- INDOOR Biotechnologies Inc., Charlottesville, VA, United States
Genome engineering with clustered regularly interspaced short palindromic repeats (CRISPR) technology offers the unique potential for unequivocally deleting allergen genes at the source. Compared to prior gene editing approaches, CRISPR boasts substantial improvements in editing efficiency, throughput, and precision. CRISPR has demonstrated success in several clinical applications such as sickle cell disease and β-thalassemia, and preliminary knockout studies of allergenic proteins using CRISPR editing show promise. Given the advantages of CRISPR, as well as specific DNA targets in the allergen genes, CRISPR gene editing is a viable approach for tackling allergy, which may lead to significant disease improvement. This review will highlight recent applications of CRISPR editing of allergens, particularly cat allergen Fel d 1, and will discuss the advantages and limitations of this approach compared to existing treatment options.
Introduction
Allergic disease is a persistent clinical challenge with limited treatment options. Inhaled allergens, such as those derived from cat, pollen, or dust mite contribute to the development or exacerbation of IgE-mediated allergic rhinitis or asthma (1). While the prevalence of allergic rhinitis among young adults in developed countries has been found to range from 12 to 46% (2), treatment options are largely limited to allergen avoidance or medications that ease the allergic symptoms (e.g., antihistamines or corticosteroids). Targeted immunotherapies specific to pollen or dust mite allergens have recently proven effective for treating allergic rhinitis (3, 4). However, efficacy has not been demonstrated for many inhaled allergens and practical constraints such as treatment duration or expense limit the broad application of immunotherapy for the treatment of allergic rhinitis or asthma (1).
Food allergy may result in potentially fatal anaphylactic immune reactions and accounts for considerable annual healthcare costs (5). Previous studies indicate that an estimated 3–8% of children in the US suffer from food allergy, and suggest that the prevalence of food allergies has increased over time (6). Recent data support that early introduction to allergens is an effective strategy to prevent the onset of food allergy (7), whereas allergen avoidance may be necessary for disease management among sensitized individuals (8). Allergen immunotherapy strategies have been developed to prompt patient desensitization or tolerance in response to repeated food allergen exposure. Though allergen immunotherapy study data show promise, the requisite duration, safety, or maintenance of sustained immunotherapy treatments have not been fully elucidated (9).
Genome engineering, particularly CRISPR editing, offers the potential to effectively delete the allergen genes at the source, which may significantly benefit allergic individuals. This review will outline the advantages, limitations, and existing clinical applications of CRISPR technology. Several applications of CRISPR editing in allergy will be discussed, highlighting the value of the approach for engineering hypoallergenic food, developing allergen-free animal models, or determining the biologic function of allergen proteins. Though the therapeutic potential of CRISPR gene editing was only recently discovered, the technology will undoubtedly shape the evolution of disease management, and guide novel approaches for tackling allergic rhinitis or food allergy.
CRISPR Gene Editing
CRISPR Technology
The clustered regularly interspaced short palindromic repeats (CRISPR) systems function as a means of adaptive immunity in bacteria and archaea for the recognition and deletion of invading viral or plasmid DNA (10–14). CRISPR systems are comprised of small, modifiable guide RNAs (sgRNA) that direct CRISPR-associated (Cas) proteins to produce site-specific DNA double-stranded breaks (DSBs) (10). The well-known Cas9 nuclease, derived from Streptococcus pyogenes, simply and efficiently cleaves a precise DNA target sequence that is complementary to the accompanying sgRNA (15). Cas9-mediated DSBs are repaired by the cell through non-homologous end joining, an innate, yet imprecise process that introduces insertions or deletions (indels) in the target DNA that lead to frameshift mutations in the corresponding protein sequence (16). Alternatively, DSBs can be mended through homology directed repair, which requires a donor DNA template with flanking homology arms to guide the precise, indel-free repair (17).
