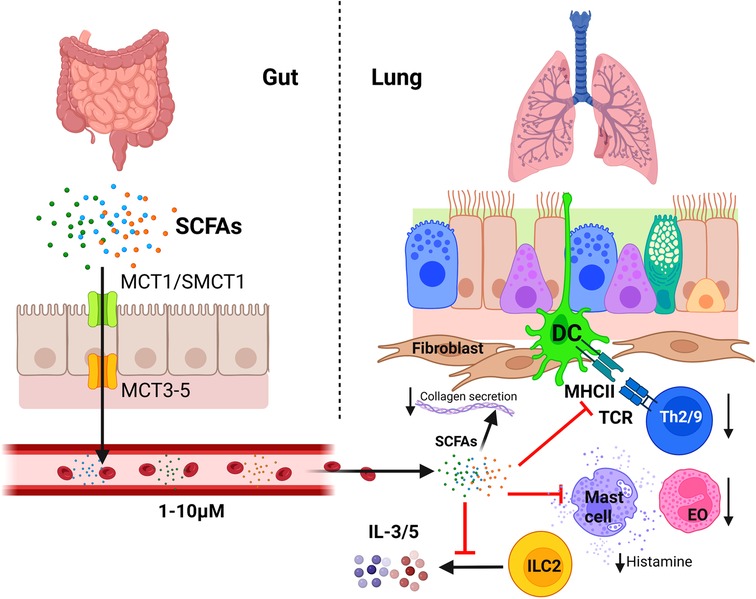
Figure 4. Regulatory influence of the gastrointestinal microbiota on the immunology of the lung: the gut-lung axis. Butyrate, propionate, and acetate produced by gut microbiota cross the intestinal epithelial barrier through monocarboxylate transporters and reach distant organs through the bloodstream and the lymphatic circulatory system. In the lung, SCFAs affect the bronchial-associated lymphoid tissue acting as efficient suppressors of the allergic immune reaction. Among other effects, they induce an inhibition of Th2 cell activation and induce Treg cell differentiation, inhibit IL-3 and IL-5 secretion by ILC2 cells and the migration of eosinophils (EO) to airways, induces eosinophils apoptosis, and inhibits mast cell degranulation. In addition, SCFAs can also reduce allergic bronchoconstriction and pulmonary fibrosis by inducing a down-regulation of collagen synthesis by lung fibroblasts (Created with BioRender.com).
Among SCFAs, butyrate is the most efficient suppressor of the allergic immune reaction in the lung. This fatty acid inhibits Th2 and Th9 cell activation (233, 234) and induces Treg cell differentiation (235). It also impedes IgE class switching in B cells (236), mast cell degranulation after FcɛRI activation (237), IL3 and IL-5 secretion by ILC2 cells (238), eosinophilic migration to airways and its chemotaxis, and induces eosinophils apoptosis (239) (Figure 4).
Recent studies using asthmatic murine models have spotlighted the effects of the diet on allergic sensitization and asthma exacerbations. Mice fed with high-fiber diet showed similar effects on allergic inflammation suppression compared to those who were fed with propionate in drinking water (231). Butyrate, but not acetate and propionate, was able to impair infiltration of white cells, particularly eosinophils, and increased FOXP3+ cell percentage in the lung, whereas the three SCFAs reduced the reaction after methacholine challenge in an OVA-sensitized mice asthma model (222). High-fiber diet and orally administered acetate suppressed airway inflammation in house dust mite-sensitized mice, inducing tolerance-related M2 macrophage polarization (which produced less IL-4, IL-5 and IL-13 after intranasal challenge) by inhibiting HDAC9, promoting acetylation on Foxp3 promoter (240). Butyrate has also a direct effect on the lung epithelium, reducing the airway remodeling-related collagen deposition on bronchioles in OVA-sensitized mice by impairing expression of matrix metalloproteases 2 and 9, and reduces allergic bronchoconstriction and pulmonary fibrosis by reducing collagen synthesis by lung fibroblasts (241, 242). Butyrate and propionate can repair human lung epithelial damage after protease damage, IL-4, or IL-13 stimulation, but only butyrate reduces IL-6 production by epithelial cells (243).
Even though there is a great number of reports on the effects of gut microbiota-produced and diet SCFAs on the lung epithelium, the effective concentration that reaches the lungs, the inflammation suppression mechanisms, and the effects of novel players in the gut-lung axis are still a matter of study with a therapeutical and preventive perspective, defining gut microbiota as a potential target for new and complementary treatments for asthma and allergic rhinitis.
Concluding statements
Allergic diseases are exacerbated immunological processes that affect more and more people worldwide, making the development of adequate therapeutic strategies increasingly necessary. Its symptoms are very varied and depend on the characteristics of the individual and include chronic processes such as asthma, atopic dermatitis, rhinitis, and food allergy.
Despite being widely described as an exacerbated Th2 process, several authors coincide on the relevant role played by the epithelial barrier in the allergic process. Epithelial cells form a defensive barrier that, in response to different environmental stimuli, are capable to orchestrate the underlying immune response through secretion of cytokines and chemokines. The epithelial barrier, either from the airways or intestinal compartments, constitutes the first line of cellular defense exposed not only to allergens, but also to various harmful environmental substances. These substances include pollutants and airborne proteases (such as those present in the dust) or, in the case of diet, detergents, emulsifiers and other food additives, conditioning the integrity and correct function of the epithelial barrier. Several in vitro/in vivo studies have already related the loss of the integrity of the epithelial barrier to the chronic development of allergic diseases, either due to the action of these agents or due to genetic defects in the junctional proteins keeping the integrity of the barrier. On the contrary, various components of the diet, such as PUFAs, liposoluble vitamins, as well as flavonoids, seem to compensate the deleterious effect of food additives, promoting a correct epithelial function and contributing to reduce the characteristic inflammation of food allergic reactions.
The integrity of the lung and intestinal epithelial barriers is strongly dependent on their respective microbial populations. Alterations in the composition of microbiomes may strongly affect the correct function of these barriers contributing to the appearance of allergic diseases. In this context, we have highlighted the role of a high fiber diet as precursor of intestinal microbiome generation of SCFAs and their importance not only in the intestinal homeostasis but also in anatomically distant environments, with special reference to the gut-lung axis.
Author contributions
MV formulated the outline of the review. JPB, RGG and JT wrote the initial version of the manuscript. JCLR, NO, MV, EB and JT revised and contributed to the article. All authors contributed to the article and approved the submitted version.
Funding
This work was supported by grants PID2020-116692RB-I00 from the Ministerio de Ciencia y Educación and cofunded by FEDER/EU (MV & EB), and Co-operative Research Centres: ARADyAL RD16/0006/0014 (MV). JPB is supported by an UCM-Santander predoctoral contract.
Conflict of interest
The authors declare that the research was conducted in the absence of any commercial or financial relationships that could be construed as a potential conflict of interest.
Publisher's note
All claims expressed in this article are solely those of the authors and do not necessarily represent those of their affiliated organizations, or those of the publisher, the editors and the reviewers. Any product that may be evaluated in this article, or claim that may be made by its manufacturer, is not guaranteed or endorsed by the publisher.
References
1. Goodman RE, Chapman MD, Slater JE. The allergen: sources, extracts, and molecules for diagnosis of allergic disease. J Allergy Clin Immunol Pract. (2020) 8(8):2506–14. doi: 10.1016/j.jaip.2020.06.043
2. Bercedo Sanz A, Martínez-Torres A, González Díaz C, López-Silvarrey Varela Á, Pellegrini Belinchón FJ, Aguinaga-Ontoso I, et al. Prevalence and temporal evolution of asthma symptoms in Spain. Global asthma network (GAN) study. An Pediatr (Engl Ed). (2022) 97(3):161–71. doi: 10.1016/j.anpede.2021.10.005
3. Hisinger-Molkanen H, Pallasaho P, Haahtela T, Lindqvist A, Sovijarvi A, Piirila P. The increase of asthma prevalence has levelled off and symptoms decreased in adults during 20 years from 1996 to 2016 in Helsinki, Finland. Respir Med. (2019) 155:121–6. doi: 10.1016/j.rmed.2019.07.014
4. Selroos O, Kupczyk M, Kuna P, Lacwik P, Bousquet J, Brennan D, et al. National and regional asthma programmes in Europe. Eur Respir Rev. (2015) 24(137):474–83. doi: 10.1183/16000617.00008114
5. Turner PJ, Gowland MH, Sharma V, Ierodiakonou D, Harper N, Garcez T, et al. Increase in anaphylaxis-related hospitalizations but no increase in fatalities: an analysis of United Kingdom national anaphylaxis data, 1992–2012. J Allergy Clin Immunol. (2015) 135(4):956–63. doi: 10.1016/j.jaci.2014.10.021
6. Reddel HK, Bacharier LB, Bateman ED, Brightling CE, Brusselle GG, Buhl R, et al. Global initiative for asthma strategy 2021: executive summary and rationale for key changes. Eur Respir J. (2022) 59(1):2102730. doi: 10.1183/13993003.02730-2021
7. Sicherer SH, Sampson HA. Food allergy: epidemiology, pathogenesis, diagnosis, and treatment. J Allergy Clin Immunol. (2014) 133(2):291–307. doi: 10.1016/j.jaci.2013.11.020
8. De Martinis M, Sirufo MM, Suppa M, Ginaldi L. New perspectives in food allergy. Int J Mol Sci. (2020) 21(4):1474. doi: 10.3390/ijms21041474
9. Noti M, Kim BS, Siracusa MC, Rak GD, Kubo M, Moghaddam AE, et al. Exposure to food allergens through inflamed skin promotes intestinal food allergy through the thymic stromal lymphopoietin-basophil axis. J Allergy Clin Immunol. (2014) 133(5):1390–9. doi: 10.1016/j.jaci.2014.01.021
10. Høst A, Koletzko B, Dreborg S, Muraro A, Wahn U, Aggett P, et al. Dietary products used in infants for treatment and prevention of food allergy. Joint statement of the European society for paediatric allergology and clinical immunology (ESPACI) committee on hypoallergenic formulas and the European society for paediatric gastroenterology, hepatology and nutrition (ESPGHAN) committee on nutrition. Arch Dis Child. (1999) 81(1):80–4. doi: 10.1136/adc.81.1.80
11. Nwaru BI, Erkkola M, Ahonen S, Kaila M, Haapala AM, Kronberg-Kippilä C, et al. Age at the Introduction of solid foods during the first year and allergic sensitization at age 5 years. Pediatrics. (2022) 125(1):50–9. doi: 10.1542/peds.2009-0813
12. Du Toit G, Katz Y, Sasieni P, Mesher D, Maleki SJ, Fisher HR, et al. Early consumption of peanuts in infancy is associated with a low prevalence of peanut allergy. J Allergy Clin Immunol. (2008) 122(5):984–91. doi: 10.1016/j.jaci.2008.08.039
13. Zutavern A, Brockow I, Schaaf B, Bolte G, von Berg A, Diez U, et al. Timing of solid food Introduction in relation to atopic dermatitis and atopic sensitization: results from a prospective birth cohort study. Pediatrics. (2022) 117(2):401–11. doi: 10.1542/peds.2004-2521
14. Skjerven HO, Lie A, Vettukattil R, Rehbinder EM, LeBlanc M, Asarnoj A, et al. Early food intervention and skin emollients to prevent food allergy in young children (PreventADALL): a factorial, multicentre, cluster-randomised trial. Lancet. (2022) 399(10344):2398–411. doi: 10.1016/S0140-6736(22)00687-0
15. de Silva D, Geromi M, Panesar SS, Muraro A, Werfel T, Hoffmann-Sommergruber K, et al. Acute and long-term management of food allergy: systematic review. Allergy. (2014) 69(2):159–67. doi: 10.1111/all.12314
16. Eichenfield L, Furuta GT, Hanifin JM, Jones C, Kraft M, Levy BD, et al. Guidelines for the diagnosis and management of food allergy in the United States: report of the NIAID-sponsored expert panel. J Allergy Clin Immunol. (2010) 126(6 Suppl):S1–58. doi: 10.1016/j.jaci.2010.10.007
17. Dodi G, Di Filippo P, Di Pillo S, Chiarelli F, Attanasi M. Total serum IgE levels as predictor of the acquisition of tolerance in children with food allergy: findings from a pilot study. Front Pediatr. (2022) 10:1013807. doi: 10.3389/fped.2022.1013807
18. Liu AH, Jaramillo R, Sicherer SH, Wood RA, Bock SA, Burks AW, et al. National prevalence and risk factors for food allergy and relationship to asthma: results from the national health and nutrition examination survey 2005-2006. J Allergy Clin Immunol. (2010) 126(4):798–806. doi: 10.1016/j.jaci.2010.07.026
19. Leung DY, Boguniewicz M, Howell MD, Nomura I, Hamid QA. New insights into atopic dermatitis. J Clin Invest. (2004) 113(5):651–7. doi: 10.1172/JCI21060
20. Weidinger S, Beck LA, Bieber T, Kabashima K, Irvine AD. Atopic dermatitis. Nat Rev Dis Primers. (2018) 4(1):1. doi: 10.1038/s41572-018-0001-z
21. Graham F, Eigenmann PA. Atopic dermatitis and its relation to food allergy. Curr Opin Allergy Clin Immunol. (2020) 20(3):305–10. doi: 10.1097/aci.0000000000000638
22. Sugita K, Akdis CA. Recent developments and advances in atopic dermatitis and food allergy. Allergol Int. (2020) 69(2):204–14. doi: 10.1016/j.alit.2019.08.013
23. Schneider S, Li L, Zink A. The new era of biologics in atopic dermatitis: a review. Dermatol Pract Concept. (2021) 11(4):e2021144. doi: 10.5826/dpc.1104a144
24. Ghazal S, Ridha Z, D'Aguanno K, Nassim D, Quaiattini A, Netchiporouk E, et al. Treatment guidelines for atopic dermatitis since the approval of dupilumab: a systematic review and quality appraisal using AGREE-II. Front Med (Lausanne). (2022) 9:821871. doi: 10.3389/fmed.2022.821871
25. Spergel JM. From atopic dermatitis to asthma: the atopic march. Ann Allergy Asthma Immunol. (2010) 105(2):99–106; quiz 7–9, 17. doi: 10.1016/j.anai.2009.10.002
26. Tulic MK, Piche T, Verhasselt V. Lung–gut cross-talk: evidence, mechanisms and implications for the mucosal inflammatory diseases. Clin Exp Allergy. (2016) 46(4):519–28. doi: 10.1111/cea.12723
27. Rosace D, Gomez-Casado C, Fernandez P, Perez-Gordo M, Dominguez MDC, Vega A, et al. Profilin-mediated food-induced allergic reactions are associated with oral epithelial remodeling. J Allergy Clin Immunol. (2019) 143(2):681–90.e1. doi: 10.1016/j.jaci.2018.03.013
28. Brandt EB, Scribner TA, Akei HS, Rothenberg ME. Experimental gastrointestinal allergy enhances pulmonary responses to specific and unrelated allergens. J Allergy Clin Immunol. (2006) 118(2):420–7. doi: 10.1016/j.jaci.2006.06.009
29. Thorburn AN, McKenzie CI, Shen S, Stanley D, Macia L, Mason LJ, et al. Evidence that asthma is a developmental origin disease influenced by maternal diet and bacterial metabolites. Nat Commun. (2015) 6:7320. doi: 10.1038/ncomms8320
30. Trompette A, Gollwitzer ES, Yadava K, Sichelstiel AK, Sprenger N, Ngom-Bru C, et al. Gut microbiota metabolism of dietary fiber influences allergic airway disease and hematopoiesis. Nat Med. (2014) 20:159–66. doi: 10.1038/nm.3444
31. Canani RB, Costanzo MD, Leone L, Pedata M, Meli R, Calignano A. Potential beneficial effects of butyrate in intestinal and extraintestinal diseases. World J Gastroenterol. (2011) 17:1519–28. doi: 10.3748/wjg.v17.i12.1519
32. Elce A, Amato F, Zarrilli F, Calignano A, Troncone R, Castaldo G, et al. Butyrate modulating effects on pro-inflammatory pathways in human intestinal epithelial cells. Benefic Microbes. (2017) 8(5):841–7. doi: 10.3920/BM2016.0197
33. Akdis CA. Does the epithelial barrier hypothesis explain the increase in allergy, autoimmunity and other chronic conditions? Nat Rev Immunol. (2021) 21(11):739–51. doi: 10.1038/s41577-021-00538-7
34. Akdis CA. The epithelial barrier hypothesis proposes a comprehensive understanding of the origins of allergic and other chronic noncommunicable diseases. J Allergy Clin Immunol. (2022) 149(1):41–4. doi: 10.1016/j.jaci.2021.11.010