Successful CRISPR editing of the target DNA sequence can be validated by several methods (18). Publicly available bioinformatics platforms can be employed to estimate CRISPR editing efficiency using sequence decomposition. Briefly, control and CRISPR-edited DNA chromatogram traces are uploaded to the platform, which then identifies all possible indels for the control trace and determines the relative abundance of those indels in the mixed trace of the edited sample (19, 20). CRISPR editing efficiency can also be estimated by enzymatic detection of base pair mismatches. Control and CRISPR-edited DNA fragments are PCR amplified around the predicted cut sites, and the resulting DNA duplexes are denatured and randomly re-annealed to form heteroduplex DNA. A mismatch-identifying enzyme (e.g., T7 endonuclease 1; T7E1) recognizes and cleaves the CRISPR-generated base pair mismatches, which are detected by gel electrophoresis and quantified by band densitometry (21, 22). Alternatively, targeted next-generation sequencing (NGS) can be used to assess CRISPR-mediated indel frequencies and subsequent editing efficiencies (18).
Advantages and Limitations of CRISPR
Unlike previous gene editing approaches such as homologous recombination, Cre-Lox, zinc-finger nucleases (ZFNs), or TALENs, CRISPR-Cas systems offer considerable advantages including improved target specificity, throughput, ease of use, and editing efficiency and precision (23, 24). CRISPR-Cas9 target specificity is determined by the 20 nucleotide sgRNA and a proximal protospacer adjacent motif (PAM) sequence (“NGG” for Cas9), which confers DNA target recognition by Cas9 (25). Multiple genomic sites may be targeted simultaneously with additional sgRNAs, which, together with Cas9 nuclease, are delivered to cells in vitro using traditional approaches such as electroporation or lipid-based transfection (15). CRISPR-Cas9 editing efficiencies may exceed 90% under ideal experimental conditions, though average efficiency may be ~40–50% at canonical NGG-adjacent target loci (26). The mean editing efficiency of CRISPR-Cas9 has been shown to be ~6 times greater than that of TALENs (15).
While CRISPR boasts enhanced target specificity compared to other gene editing technologies, the potential remains for off-target editing due to sufficient homology between the sgRNAs and off-target sequences in unintended genomic sites (27). To limit the possibility of off-target editing, guide design bioinformatics platforms predict off-targets by comparing the CRISPR sgRNA sequences with the whole genome of the species of interest (28). Alternatively, unbiased approaches for identifying CRISPR off-target sites, such as GUIDE-seq technology, can detect genome-wide DSBs for each sgRNA tested (29). The latest in vitro off-target prediction tools (e.g., CIRCLE-seq, SITE-seq, and Digenome-seq) allow for genome-wide as well as population-scale off-target profiling to account for genetic variants (30–32). If significant off-target editing is detected, wild-type CRISPR-Cas9 may be replaced with other CRISPR systems that offer improved on-target specificity with reduced off-target potential. These systems include Cas9 nickases that produce staggered DSBs from adjacent single-stranded cuts (33), as well as base or prime editors that convert specific nucleotide bases using catalytically impaired Cas9 fused to deaminase or reverse transcriptase (34–36). Alternatively, type V (e.g., Cas12) or type VI (e.g., Cas13) CRISPR systems may be utilized to produce staggered DNA DSBs or to directly target RNA, respectively (37, 38).
CRISPR in the Clinic
To date, CRISPR has demonstrated promise in several therapeutic applications, which will undoubtedly guide the development of novel approaches for disease management and treatment. For example, CRISPR-Cas9 was used to delete a mutation in the CEP290 gene that is responsible for Leber congenital amaurosis type 10 (LCA10), a severe form of retinal dystrophy that often stems from aberrant splicing due to a single point mutation (39, 40). CEP290-specific sgRNAs and Cas9 nuclease were delivered in viral vectors (adeno-associated virus, AAV) to non-human primate (NHP) photoreceptor cells using subretinal injection. CRISPR editing efficiencies of up to ~28% were observed for the treated NHPs, which exceeded the threshold of 10% functional rescue considered necessary to be clinically effective (39). Analogous CRISPR editing in human retinal explants followed by GUIDE-seq analysis found no evidence of off-target mutations. The strong preliminary data support the clinical investigation of the CRISPR-based therapeutic for treating CEP290-associated retinal disease (41).