35. López-Rodríguez JC, Benedé S, Barderas R, Villalba M, Batanero E. Airway epithelium plays a leading role in the Complex framework underlying respiratory allergy. J Investig Allergol Clin Immunol. (2017) 27(6):346–55. doi: 10.18176/jiaci.0201
36. Ulluwishewa D, Anderson RC, McNabb WC, Moughan PJ, Wells JM, Roy NC. Regulation of tight junction permeability by intestinal bacteria and dietary components. J Nutr. (2011) 141(5):769–76. doi: 10.3945/jn.110.135657
37. Lee SH. Intestinal permeability regulation by tight junction: implication on inflammatory bowel diseases. Intest Res. (2015) 13(1):11–8. doi: 10.5217/ir.2015.13.1.11
38. Niewiem M, Grzybowska-Chlebowczyk U. Intestinal barrier permeability in allergic diseases. Nutrients. (2022) 14(9):1893. doi: 10.3390/nu14091893
39. Heinemann U, Schuetz A. Structural features of tight-junction proteins. Int J Mol Sci. (2019) 20(23):6020. doi: 10.3390/ijms20236020
40. Buckley A, Turner JR. Cell biology of tight junction barrier regulation and mucosal disease. Cold Spring Harb Perspect Biol. (2018) 10(1):a029314. doi: 10.1101/cshperspect.a029314
41. Eyerich K, Dimartino V, Cavani A. IL-17 and IL-22 in immunity: driving protection and pathology. Eur J Immunol. (2017) 47(4):607–14. doi: 10.1002/eji.201646723
42. Delbue D, Lebenheim L, Cardoso-Silva D, Dony V, Krug SM, Richter JF, et al. Reprogramming intestinal epithelial cell polarity by interleukin-22. Front Med (Lausanne). (2021) 8:656047. doi: 10.3389/fmed.2021.656047
43. Roan F, Obata-Ninomiya K, Ziegler SF. Epithelial cell-derived cytokines: more than just signaling the alarm. J Clin Invest. (2019) 129(4):1441–51. doi: 10.1172/JCI124606
44. Christensen S, Jaffar Z, Cole E, Porter V, Ferrini M, Postma B, et al. Prenatal environmental tobacco smoke exposure increases allergic asthma risk with methylation changes in mice. Environ Mol Mutagen. (2017) 58(6):423–33. doi: 10.1002/em.22097
45. Stier MT, Peebles RS Jr. Innate lymphoid cells and allergic disease. Ann Allergy Asthma Immunol. (2017) 119(6):480–8. doi: 10.1016/j.anai.2017.08.290
46. Pelaia C, Pelaia G, Longhini F, Crimi C, Calabrese C, Gallelli L, et al. Monoclonal antibodies targeting alarmins: a new perspective for biological therapies of severe asthma. Biomedicines. (2021) 9(9):1108. doi: 10.3390/biomedicines9091108
48. Chen YL, Gutowska-Owsiak D, Hardman CS, Westmoreland M, MacKenzie T, Cifuentes L, et al. Proof-of-concept clinical trial of etokimab shows a key role for IL-33 in atopic dermatitis pathogenesis. Sci Transl Med. (2019) 11(515):eaax2945. doi: 10.1126/scitranslmed.aax2945
49. Chinthrajah S, Cao S, Liu C, Lyu SC, Sindher SB, Long A, et al. Phase 2a randomized, placebo-controlled study of anti-IL-33 in peanut allergy. JCI Insight. (2019) 4(22):e131347. doi: 10.1172/jci.insight.131347
50. Zeng HT, Liu JQ, Zhao M, Yu D, Yang G, Mo LH, et al. Exosomes carry IL-10 and antigen/MHC II complexes to induce antigen-specific oral tolerance. Cytokine. (2020) 133:155176. doi: 10.1016/j.cyto.2020.155176
51. Van Niel G, Mallegol J, Bevilacqua C, Candalh C, Brugière S, Tomaskovic-Crook E, et al. Intestinal epithelial exosomes carry MHC class II/peptides able to inform the immune system in mice. Gut. (2003) 52(12):1690–7. doi: 10.1136/gut.52.12.1690
52. Steelant B, Seys SF, Boeckxstaens G, Akdis CA, Ceuppens JL, Hellings PW. Restoring airway epithelial barrier dysfunction: a new therapeutic challenge in allergic airway disease. Rhinology. (2016) 54(3):195–205. doi: 10.4193/Rhino15.376
53. Kim Y, Lee Y, Heo G, Jeong S, Park S, Yoo JW, et al. Modulation of intestinal epithelial permeability via protease-activated receptor-2-induced autophagy. Cells. (2022) 11(5):878. doi: 10.3390/cells11050878
54. Prossomariti A, Sokol H, Ricciardiello L. Nucleotide-Binding domain leucine-rich repeat containing proteins and intestinal Microbiota: pivotal players in colitis and colitis-associated cancer development. Front Immunol. (2018) 9:1039. doi: 10.3389/fimmu.2018.01039
55. Gubatan J, Holman DR, Puntasecca CJ, Polevoi D, Rubin SJ, Rogalla S. Antimicrobial peptides and the gut microbiome in inflammatory bowel disease. World J Gastroenterol. (2021) 27(43):7402–22. doi: 10.3748/wjg.v27.i43.7402
56. Lozano-Ojalvo D, Lopez-Fandino R. Immunomodulating peptides for food allergy prevention and treatment. Crit Rev Food Sci Nutr. (2018) 58(10):1629–49. doi: 10.1080/10408398.2016.1275519
57. Suzuki T, Yoshinaga N, Tanabe S. Interleukin-6 (IL-6) regulates claudin-2 expression and tight junction permeability in intestinal epithelium. J Biol Chem. (2011) 286(36):31263–71. doi: 10.1074/jbc.M111.238147
58. Akdis CA, Arkwright PD, Bruggen MC, Busse W, Gadina M, Guttman-Yassky E, et al. Type 2 immunity in the skin and lungs. Allergy. (2020) 75(7):1582–605. doi: 10.1111/all.14318
59. Steelant B, Seys SF, Van Gerven L, Van Woensel M, Farre R, Wawrzyniak P, et al. Histamine and T helper cytokine-driven epithelial barrier dysfunction in allergic rhinitis. J Allergy Clin Immunol. (2018) 141(3):951–63.e8. doi: 10.1016/j.jaci.2017.08.039
60. Rappaport SM, Smith MT. Epidemiology. Environment and disease risks. Science. (2010) 330(6003):460–1. doi: 10.1126/science.1192603
61. Annesi-Maesano I, Maesano CN, Biagioni B, D'Amato G, Cecchi L. Call to action: air pollution, asthma, and allergy in the exposome era. J Allergy Clin Immunol. (2021) 148(1):70–2. doi: 10.1016/j.jaci.2021.05.026
62. Vineis P, Demetriou CA, Probst-Hensch N. Long-term effects of air pollution: an exposome meet-in-the-middle approach. Int J Public Health. (2020) 65(2):125–7. doi: 10.1007/s00038-019-01329-7
63. Wang M, Tan G, Eljaszewicz A, Meng Y, Wawrzyniak P, Acharya S, et al. Laundry detergents and detergent residue after rinsing directly disrupt tight junction barrier integrity in human bronchial epithelial cells. J Allergy Clin Immunol. (2019) 143(5):1892–903. doi: 10.1016/j.jaci.2018.11.016
64. Acevedo N, Zakzuk J, Caraballo L. House dust Mite allergy under changing environments. Allergy Asthma Immunol Res. (2019) 11(4):450–69. doi: 10.4168/aair.2019.11.4.450
65. Dronen EK, Namork E, Dirven H, Nygaard UC. Suspected gut barrier disruptors and development of food allergy: adjuvant effects and early immune responses. Front Allergy. (2022) 3:1029125. doi: 10.3389/falgy.2022.1029125
66. Viennois E, Chassaing B. First victim, later aggressor: how the intestinal microbiota drives the pro-inflammatory effects of dietary emulsifiers? Gut Microbes. (2018) 9(3):289–91. doi: 10.1080/19490976.2017.1421885
67. Sozener Z C, Cevhertas L, Nadeau K, Akdis M, Akdis CA. Environmental factors in epithelial barrier dysfunction. J Allergy Clin Immunol. (2020) 145(6):1517–28. doi: 10.1016/j.jaci.2020.04.024
68. Celebi Sozener Z, Ozdel Ozturk B, Cerci P, Turk M, Gorgulu Akin B, Akdis M, et al. Epithelial barrier hypothesis: effect of the external exposome on the microbiome and epithelial barriers in allergic disease. Allergy. (2022) 77(5):1418–49. doi: 10.1111/all.15240
69. Mitamura Y, Ogulur I, Pat Y, Rinaldi AO, Ardicli O, Cevhertas L, et al. Dysregulation of the epithelial barrier by environmental and other exogenous factors. Contact Dermatitis. (2021) 85(6):615–26. doi: 10.1111/cod.13959
70. Perrier C, Corthésy B. Gut permeability and food allergies. Clin Exp Allergy. (2011) 41(1):20–8. doi: 10.1111/j.1365-2222.2010.03639.x
71. López-Rodríguez JC, Rodríguez-Coira J, Benedé S, Barbas C, Barber D, Villalba MT, et al. Comparative metabolomics analysis of bronchial epithelium during barrier establishment after allergen exposure. Clinical Trans Allergy. (2021) 11(7):e12051. doi: 10.1002/clt2.12051
72. Olivera DS, Boggs SE, Beenhouwer C, Aden J, Knall C. Cellular mechanisms of mainstream cigarette smoke-induced lung epithelial tight junction permeability changes in vitro. Inhal Toxicol. (2007) 19(1):13–22. doi: 10.1080/08958370600985768
73. Short KR, Kasper J, van der Aa S, Andeweg AC, Zaaraoui-Boutahar F, Goeijenbier M, et al. Influenza virus damages the alveolar barrier by disrupting epithelial cell tight junctions. Eur Respir J. (2016) 47(3):954–66. doi: 10.1183/13993003.01282-2015
74. Wan H, Winton HL, Soeller C, Tovey ER, Gruenert DC, Thompson PJ, et al. Der p 1 facilitates transepithelial allergen delivery by disruption of tight junctions. J Clin Invest. (1999) 104(1):123–33. doi: 10.1172/JCI5844
75. Kauffman HF, Tamm M, Timmerman JA, Borger P. House dust mite major allergens der p 1 and der p 5 activate human airway-derived epithelial cells by protease-dependent and protease-independent mechanisms. Clin Mol Allergy. (2006) 4:5. doi: 10.1186/1476-7961-4-5
76. Balenga NA, Klichinsky M, Xie Z, Chan EC, Zhao M, Jude J, et al. A fungal protease allergen provokes airway hyper-responsiveness in asthma. Nat Commun. (2015) 6:6763. doi: 10.1038/ncomms7763
77. López-Rodríguez JC, González M, Bogas G, Mayorga C, Villalba M, Batanero E. Epithelial permeability to ole e 1 is more dependent on the functional state of the bronchial epithelium than on the activity of der p 1 protease acting as an adjuvant to the bystander allergen. J Investig Allergol Clin Immunol. (2021) 31(4):343–6. doi: 10.18176/jiaci.0603
78. Georas SN, Rezaee F. Epithelial barrier function: at the front line of asthma immunology and allergic airway inflammation. J Allergy Clin Immunol. (2014) 134(3):509–20. doi: 10.1016/j.jaci.2014.05.049
79. Abu Khweek A, Kim E, Joldrichsen MR, Amer AO, Boyaka PN. Insights into mucosal innate immune responses in house dust Mite-mediated allergic asthma. Front Immunol. (2020) 11:534501. doi: 10.3389/fimmu.2020.534501
80. López-Rodríguez JC, Manosalva J, Cabrera-García JD, Escribese MM, Villalba M, Barber D, et al. Human glutathione-S-transferase pi potentiates the cysteine-protease activity of the der p 1 allergen from house dust mite through a cysteine redox mechanism. Redox Biol. (2019) 26:101256. doi: 10.1016/j.redox.2019.101256
81. McKelvey MC, Brown R, Ryan S, Mall MA, Weldon S, Taggart CC. Proteases, mucus, and mucosal immunity in chronic lung disease. Int J Mol Sci. (2021) 22(9):5018. doi: 10.3390/ijms22095018
82. Lin J, Chen D, Guan L, Chang K, Li D, Sun B, et al. House dust mite exposure enhances immune responses to ovalbumin-induced intestinal allergy. Sci Rep. (2022) 12(1):5216. doi: 10.1038/s41598-022-09196-8
83. Van Spaendonk H, Ceuleers H, Witters L, Patteet E, Joossens J, Augustyns K, et al. Regulation of intestinal permeability: the role of proteases. World J Gastroenterol. (2017) 23(12):2106–23. doi: 10.3748/wjg.v23.i12.2106
84. Grozdanovic MM, Cavic M, Nesic A, Andjelkovic U, Akbari P, Smit JJ, et al. Kiwifruit cysteine protease actinidin compromises the intestinal barrier by disrupting tight junctions. Biochim Biophys Acta. (2016) 1860(3):516–26. doi: 10.1016/j.bbagen.2015.12.005
85. Tulic MK, Vivinus-Nebot M, Rekima A, Rabelo Medeiros S, Bonnart C, Shi H, et al. Presence of commensal house dust mite allergen in human gastrointestinal tract: a potential contributor to intestinal barrier dysfunction. Gut. (2016) 65(5):757–66. doi: 10.1136/gutjnl-2015-310523
86. Takahashi K, Yanuma N, Hirokawa M, Miyajima M, Ogawa M, Osada H, et al. Presence of the house dust mite allergen in the gastrointestinal tract of dogs with chronic enteropathy: a potential inducer of interleukin-1beta. Vet Immunol Immunopathol. (2020) 230:110150. doi: 10.1016/j.vetimm.2020.110150
87. Murrison LB, Brandt EB, Myers JB, Hershey GKK. Environmental exposures and mechanisms in allergy and asthma development. J Clin Invest. (2019) 129(4):1504–15. doi: 10.1172/JCI124612
88. Khreis H, Kelly C, Tate J, Parslow R, Lucas K, Nieuwenhuijsen M. Exposure to traffic-related air pollution and risk of development of childhood asthma: a systematic review and meta-analysis. Environ Int. (2017) 100:1–31. doi: 10.1016/j.envint.2016.11.012
89. Yue H, Yan W, Ji X, Zhang Y, Li G, Sang N. Maternal exposure to NO(2) enhances airway sensitivity to allergens in BALB/c mice through the JAK-STAT6 pathway. Chemosphere. (2018) 200:455–63. doi: 10.1016/j.chemosphere.2018.02.116
90. De Grove KC, Provoost S, Hendriks RW, McKenzie ANJ, Seys LJM, Kumar S, et al. Dysregulation of type 2 innate lymphoid cells and T(H)2 cells impairs pollutant-induced allergic airway responses. J Allergy Clin Immunol. (2017) 139(1):246–57.e4. doi: 10.1016/j.jaci.2016.03.044
91. Bayram H, Sapsford RJ, Abdelaziz MM, Khair OA. Effect of ozone and nitrogen dioxide on the release of proinflammatory mediators from bronchial epithelial cells of nonatopic nonasthmatic subjects and atopic asthmatic patients in vitro. J Allergy Clin Immunol. (2001) 107(2):287–94. doi: 10.1067/mai.2001.111141
92. Mirowsky JE, Dailey LA, Devlin RB. Differential expression of pro-inflammatory and oxidative stress mediators induced by nitrogen dioxide and ozone in primary human bronchial epithelial cells. Inhal Toxicol. (2016) 28(8):374–82. doi: 10.1080/08958378.2016.1185199
93. Baena-Cagnani CE, Gomez RM, Baena-Cagnani R, Canonica GW. Impact of environmental tobacco smoke and active tobacco smoking on the development and outcomes of asthma and rhinitis. Curr Opin Allergy Clin Immunol. (2009) 9(2):136–40. doi: 10.1097/ACI.0b013e3283294038
94. Burke H, Leonardi-Bee J, Hashim A, Pine-Abata H, Chen Y, Cook DG, et al. Prenatal and passive smoke exposure and incidence of asthma and wheeze: systematic review and meta-analysis. Pediatrics. (2012) 129(4):735–44. doi: 10.1542/peds.2011-2196
95. Jin Y, Seiber EE, Ferketich AK. Secondhand smoke and asthma: what are the effects on healthcare utilization among children? Prev Med. (2013) 57(2):125–8. doi: 10.1016/j.ypmed.2013.05.003
96. Sun YB, Liu M, Fan XS, Zhou LP, Li MW, Hu FY, et al. Effects of cigarette smoke on the aggravation of ovalbumin-induced asthma and the expressions of TRPA1 and tight junctions in mice. Mol Immunol. (2021) 135:62–72. doi: 10.1016/j.molimm.2021.04.006
97. Wu CC, Hsu TY, Chang JC, Ou CY, Kuo HC, Liu CA, et al. Paternal tobacco smoke correlated to offspring asthma and prenatal epigenetic programming. Front Genet. (2019) 10:471. doi: 10.3389/fgene.2019.00471
98. Lu K, Lai KP, Stoeger T, Ji S, Lin Z, Lin X, et al. Detrimental effects of microplastic exposure on normal and asthmatic pulmonary physiology. J Hazard Mater. (2021) 416:126069. doi: 10.1016/j.jhazmat.2021.126069
99. Sly PD, Kusel M, Holt PG. Do early-life viral infections cause asthma? J Allergy Clini Immunol. (2010) 125(6):1202–5. doi: 10.1016/j.jaci.2010.01.024
100. Mackenzie KJ, Anderton SM, Schwarze J. Viral respiratory tract infections and asthma in early life: cause and effect? Clin Exp Allergy. (2014) 44(1):9–19. doi: 10.1111/cea.12246
101. Heine RG. Food allergy prevention and treatment by targeted nutrition. Ann Nutr Metab. (2018) 72(Suppl 3):33–45. doi: 10.1159/000487380
102. De Nuccio F, Piscitelli P, Toraldo DM. Gut–lung Microbiota interactions in chronic obstructive pulmonary disease (COPD): potential mechanisms driving progression to COPD and epidemiological data. Lung. (2022) 200(6):773–81. doi: 10.1007/s00408-022-00581-8
103. Dang AT, Marsland BJ. Microbes, metabolites, and the gut-lung axis. Mucosal Immunol. (2019) 12(4):843–50. doi: 10.1038/s41385-019-0160-6
104. Suzuki T. Regulation of the intestinal barrier by nutrients: the role of tight junctions. Anim Sci J. (2020) 91(1):e13357. doi: 10.1111/asj.13357
105. Halken S, Muraro A, de Silva D, Khaleva E, Angier E, Arasi S, et al. EAACI Guideline: preventing the development of food allergy in infants and young children (2020 update). Pediatr Allergy Immunol. (2021) 32(5):843–58. doi: 10.1111/pai.13496
106. Lodge CJ, Tan DJ, Lau MX, Dai X, Tham R, Lowe AJ, et al. Breastfeeding and asthma and allergies: a systematic review and meta-analysis. Acta Paediatr. (2015) 104(467):38–53. doi: 10.1111/apa.13132
107. Oddy WH. Breastfeeding, childhood asthma, and allergic disease. Ann Nutr Metab. (2017) 70(Suppl 2):26–36. doi: 10.1159/000457920
108. Vidal K, Labéta MO, Schiffrin EJ, Donnet-Hughes A. Soluble CD14 in human breast milk and its role in innate immune responses. Acta Odontol Scand. (2001) 59(5):330–4. doi: 10.1080/000163501750541219
109. Zanoni I, Ostuni R, Marek LR, Barresi S, Barbalat R, Barton GM, et al. CD14 Controls the LPS-induced endocytosis of toll-like receptor 4. Cell. (2011) 147(4):868–80. doi: 10.1016/j.cell.2011.09.051