Sickle cell disease (SCD) and transfusion-dependent β-thalassemia (TBT), two conditions of abnormal or insufficient erythrocytes resulting from mutations of the hemoglobin β subunit gene (HBB), were also targeted with CRISPR-Cas9 (42). Previously, single nucleotide polymorphisms (SNPs) in the BCL11A gene were found to correspond with increased fetal hemoglobin expression in adults, which subsequently correlated with reduced severity of SCD or TDT phenotypes (43, 44). Thus, to reactivate production of fetal hemoglobin, patients with SCD or TDT were infused with CRISPR-Cas9 edited hematopoietic stem and progenitor cells (HSPCs) that were mutated at the BCL11A locus. Preliminary data from two patients showed that the percentage of circulating erythrocytes expressing fetal hemoglobin increased from ~4 to >98% within 15 months of treatment (42). Regularly required disease-related transfusions were eliminated following treatment, and pre-clinical GUIDE-seq analyses found no evidence of off-target CRISPR editing (42).
The above applications underscore the value of CRISPR technology in tackling monogenic disorders, particularly those with relatively straightforward approaches for delivering treatments (e.g., direct subretinal injection or ex vivo blood cell editing). However, many CRISPR applications will require in situ editing of the relevant cells or tissues, which will ultimately necessitate more complex delivery methods or vehicles. Currently, in vivo delivery is predominantly limited to the use of viral vectors or lipid nanoparticles. Though viral vectors such as AAVs offer remarkable efficiency and specificity, the limited cargo capacity of the AAV genome inherently restricts the scope of potential applications (45). By contrast, delivering CRISPR reagents with nanoparticles may be constrained by potential toxicity concerns or inadequate targeting specificity (45). The current clinical applications of CRISPR editing, as well as the technological advances or limitations of those applications, will likely inform innovative treatments for many complex therapeutic targets including allergic disease.
CRISPR Gene Editing of Allergens
Editing the Major Cat Allergen, Fel d 1
Allergy to domestic cat (Felis catus, also known as Felis domesticus) affects 10–15% of adults and children, and may produce symptoms ranging in severity from rhinoconjunctivitis to asthma (46–48). Cat is the most common source of mammalian allergen, with high levels of the major cat allergen, Fel d 1, accumulating in house dust (10 → 1,000 μg/g dust) (49, 50). Roughly 95% of cat allergic patients produce IgE antibodies to Fel d 1, which accounts for 60–90% of total anti-cat IgE (51–55). Substantial exposure to Fel d 1 drives IgG4 antibody production in allergic and non-allergic individuals, and Fel d 1 is a prominent cause of Th2 immune responses (56). While several other cat allergens have been identified (e.g., Fel d 4), their allergenic and clinical significance has not been resolved (57, 58).
Fel d 1 is a tetrameric protein (35 kD) that is comprised of two heterodimers, each of which consists of two chains, chains 1 (70 AA, 8 kD) and 2 (92 AA, 10 kD) (59, 60). The genes, CH1 and CH2, encoding chain 1 and chain 2, respectively, are situated in a span of ~10,000 base pairs in the genome. The structure of recombinant Fel d 1 (PDB 2EJN) indicates that the protein binds Ca2+ ions and contains internal hydrophobic cavities that may bind steroid ligands (61). Fel d 1 is a secretoglobin that is similar in structure to uteroglobin proteins, and is produced by cat salivary, lachrymal, sebaceous, and perianal glands (62–65). On average, kittens produce less Fel d 1 than adult cats, and females produce lower levels of Fel d 1 compared to males (66).
The precise biologic function of Fel d 1 is unknown, though studies of homologous proteins suggest the allergen may be involved in epithelium defense, immune regulation, or chemical communication (67–71). One recent study noted the sequence homology and common structural features between Fel d 1 and a defensive toxin secreted by the brachial glands of the slow loris primate (67). In another study, the binding properties of Fel d 1 were found to mirror those of mouse androgen-binding protein (ABP), a structural homolog of Fel d 1 that is secreted in mouse saliva and is involved in mate selection and chemical communication among mice (71–74).