110. Hua MC, Su HM, Kuo ML, Chen CC, Yao TC, Tsai MH, et al. Association of maternal allergy with human milk soluble CD14 and fatty acids, and early childhood atopic dermatitis. Pediatr Allergy Immunol. (2019) 30(2):204–13. doi: 10.1111/pai.13011
111. Fikri B, Tani Y, Nagai K, Sahara M, Mitsuishi C, Togawa Y, et al. Soluble CD14 in breast milk and its relation to atopic manifestations in early infancy. Nutrients. (2019) 11(9):2118. doi: 10.3390/nu11092118
112. Dawod B, Marshall JS. Cytokines and soluble receptors in breast milk as enhancers of oral tolerance development. Front Immunol. (2019) 10:16. doi: 10.3389/fimmu.2019.00016
113. Akdis CA, Akdis M. Mechanisms of immune tolerance to allergens: role of IL-10 and tregs. J Clin Invest. (2014) 124(11):4678–80. doi: 10.1172/JCI78891
114. Pastor-Vargas C, Maroto AS, Díaz-Perales A, Villaba M, Casillas Diaz N, Vivanco F, et al. Sensitive detection of major food allergens in breast milk: first gateway for allergenic contact during breastfeeding. Allergy. (2015) 70(8):1024–7. doi: 10.1111/all.12646
115. Kosmeri C, Rallis D, Kostara M, Siomou E, Tsabouri S. Characteristics of exogenous allergen in breast milk and their impact on oral tolerance induction. Front Pediatr. (2022) 10:830718. doi: 10.3389/fped.2022.830718
116. Pastor-Vargas C, Maroto AS, Díaz-Perales A, Villalba M, Esteban V, Ruiz-Ramos M, et al. Detection of major food allergens in amniotic fluid: initial allergenic encounter during pregnancy. Pediatr Allergy Immunol. (2016) 27(7):716–20. doi: 10.1111/pai.12608
117. Mosconi E, Rekima A, Seitz-Polski B, Kanda A, Fleury S, Tissandie E, et al. Breast milk immune complexes are potent inducers of oral tolerance in neonates and prevent asthma development. Mucosal Immunol. (2010) 3(5):461–74. doi: 10.1038/mi.2010.23
118. Ohsaki A, Venturelli N, Buccigrosso TM, Osganian SK, Lee J, Blumberg RS, et al. Maternal IgG immune complexes induce food allergen-specific tolerance in offspring. J Exp Med. (2018) 215(1):91–113. doi: 10.1084/jem.20171163
119. Rajani PS, Seppo AE, Järvinen KM. Immunologically active components in human milk and development of atopic disease, with emphasis on food allergy, in the pediatric population. Front Pediatrics. (2018) 6:218. doi: 10.3389/fped.2018.00218
120. Lauritzen L, Halkjaer LB, Mikkelsen TB, Olsen SF, Michaelsen KF, Loland L, et al. Fatty acid composition of human milk in atopic danish mothers. Am J Clin Nutr. (2006) 84(1):190–6. doi: 10.1093/ajcn/84.1.190
121. Wicinski M, Sawicka E, Gebalski J, Kubiak K, Malinowski B. Human milk oligosaccharides: health benefits, potential applications in infant formulas, and pharmacology. Nutrients. (2020) 12(1):266. doi: 10.3390/nu12010266
122. Simopoulos AP. Omega-3 fatty acids in inflammation and autoimmune diseases. J Am Coll Nutr. (2002) 21(6):495–505. doi: 10.1080/07315724.2002.10719248
123. Miles EA, Childs CE, Calder PC. Long-Chain polyunsaturated fatty acids (LCPUFAs) and the developing immune system: a narrative review. Nutrients. (2021) 13(1):247. doi: 10.3390/nu13010247
124. D'Vaz N, Meldrum SJ, Dunstan JA, Lee-Pullen TF, Metcalfe J, Holt BJ, et al. Fish oil supplementation in early infancy modulates developing infant immune responses. Clin Exp Allergy. (2012) 42(8):1206–16. doi: 10.1111/j.1365-2222.2012.04031.x
125. Li E, Horn N, Ajuwon KM. EPA And DHA inhibit endocytosis of claudin-4 and protect against deoxynivalenol-induced intestinal barrier dysfunction through PPARγ dependent and independent pathways in jejunal IPEC-J2 cells. Food Res Int. (2022) 157:111420. doi: 10.1016/j.foodres.2022.111420
126. Xiao K, Liu C, Qin Q, Zhang Y, Wang X, Zhang J, et al. EPA And DHA attenuate deoxynivalenol-induced intestinal porcine epithelial cell injury and protect barrier function integrity by inhibiting necroptosis signaling pathway. FASEB J. (2020) 34(2):2483–96. doi: 10.1096/fj.201902298R
127. Willemsen LE, Koetsier MA, Balvers M, Beermann C, Stahl B, van Tol EAF. Polyunsaturated fatty acids support epithelial barrier integrity and reduce IL-4 mediated permeability in vitro. Eur J Nutr. (2008) 47(4):183–91. doi: 10.1007/s00394-008-0712-0
128. Nordgren TM, Heires AJ, Bailey KL, Katafiasz DM, Toews ML, Wichman CS, et al. Docosahexaenoic acid enhances amphiregulin-mediated bronchial epithelial cell repair processes following organic dust exposure. Am J Physiol Lung Cell Mol Physiol. (2018) 314(3):L421–L31. doi: 10.1152/ajplung.00273.2017
129. Gombart AF, Borregaard N, Koeffler HP. Human cathelicidin antimicrobial peptide (CAMP) gene is a direct target of the vitamin D receptor and is strongly up-regulated in myeloid cells by 1,25-dihydroxyvitamin D3. FASEB J. (2005) 19(9):1067–77. doi: 10.1096/fj.04-3284com
130. Briceno Noriega D, Savelkoul HFJ. Vitamin D and allergy susceptibility during gestation and early life. Nutrients. (2021) 13(3):1015. doi: 10.3390/nu13031015
131. Kanhere M, Chassaing B, Gewirtz AT, Tangpricha V. Role of vitamin D on gut microbiota in cystic fibrosis. J Steroid Biochem Mol Biol. (2018) 175:82–7. doi: 10.1016/j.jsbmb.2016.11.001
132. Murdaca G, Gerosa A, Paladin F, Petrocchi L, Banchero S, Gangemi S. Vitamin D and Microbiota: is there a link with allergies? Int J Mol Sci. (2021) 22(8):4288. doi: 10.3390/ijms22084288
133. Yepes-Nuñez JJ, Brożek JL, Fiocchi A, Pawankar R, Cuello-García C, Zhang Y, et al. Vitamin D supplementation in primary allergy prevention: systematic review of randomized and non-randomized studies. Allergy. (2018) 73(1):37–49. doi: 10.1111/all.13241
134. Venter C, Agostoni C, Arshad SH, Ben-Abdallah M, Du Toit G, Fleischer DM, et al. Dietary factors during pregnancy and atopic outcomes in childhood: a systematic review from the European academy of allergy and clinical immunology. Pediatr Allergy Immunol. (2020) 31(8):889–912. doi: 10.1111/pai.13303
135. Litonjua AA, Carey VJ, Laranjo N, Stubbs BJ, Mirzakhani H, O'Connor GT, et al. Six-Year follow-up of a trial of antenatal vitamin D for asthma reduction. N Engl J Med. (2020) 382(6):525–33. doi: 10.1056/NEJMoa1906137
136. Baris S, Kiykim A, Ozen A, Tulunay A, Karakoc-Aydiner E, Barlan IB. Vitamin D as an adjunct to subcutaneous allergen immunotherapy in asthmatic children sensitized to house dust mite. Allergy. (2014) 69(2):246–53. doi: 10.1111/all.12278
137. Thorisdottir B, Gunnarsdottir I, Vidarsdottir AG, Sigurdardottir S, Birgisdottir BE, Thorsdottir I. Infant feeding, vitamin D and IgE sensitization to food allergens at 6 years in a longitudinal Icelandic cohort. Nutrients. (2019) 11(7):1690. doi: 10.3390/nu11071690
138. Forno E, Bacharier LB, Phipatanakul W, Guilbert TW, Cabana MD, Ross K, et al. Effect of vitamin D3 supplementation on severe asthma exacerbations in children with asthma and low vitamin D levels: the VDKA randomized clinical trial. JAMA. (2020) 324(8):752–60. doi: 10.1001/jama.2020.12384
139. Kim E, Bonnegarde-Bernard A, Opiyo SO, Joldrichsen MR, Attia Z, Ahmer BH, et al. Pollutants enhance IgE sensitization in the gut via local alteration of vitamin D-metabolizing enzymes. Mucosal Immunol. (2022) 15(1):143–53. doi: 10.1038/s41385-021-00440-4
140. Nowak S, Wang H, Schmidt B, Jarvinen KM. Vitamin D and iron status in children with food allergy. Ann Allergy Asthma Immunol. (2021) 127(1):57–63. doi: 10.1016/j.anai.2021.02.027
141. Rosenlund H, Magnusson J, Kull I, Håkansson N, Wolk A, Pershagen G, et al. Antioxidant intake and allergic disease in children. Clin Exp Allergy. (2012) 42(10):1491–500. doi: 10.1111/j.1365-2222.2012.04053.x
142. Shams MH, Jafari R, Eskandari N, Masjedi M, Kheirandish F, Ganjalikhani Hakemi M, et al. Anti-allergic effects of vitamin E in allergic diseases: an updated review. Int Immunopharmacol. (2021) 90:107196. doi: 10.1016/j.intimp.2020.107196
143. Pieper-Fürst U, Dao V-A, Shah-Hosseini K, Panin G, Lamprecht J, Mösges R. Alpha-tocopherol acetate nasal spray in the treatment of pollen-induced allergic rhinitis. Allergo J Int. (2018) 28(5):152–9. doi: 10.1007/s40629-018-0086-7
144. Lee EJ, Ji GE, Sung MK. Quercetin and kaempferol suppress immunoglobulin E-mediated allergic inflammation in RBL-2H3 and caco-2 cells. Inflamm Res. (2010) 59(10):847–54. doi: 10.1007/s00011-010-0196-2
145. Gong JH, Shin D, Han SY, Kim JL, Kang YH. Kaempferol suppresses eosionphil infiltration and airway inflammation in airway epithelial cells and in mice with allergic asthma. J Nutr. (2012) 142(1):47–56. doi: 10.3945/jn.111.150748
146. Sozmen SC, Karaman M, Cilaker Micili S, Isik S, Bagriyanik A, Arikan Ayyildiz Z, et al. Effects of quercetin treatment on epithelium-derived cytokines and epithelial cell apoptosis in allergic airway inflammation mice model. Iran J Allergy Asthma Immunol. (2016) 15(6):487–97.28129681
147. Antoni L, Nuding S, Wehkamp J, Stange EF. Intestinal barrier in inflammatory bowel disease. World J Gastroenterol. (2014) 20(5):1165–79. doi: 10.3748/wjg.v20.i5.1165
148. König J, Wells J, Cani PD, García-Ródenas CL, Macdonald T, Mercenier A, et al. Human intestinal barrier function in health and disease. Clin Trans Gastroenterol. (2016) 7:e196. doi: 10.1038/ctg.2016.54
149. Glynn A, Igra AM, Sand S, Ilback NG, Hellenas KE, Rosen J, et al. Are additive effects of dietary surfactants on intestinal tight junction integrity an overlooked human health risk? - A mixture study on caco-2 monolayers. Food Chem Toxicol. (2017) 106(Pt A):314–23. doi: 10.1016/j.fct.2017.05.068
150. Lu Y, Wang YY, Yang N, Zhang D, Zhang FY, Gao HT, et al. Food emulsifier polysorbate 80 increases intestinal absorption of di-(2-ethylhexyl) phthalate in rats. Toxicol Sci. (2014) 139(2):317–27. doi: 10.1093/toxsci/kfu055
151. Zhu YT, Yuan YZ, Feng QP, Hu MY, Li WJ, Wu X, et al. Food emulsifier polysorbate 80 promotes the intestinal absorption of mono-2-ethylhexyl phthalate by disturbing intestinal barrier. Toxicol Appl Pharmacol. (2021) 414:115411. doi: 10.1016/j.taap.2021.115411
152. Khuda SE, Nguyen AV, Sharma GM, Alam MS, Balan KV, Williams KM. Effects of emulsifiers on an in vitro model of intestinal epithelial tight junctions and the transport of food allergens. Mol Nutr Food Res. (2022) 66(4):e2100576. doi: 10.1002/mnfr.202100576
153. Nishimura S, Aoi W, Kodani H, Kobayashi Y, Wada S, Kuwahata M, et al. Polysorbate 80-induced leaky gut impairs skeletal muscle metabolism in mice. Physiol Rep. (2020) 8(20):e14629. doi: 10.14814/phy2.14629
154. Laudisi F, Di Fusco D, Dinallo V, Stolfi C, Di Grazia A, Marafini I, et al. The food additive maltodextrin promotes endoplasmic Reticulum stress-driven mucus depletion and exacerbates intestinal inflammation. Cell Mol Gastroenterol Hepatol. (2019) 7(2):457–73. doi: 10.1016/j.jcmgh.2018.09.002
155. Drago S, El Asmar R, Di Pierro M, Grazia Clemente M, Tripathi A, Sapone A, et al. Gliadin, zonulin and gut permeability: effects on celiac and non-celiac intestinal mucosa and intestinal cell lines. Scand J Gastroenterol. (2006) 41(4):408–19. doi: 10.1080/00365520500235334
156. Hashimoto K, Kawagishi H, Nakayama T, Shimizu M. Effect of capsianoside, a diterpene glycoside, on tight-junctional permeability. Biochim Biophys Acta. (1997) 1323(2):281–90. doi: 10.1016/s0005-2736(96)00196-4
157. Jensen-Jarolim E, Gajdzik L, Haberl I, Kraft D, Scheiner O, Graf J. Hot spices influence permeability of human intestinal epithelial monolayers. J Nutr. (1998) 128(3):577–81. doi: 10.1093/jn/128.3.577
158. Mohamed Nor NH, Kooi M, Diepens NJ, Koelmans AA. Lifetime accumulation of microplastic in children and adults. Environ Sci Technol. (2021) 55(8):5084–96. doi: 10.1021/acs.est.0c07384
159. Jung YS, Sampath V, Prunicki M, Aguilera J, Allen H, LaBeaud D, et al. Characterization and regulation of microplastic pollution for protecting planetary and human health. Environ Pollut. (2022) 315:120442. doi: 10.1016/j.envpol.2022.120442
160. Jin Y, Lu L, Tu W, Luo T, Fu Z. Impacts of polystyrene microplastic on the gut barrier, microbiota and metabolism of mice. Sci Total Environ. (2019) 649:308–17. doi: 10.1016/j.scitotenv.2018.08.353
161. Lu L, Wan Z, Luo T, Fu Z, Jin Y. Polystyrene microplastics induce gut microbiota dysbiosis and hepatic lipid metabolism disorder in mice. Sci Total Environ. (2018) 631–632:449–58. doi: 10.1016/j.scitotenv.2018.03.051
162. Hirt N, Body-Malapel M. Immunotoxicity and intestinal effects of nano- and microplastics: a review of the literature. Part Fibre Toxicol. (2020) 17(1):57. doi: 10.1186/s12989-020-00387-7
163. Hwang J, Choi D, Han S, Choi J, Hong J. An assessment of the toxicity of polypropylene microplastics in human derived cells. Sci Total Environ. (2019) 684:657–69. doi: 10.1016/j.scitotenv.2019.05.071
164. Lei L, Wu S, Lu S, Liu M, Song Y, Fu Z, et al. Microplastic particles cause intestinal damage and other adverse effects in zebrafish danio rerio and nematode caenorhabditis elegans. Sci Total Environ. (2018) 619–620:1–8. doi: 10.1016/j.scitotenv.2017.11.103
165. Molina E, Benede S. Is there evidence of health risks from exposure to micro- and nanoplastics in foods? Front Nutr. (2022) 9:910094. doi: 10.3389/fnut.2022.910094
166. Finlay BB, Reynolds A. A case for antibiotic perturbation of the microbiota leading to allergy development. Expert Rev Clin Immunol. (2013) 9(11):1019–30. doi: 10.1586/1744666X.2013.851603
167. Isolauri SS, Gibson GR, McCartney AL. Influence of mode of delivery on gut microbiota composition in seven year old children. Gut. (2004) 53:1386–90. doi: 10.1136/gut.2004.041640
168. Zubeldia-Varela E, Barker-Tejeda TC, Obeso D, Villasenor A, Barber D, Perez-Gordo M. Microbiome and allergy: new insights and perspectives. J Investig Allergol Clin Immunol. (2022) 32(5):327–44. doi: 10.18176/jiaci.0852
169. Hou K, Wu ZX, Chen XY, Wang JQ, Zhang D, Xiao C, et al. Microbiota in health and diseases. Signal Transduct Target Ther. (2022) 7(1):135. doi: 10.1038/s41392-022-00974-4
170. Kumar J, Rani K, Datt C. Molecular link between dietary fibre, gut microbiota and health. Mol Biol Rep. (2020) 47:6229–37. doi: 10.1007/s11033-020-05611-3
171. King CH, Desai H, Sylvetsky AC, LoTempio J, Ayanyan S, Carrie J, et al. Baseline human gut microbiota profile in healthy people and standard reporting template. PLoS One. (2019) 14(9):e0206484. doi: 10.1371/journal.pone.0206484
172. Valitutti F, Cucchiara S, Fasano A. Celiac disease and the microbiome. Nutrients. (2019) 11(10):2403. doi: 10.3390/nu11102403
173. Molloy J, Allen K, Collier F, Tang ML, Ward AC, Vuillermin P. The potential link between gut microbiota and IgE-mediated food allergy in early life. Int J Environ Res Public Health. (2013) 10(12):7235–56. doi: 10.3390/ijerph10127235
174. Schwierzeck V, Hülpüsch C, Reiger M. Microbiome of barrier organs in allergy: who runs the world? Germs!. Handb Exp Pharmacol. (2022) 268:53–65. doi: 10.1007/164_2021_478
175. Mele ER, Pauline R, Marco C, Francesco F. Giacinto abele donato M, antonio G, et al. What is the healthy gut Microbiota composition? A changing ecosystem across age, environment, diet, and diseases. Microorganisms. (2019) 7(1):14. doi: 10.3390/microorganisms7010014
176. Fisher CK, Mehta P. Identifying keystone species in the human gut microbiome from metagenomic timeseries using sparse linear regression. PLoS One. (2014) 9(7):e102451. doi: 10.1371/journal.pone.0102451
178. Thompson-Chagoyan OC, Vieites JM, Maldonado J, Edwards C, Gil A. Changes in faecal microbiota of infants with cow's Milk protein allergy–a spanish prospective case-control 6-month follow-up study. Pediatr Allergy Immunol. (2010) 21(2 Pt 2):e394–400. doi: 10.1111/j.1399-3038.2009.00961.x
179. Thompson-Chagoyan OC, Fallani M, Maldonado J, Vieites JM, Khanna S, Edwards C, et al. Faecal microbiota and short-chain fatty acid levels in faeces from infants with cow's Milk protein allergy. Int Arch Allergy Immunol. (2011) 156(3):325–32. doi: 10.1159/000323893
180. Griffiths ZL, Zailing L, Xia L, Yiwen C, Yueqiu L, Xiaojuan T, et al. Altered fecal Microbiota composition associated with food allergy in infants. Appl Environ Microbiol. (2014) 80(8):2456–554. doi: 10.1128/AEM.00003-14
181. Topping DL, Clifton PM. Short-chain fatty acids and human colonic function: roles of resistant starch and nonstarch polysaccharides. Physiol Rev. (2001) 81(3):1031–64. doi: 10.1152/physrev.2001.81.3.1031