Most treatment options for cat allergy sufferers merely address the allergic symptoms, which may have limited impact on patient health and quality of life. Immunotherapy for cat allergen using extracts or Fel d 1 peptides has been investigated, but consistent improvement for all patients has not been achieved (75, 76). Several recent approaches to cat allergy aim to reduce Fel d 1 exposure. One group introduced anti-Fel d 1 polyclonal egg IgY antibody into cat food to reduce the cats' salivary allergen levels (77, 78). The treated cats showed a 47% reduction in haircoat Fel d 1 compared to baseline (78). Alternatively, the immunization of cats with an anti-Fel d 1 vaccine resulted in a ~50% reduction in allergen detected in cat tear extracts and a ~30% decrease in allergic patient symptom severity (79, 80). The purported threshold at which nearly all cat allergic patients experience symptoms is 8 μg of Fel d 1 per gram of house dust (48). Therefore, a 50% reduction in Fel d 1 expression in a home with moderate levels of Fel d 1 (~100 μg/g dust) would likely have negligible clinical effects.
Given that Fel d 1 is both an immunodominant allergen and a specific, well-defined genomic target, deleting Fel d 1 with CRISPR gene editing is a rational approach for tackling cat allergic disease. Recently, CRISPR technology was used to knockout the Fel d 1 genes in vitro (81–83). Genomic DNA was extracted from tissue samples of 50 domestic cats, and CH1 and CH2 were sequenced to identify conserved regions in the genes suitable for targeting with CRISPR (83). A panel of 10 sgRNAs targeted to either Fel d 1 chains 1 or 2 were evaluated. Each of the CRISPR sgRNAs along with Cas9 nuclease were delivered to immortalized feline kidney epithelial cells using lipid-based transfection. Fel d 1 gene knockout resulting from CRISPR-induced frameshift mutations was evaluated by DNA sequence decomposition or T7E1 mismatch detection for each sgRNA (Figure 1).
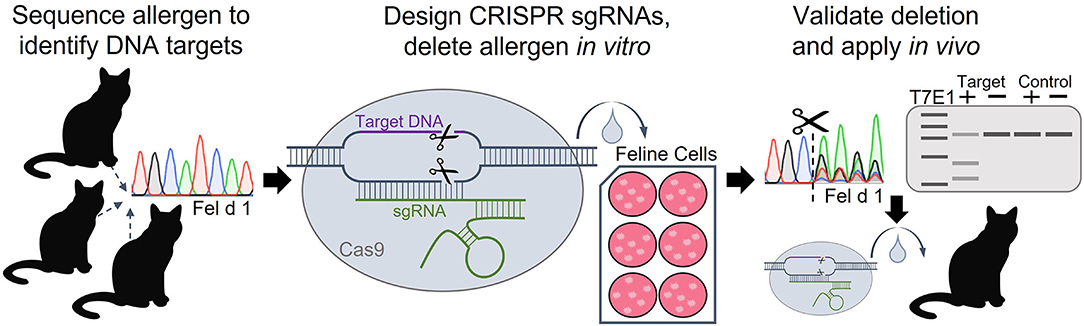
Figure 1. Workflow of experimental approach to delete the Fel d 1 genes using CRISPR-Cas9. Fel d 1 chains 1 and 2 were sequenced to identify conserved regions in the genes to target with CRISPR editing. Guide RNAs (sgRNAs) with sequences complementary to the conserved DNA target regions were designed and synthesized. The Fel d 1-specific sgRNAs and Cas9 nuclease were delivered to immortalized cat cells using lipid-based transfection. Successful in vitro editing, evaluated by DNA sequence decomposition and T7E1 (T7 endonuclease 1) mismatch detection, will guide future in vivo knockouts of Fel d 1.
Sequence decomposition determined CRISPR editing efficiencies ranging from 5 to 55% for each of the 10 Fel d 1-specific sgRNAs, while T7E1 analysis found editing efficiencies of 5–45% (83). Analyses of several predicted potential off-target cleavage sites found no evidence of off-target CRISPR editing due to the Fel d 1-specific sgRNAs. Future studies aim to replicate the work in Fel d 1-expressing primary feline cells to confirm protein expression knockout and, eventually, to apply the work in vivo in cats. These preliminary in vitro data indicate that Fel d 1 is a viable target for gene deletion using CRISPR and provide the first step in creating Fel d 1-free cats. Targeting the allergen with CRISPR technology is expected to substantially benefit cat allergic individuals by effectively removing Fel d 1 at the source, and may serve as the critical step in determining the definitive, biologic function of the allergen.
Editing Allergen Genes in Peanut
Allergy to peanut is one of the most severe food allergies and accounts for a significant proportion of food-induced allergic reactions that result in anaphylaxis (84, 85). The prevalence of peanut allergy among children in the US is ~2% but studies suggest this prevalence may be increasing (86, 87). While allergies to cow's milk or chicken egg proteins may resolve naturally during adolescence, allergy to peanut frequently persists into adulthood (88). Physical or chemical processes can be employed to reduce the allergenicity of peanuts and peanut products, however, avoidance or allergen immunotherapy [e.g., oral immunotherapy, Palforzia (89)] are recommended for sensitized individuals (90, 91). Several major peanut allergens have been identified including glycoprotein Ara h 2, which is recognized by IgE antibodies in more than 90% of peanut-allergic individuals (92, 93). Recently, peanut Ara h 2 was targeted using RNA interference (RNAi), a genetic engineering predecessor of CRISPR that knocks down gene expression at the mRNA level (94). An RNAi-expressing plasmid was delivered to peanut explants using Agrobacterium-mediated transformation, resulting in stable transgene integration in 44% of the plants. Seeds from the transgenic plants produced ~25% less Ara h 2 than control plants, and the IgE binding of peanut-allergic patient sera with the transgenic peanut samples was significantly reduced compared to wild type (94). The researchers propose transitioning from merely knocking down gene expression with RNAi to effectively deleting Ara h 2 and several other major peanut allergens using CRISPR (95).
Editing Egg White Proteins in Chickens
Allergy to hen's egg is one of the more prevalent food allergies, affecting up to ~2% of young children in industrialized regions (96). Though egg allergy has been shown to naturally resolve in ~50% of allergic children, egg allergen avoidance remains challenging (97). The majority of the allergenic egg proteins from domestic chicken (Gallus domesticus) are found in egg whites, including ovalbumin (Gal d 2) and ovomucoid (Gal d 1) (98). Recently, the genes (ovalbumin and ovomucoid) that code for the egg white proteins were targeted with CRISPR editing. The genes were knocked out in cultured chicken primordial germ cells using CRISPR-Cas9 with sgRNA editing efficiencies ranging from 13 to >90% (99). The ovomucoid-free primordial germ cells were transplanted into chicken embryos, resulting in homozygous ovomucoid knockouts among the second generation of offspring (99). Analyses of several predicted potential off-target sites found no evidence of off-target CRISPR mutations. Though the allergenicity of eggs produced by the ovomucoid knockout chickens was not determined, the study demonstrates proof-of-principle for using CRISPR-Cas9 to eliminate the major egg allergen proteins and to ultimately produce hypoallergenic eggs.
Editing Allergen Genes in Soybean
While soybean is an important food crop, several soy proteins are known to be major allergens. Allergies to soy-based protein formulas have been identified in ~0.5% of all children and up to 13% of children with other existing allergies (100). Given the value and abundance of soybean proteins that are increasingly used in food processing, gene editing offers a direct solution for developing hypoallergenic soybean products. Two soybean allergenic proteins include glycoprotein Gly m Bd 28 K (a Gly m 5 homolog) and oil-body-associated protein Gly m Bd 30 K (a cysteine protease), neither protein listed in the official WHO/IUIS Allergen Nomenclature database (101, 102). A recent study used CRISPR-Cas9 coupled with Agrobacterium-mediated transformation to simultaneously knockout the genes that code for these proteins in two varieties of soybean plants (103). Second and third generation soybean seeds exhibited indels at both target loci, including several deletions that produced frame-shift mutations and subsequently reduced protein expression and accumulation in the seeds (103). While Gly m Bd 30 K has been removed successfully from soy milk using pH-based protein fractionation (104), the CRISPR knockouts demonstrate proof-of-principle for the development of hypoallergenic soybean plants.