182. Cait A, Cardenas E, Dimitriu PA, Amenyogbe N, Dai D, Cait J, et al. Reduced genetic potential for butyrate fermentation in the gut microbiome of infants who develop allergic sensitization. J Allergy Clin Immunol. (2019) 144:1638–47.e3. doi: 10.1016/j.jaci.2019.06.029
183. Lecona E, Olmo N, Turnay J, Santiago-Gómez A, López de Silanes I, Gorospe M, et al. Kinetic analysis of butyrate transport in human colon adenocarcinoma cells reveals two different carrier-mediated mechanisms. Biochem J. (2008) 409(1):311–20. doi: 10.1042/BJ20070374
184. Luu M, Monning H, Visekruna A. Exploring the molecular mechanisms underlying the protective effects of microbial SCFAs on intestinal tolerance and food allergy. Front Immunol. (2020) 11:1225. doi: 10.3389/fimmu.2020.01225
185. Pral LP, Fachi JL, Corrêa RO, Colonna M, Vinolo MAR. Hypoxia and HIF-1 as key regulators of gut microbiota and host interactions. Trends Immunol. (2021) 42(7):604–21. doi: 10.1016/j.it.2021.05.004
186. Tan J, McKenzie C, Potamitis M, Thorburn AN, Mackay CR, Macia L. The role of short-chain fatty acids in health and disease. Adv Immunol. (2014) 121:91–119. doi: 10.1016/B978-0-12-800100-4.00003-9
187. Singh N, Gurav A, Sivaprakasam S, Brady E, Padia R, Shi H, et al. Activation of Gpr109a, receptor for niacin and the commensal metabolite butyrate, suppresses colonic inflammation and carcinogenesis. Immunity. (2014) 40:128–39. doi: 10.1016/j.immuni.2013.12.007
188. Macia L, Tan J, Vieira AT, Leach K, Stanley D, Luong S, et al. Metabolite-sensing receptors GPR43 and GPR109A facilitate dietary fibre-induced gut homeostasis through regulation of the inflammasome. Nat Commun. (2015) 6:6734. doi: 10.1038/ncomms7734
189. Mariño E, Richards JL, McLeod KH, Stanley D, Yap YA, Knight J, et al. Gut microbial metabolites limit the frequency of autoimmune T cells and protect against type 1 diabetes. Nat Immunol. (2017) 18(5):552–62. doi: 10.1038/ni.3713
190. Tan J, McKenzie C, Vuillermin PJ, Goverse G, Vinuesa CG, Mebius RE, et al. Dietary fiber and bacterial SCFA enhance oral tolerance and protect against food allergy through diverse cellular pathways. Cell Rep. (2016) 15:2809–24. doi: 10.1016/j.celrep.2016.05.047
191. Zhao Y, Chen F, Wu W, Sun M, Bilotta AJ, Yao S, et al. GPR43 Mediates microbiota metabolite SCFA regulation of antimicrobial peptide expression in intestinal epithelial cells via activation of mTOR and STAT3. Mucosal Immunol. (2018) 11(3):752–62. doi: 10.1038/mi.2017.118
192. Brown AJ, Goldsworthy SM, Barnes AA, Eilert MM, Tcheang L, Daniels D, et al. The orphan G protein-coupled receptors GPR41 and GPR43 are activated by propionate and other short chain carboxylic acids. J Biol Chem. (2003) 278(13):11312–9. doi: 10.1074/jbc.M211609200
193. Li M, van Esch BCAM, Wagenaar GTM, Garssen J, Folkerts G, Henricks PAJ. Pro- and anti-inflammatory effects of short chain fatty acids on immune and endothelial cells. Eur J Pharmacol. (2018) 831:52–9. doi: 10.1016/j.ejphar.2018.05.003
194. Kalina U, Koyama N, Hosoda T, Nuernberger H, Sato K, Hoelzer D, et al. Enhanced production of IL-18 in butyrate-treated intestinal epithelium by stimulation of the proximal promoter region. Eur J Immunol. (2002) 32(9):2635–43. doi: 10.1002/1521-4141(200209)32:9%3C2635::AID-IMMU2635%3E3.0.CO;2-N
195. Ratsimandresy RA, Indramohan M, Dorfleutner A, Stehlik C. The AIM2 inflammasome is a central regulator of intestinal homeostasis through the IL-18/IL-22/STAT3 pathway. Cell Mol Immunol. (2017) 14(1):127–42. doi: 10.1038/cmi.2016.35
196. Smith PM, Howitt MR, Panikov N, Michaud M, Gallini CA, Bohlooly-Y M, et al. The microbial metabolites, short-chain fatty acids, regulate colonic T reg cell homeostasis. Science. (2013) 341:569–73. doi: 10.1126/science.1241165
197. Li W, Sun Z. Mechanism of action for HDAC inhibitors-insights from omics approaches. Int J Mol Sci. (2019) 20(7):1616. doi: 10.3390/ijms20071616
198. Tsolis RM, Revzin A, Lebrilla CB, Bäumler AJ XBM, Olsan EE, et al. Microbiota-activated PPAR-γ signaling inhibits dysbiotic Enterobacteriaceae expansion. Science. (2017) 357(6351):570–5. doi: 10.1126/science.aam9949
199. Kinoshita M, Suzuki Y, Saito Y. Butyrate reduces colonic paracellular permeability by enhancing PPARgamma activation. Biochem Biophys Res Commun. (2002) 293(2):827–31. doi: 10.1016/S0006-291X(02)00294-2
200. Antunes KH, Fachi JL, de Paula R, da Silva EF, Pral LP, Dos Santos A, et al. Microbiota-derived acetate protects against respiratory syncytial virus infection through a GPR43-type 1 interferon response. Nat Commun. (2019) 10(1):3273. doi: 10.1038/s41467-019-11152-6
201. Shi Y, Xu M, Pan S, Gao S, Ren J, Bai R, et al. Induction of the apoptosis, degranulation and IL-13 production of human basophils by butyrate and propionate via suppression of histone deacetylation. Immunology. (2021) 164(2):292–304. doi: 10.1111/imm.13370
202. Cario E, Gerken G, Podolsky DK. Toll-like receptor 2 enhances ZO-1-associated intestinal epithelial barrier integrity via protein kinase C. Gastroenterology. (2004) 127(1):224–38. doi: 10.1053/j.gastro.2004.04.015
203. Harrison OJ, Srinivasan N, Pott J, Schiering C, Krausgruber T, Ilott NE, et al. Epithelial-derived IL-18 regulates Th17 cell differentiation and Foxp3⁺ treg cell function in the intestine. Mucosal Immunol. (2015) 8(6):1226–36. doi: 10.1038/mi.2015.13
204. Han H, Roan F, Johnston LK, Smith DE, Bryce PJ, Ziegler SF. IL-33 promotes gastrointestinal allergy in a TSLP-independent manner. Mucosal Immunol. (2018) 11(2):394–403. doi: 10.1038/mi.2017.61
205. Schmitz J, Owyang A, Oldham E, Song Y, Murphy E, McClanahan TK, et al. IL-33, an interleukin-1-like cytokine that signals via the IL-1 receptor-related protein ST2 and induces T helper type 2-associated cytokines. Immunity. (2005) 23:479–90. doi: 10.1016/j.immuni.2005.09.015
206. Khodoun MV, Tomar S, Tocker JE, Wang YH, Finkelman FD. Prevention of food allergy development and suppression of established food allergy by neutralization of thymic stromal lymphopoietin, IL-25, and IL-33. J Allergy Clin Immunol. (2018) 141(1):171–9. doi: 10.1016/j.jaci.2017.02.046
207. Geremia A, Arancibia-Carcamo CV. Innate lymphoid cells in intestinal inflammation. Front Immunol. (2017) 8:1296. doi: 10.3389/fimmu.2017.01296
208. Shah K, Ignacio A, McCoy KD, Harris NL. The emerging roles of eosinophils in mucosal homeostasis. Mucosal Immunol. (2020) 13(4):574–83. doi: 10.1038/s41385-020-0281-y
209. Zheng Y, Valdez PA, Danilenko DM, Hu Y, Sa SM, Gong Q, et al. Interleukin-22 mediates early host defense against attaching and effacing bacterial pathogens. Nat Med. (2008) 14(3):282–9. doi: 10.1038/nm1720
210. Rescigno M, Rotta G, Valzasina B, Ricciardi-Castagnoli P. Dendritic cells shuttle microbes across gut epithelial monolayers. Immunobiology. (2001) 204(5):572–81. doi: 10.1078/0171-2985-00094
211. Rescigno M, Urbano M, Valzasina B, Francolini M, Rotta G, Bonasio R, et al. Dendritic cells express tight junction proteins and penetrate gut epithelial monolayers to sample bacteria. Nat Immunol. (2001) 2:361–7. doi: 10.1038/86373
212. Coombes JL, Siddiqui KRR, Arancibia-Cárcamo CV, Hall J, Sun CM, Belkaid Y, et al. A functionally specialized population of mucosal CD103+ DCs induces Foxp3 + regulatory T cells via a TGF-β -and retinoic acid-dependent mechanism. J Exp Med. (2007) 204:1757–64. doi: 10.1084/jem.20070590
213. Iwata M, Hirakiyama A, Eshima Y, Kagechika H, Kato C, Song SY. Retinoic acid imprints gut-homing specificity on T cells. Immunity. (2004) 21:527–38. doi: 10.1016/j.immuni.2004.08.011
214. Cassani B, Villablanca EJ, Quintana FJ, Love PE, Lacy-Hulbert A, Blaner WS, et al. Gut-tropic T cells that express integrin α4β7 and CCR9 are required for induction of oral immune tolerance in mice. Gastroenterology. (2011) 141(6):2019–18. doi: 10.1053/j.gastro.2011.09.015
215. Maslowski KM, Vieira AT, Ng A, Kranich J, Sierro F, Yu D, et al. Regulation of inflammatory responses by gut microbiota and chemoattractant receptor GPR43. Nature. (2009) 461(7268):1282–6. doi: 10.1038/nature08530
216. Frati F, Salvatori C, Incorvaia C, Bellucci A, Di Cara G, Marcucci F, et al. The role of the microbiome in asthma: the gut(-)lung axis. Int J Mol Sci. (2018) 20(1):123. doi: 10.3390/ijms20010123
217. Trompette A, Pernot J, Perdijk O, Alqahtani RAA, Domingo JS, Camacho-Munoz D, et al. Gut-derived short-chain fatty acids modulate skin barrier integrity by promoting keratinocyte metabolism and differentiation. Mucosal Immunol. (2022) 15(5):908–26. doi: 10.1038/s41385-022-00524-9
218. Kleuskens MTA, Haasnoot ML, Herpers BM, Ampting M, Bredenoord AJ, Garssen J, et al. Butyrate and propionate restore interleukin 13-compromised esophageal epithelial barrier function. Allergy. (2022) 77(5):1510–21. doi: 10.1111/all.15069
219. Hevia A, Milani C, Lopez P, Donado CD, Cuervo A, Gonzalez S, et al. Allergic patients with long-term asthma display low levels of Bifidobacterium adolescentis. PLoS One. (2016) 11(2):e0147809. doi: 10.1371/journal.pone.0147809
220. Raftis EJ, Delday MI, Cowie P, McCluskey SM, Singh MD, Ettorre A, et al. Bifidobacterium breve MRx0004 protects against airway inflammation in a severe asthma model by suppressing both neutrophil and eosinophil lung infiltration. Sci Rep. (2018) 8(1):12024. doi: 10.1038/s41598-018-30448-z
221. Kalliomäki M, Kirjavainen P, Eerola E, Kero P, Salminen S, Isolauri E. Distinct patterns of neonatal gut microflora in infants in whom atopy was and was not developing. J Allergy Clin Immunol. (2001) 107(1):129–34. doi: 10.1067/mai.2001.111237
222. Mutius E, Braun-Fahrländer C, Karvonen AM, Kirjavainen PV, Pekkanen J, Dalphin JC, et al. High levels of butyrate and propionate in early life are associated with protection against atopy. Allergy. (2019) 74(4):799–809. doi: 10.1111/all.13660
223. Desch AN, Henson PM, Jakubzick CV. Pulmonary dendritic cell development and antigen acquisition. Immunol Res. (2013) 55(1-3):178–86. doi: 10.1007/s12026-012-8359-6
224. Kopf M, Schneider C, Nobs SP. The development and function of lung-resident macrophages and dendritic cells. Nat Immunol. (2015) 16(1):36–44. doi: 10.1038/ni.3052
225. Stiemsma LT, Arrieta MC, Dimitriu PA, Cheng J, Thorson L, Lefebvre DL, et al. Shifts in Lachnospira and Clostridium sp. In the 3-month stool microbiome are associated with preschool age asthma. Clin Sci (Lond). (2016) 130(23):2199–207. doi: 10.1042/CS20160349
226. Liao HY, Tao L, Zhao J, Qin J, Zeng GC, Cai SW, et al. Clostridium butyricum in combination with specific immunotherapy converts antigen-specific B cells to regulatory B cells in asthmatic patients. Sci Rep. (2016) 6:20481. doi: 10.1038/srep20481
227. Parada Venegas D, De la Fuente MK, Landskron G, González MJ, Quera R, Dijkstra G, et al. Short chain fatty acids (SCFAs)-mediated gut epithelial and immune regulation and its relevance for inflammatory bowel diseases. Front Immunol. (2019) 10:277. doi: 10.3389/fimmu.2019.00277
228. Liu H, Wang J, He T, Becker S, Zhang G, Li D, et al. Butyrate: a double-edged sword for health? Adv Nutr. (2018) 9(1):21–9. doi: 10.1093/advances/nmx009
229. Cummings JH, Pomare EW, Branch WJ, Naylor CP, Macfarlane GT. Short chain fatty acids in human large intestine, portal, hepatic and venous blood. Gut. (1987) 28(10):1221–7. doi: 10.1136/gut.28.10.1221
230. Bach Knudsen KE, Serena A, Canibe N, Juntunen KS. New insight into butyrate metabolism. Proc Nutr Soc. (2003) 62(1):81–6. doi: 10.1079/PNS2002212
231. Trompette A, Gollwitzer ES, Pattaroni C, Lopez-Mejia IC, Riva E, Pernot J, et al. Dietary fiber confers protection against flu by shaping Ly6c(-) patrolling monocyte hematopoiesis and CD8(+) T cell metabolism. Immunity. (2018) 48(5):992–1005.e8. doi: 10.1016/j.immuni.2018.04.022
232. Bertges M, van Helden J, Weiskirchen R. Quantification of short chain fatty acids (acetate, butyrate, propionate) in human blood with ion exclusion chromatography. Pract Lab Med. (2021) 26:e00244. doi: 10.1016/j.plabm.2021.e00244
233. Kaisar MM, Pelgrom LR, van der Ham AJ, Yazdanbakhsh M, Everts B. Butyrate conditions human dendritic cells to prime type 1 regulatory T cells via both histone deacetylase inhibition and G protein-coupled receptor 109A signaling. Front Immunol. (2017) 8:1429. doi: 10.3389/fimmu.2017.01429
234. Vieira RS, Castoldi A, Basso PJ, Hiyane MI, Câmara NOS, Almeida RR. Butyrate attenuates lung inflammation by negatively modulating Th9 cells. Front Immunol. (2019) 10:67. doi: 10.3389/fimmu.2019.00067
235. Arpaia N, Campbell C, Fan X, Dikiy S, Van Der Veeken J, Deroos P, et al. Metabolites produced by commensal bacteria promote peripheral regulatory T-cell generation. Nature. (2013) 504:451–5. doi: 10.1038/nature12726
236. Sanchez HN, Moroney JB, Gan H, Shen T, Im JL, Li T, et al. B cell-intrinsic epigenetic modulation of antibody responses by dietary fiber-derived short-chain fatty acids. Nat Commun. (2020) 11(1):60. doi: 10.1038/s41467-019-13603-6
237. Folkerts J, Redegeld F, Folkerts G, Blokhuis B, van den Berg MPM, de Bruijn MJW, et al. Butyrate inhibits human mast cell activation via epigenetic regulation of FcεRI-mediated signaling. Allergy. (2020) 75(8):1966–78. doi: 10.1111/all.14254
238. Thio CL, Chi PY, Lai AC, Chang YJ. Regulation of type 2 innate lymphoid cell-dependent airway hyperreactivity by butyrate. J Allergy Clin Immunol. (2018) 142(6):1867–83. doi: 10.1016/j.jaci.2018.02.032
239. Theiler A, Bärnthaler T, Platzer W, Richtig G, Peinhaupt M, Rittchen S, et al. Butyrate ameliorates allergic airway inflammation by limiting eosinophil trafficking and survival. J Allergy Clin Immunol. (2019) 144(3):764–76. doi: 10.1016/j.jaci.2019.05.002
240. Huang C, Du W, Ni Y, Lan G, Shi G. The effect of short-chain fatty acids on M2 macrophages polarization in vitro and in vivo. Clin Exp Immunol. (2022) 207(1):53–64. doi: 10.1093/cei/uxab028
241. Islam R, Dash D, Singh R. Intranasal curcumin and sodium butyrate modulates airway inflammation and fibrosis via HDAC inhibition in allergic asthma. Cytokine. (2022) 149:155720. doi: 10.1016/j.cyto.2021.155720
242. Zhang W, Zhang Q, Zhu Y, Zhang Y, Xia Y, Wei Z, et al. Rectal administration of butyrate ameliorates pulmonary fibrosis in mice through induction of hepatocyte growth factor in the colon via the HDAC-PPARgamma pathway. Life Sci. (2022) 309:120972. doi: 10.1016/j.lfs.2022.120972
Keywords: allergy, asthma, epithelial barrier, exposome, food allergy, gut-lung axis, microbiota, SCFAs
Citation: Parrón-Ballesteros J, Gordo RG, López-Rodríguez JC, Olmo N, Villalba M, Batanero E and Turnay J (2023) Beyond allergic progression: From molecules to microbes as barrier modulators in the gut-lung axis functionality. Front. Allergy 4:1093800. doi: 10.3389/falgy.2023.1093800
Received: 9 November 2022; Accepted: 10 January 2023;
Published: 30 January 2023.
Edited by:
Rajna Minic, University of Belgrade, SerbiaReviewed by:
Marie Bodinier, U1268 Biopolymères, Interactions, Assemblages (BIA), FranceHubert Dirven, Norwegian Institute of Public Health (NIPH), Norway
© 2023 Parrón-Ballesteros, Gordo, López-Rodríguez, Olmo, Villalba, Batanero and Turnay. This is an open-access article distributed under the terms of the Creative Commons Attribution License (CC BY). The use, distribution or reproduction in other forums is permitted, provided the original author(s) and the copyright owner(s) are credited and that the original publication in this journal is cited, in accordance with accepted academic practice. No use, distribution or reproduction is permitted which does not comply with these terms.
*Correspondence: Javier Turnay dHVybmF5QHVjbS5lcw==
†These authors have contributed equally to this work and share first authorship
Specialty Section: This article was submitted to Allergens, a section of the journal Frontiers in Allergy
Abbreviations AA, Arachidonic Acid; AD: Atopic Dermatitis; DCs, Dendritic Cells; DHA, DocosaHexanoic Acid; EPA, EicosaPentanoic Acid; FA, Food Allergies; GPCRs, G Protein-Coupled Receptors; IECs, Intestinal Epithelia Cells; IL, InterLeukin; ILC2, Type 2 Innate Lymphoid Cells; MP, MicroPlastic; OVA, OVAlbumin; PUFAs, PolyUnsaturated Fatty Acids; SCFAs, Short-Chain Fatty Acids; TJs, Tight Junctions; TLR, toll-like receptor; TSLP, Thymic Stromal Lymphopoietin; ZO-1, Zonula Occludens-1.