Editing Allergen Genes in Wheat
Wheat is a staple food crop and a key element of human nutrition. Wheat grains are comprised of a broad spectrum of protein families, including the α-gliadin gluten proteins, which are primarily responsible for the development of celiac disease and gluten sensitivity (105). The α-gliadin genes contain several conserved stimulatory peptides including an immunodominant 33-mer peptide, which was targeted with CRISPR-Cas9 in polyploid bread and durum (pasta) wheat cultivars (106). Twenty-one transgenic wheat lines were produced, with CRISPR editing efficiencies of up to 75% detected (106). Gluten content or immunoreactivity of the edited lines was reduced by up to 85%, and no off-target mutations were observed at predicted potential off-target sites (106). Beyond gluten proteins, protective or metabolic proteins such as α-amylase/trypsin inhibitors (ATIs) contribute to the development of wheat allergies (107). CM3 and CM16, ATI subunit proteins shown to produce strong IgE reactivity, were targeted with CRISPR-Cas9 in durum wheat (108). Fourteen of 97 regenerated plants exhibited CRISPR edits in the CM3 or CM16 genes, which were evaluated by sequencing and biochemical analyses (108). Taken together, these studies demonstrate the value of high-throughput CRISPR editing for the development of novel wheat varieties with reduced immunogenic profiles. Though the polyploid nature of wheat poses the additional challenge of simultaneously targeting several alleles or gene copies to achieve a functional knockout, the enhanced efficiency and versatility of CRISPR systems will certainly improve the targeted editing of polyploid genomes compared to traditional breeding approaches (109).
Editing β-Lactoglobulin in Cow's and Goat's Milk
Allergy to milk is the most common childhood food allergy, with an estimated 3% of infants experiencing adverse reactions to cow's milk proteins (110). The major cow's milk allergens include caseins and β-lactoglobulin (BLG, also known as Bos d 5), though several other minor allergen proteins have been identified (111). β-lactoglobulin, the primary component of milk whey proteins, is a particularly important allergen given its absence from human milk. Several groups have applied gene editing technology to produce BLG-free cows and goats. A BLG gene knockout cow generated using ZFN technology, a predecessor of CRISPR, produced BLG-free milk that resulted in significantly less IgE binding in cow's milk allergic individuals compared to wild type (112). Whole genome sequencing found no off-target effects due to the BLG-specific ZFN mRNA, while PCR confirmed that the BLG mutation is stably passed to offspring through germline transmission (112). Similarly, CRISPR-Cas9 was used to generate BLG knockout goats. Three BLG-specific CRISPR sgRNAs were co-injected with Cas9 mRNA into goat embryos, resulting in editing efficiencies of ~25% (113). The BLG-knockout goats produced significantly less BLG protein in milk, and no off-target editing was detected at predicted potential loci (113). These hypoallergenic milk studies highlight the value and therapeutic potential of applying genome editing technology to livestock for the benefit of human health.
Summary
CRISPR editing has shown promise in numerous applications of allergy research (Figure 2). These studies demonstrate the value of the technology in improving our understanding of allergen proteins, and underscore the vast potential for CRISPR editing to provide better, alternative treatment options for allergic disease. The applications highlighted in this review illustrate how CRISPR may be used to determine allergen protein function, engineer hypoallergenic foods, or develop allergen-free animals. In the future, CRISPR technology could also be employed for the identification of novel allergens, or for modifying the immune response directly as an approach to prevent recognition of allergen proteins. Moving forward, comprehensive analyses of allergen protein sequences, structures, or antibody binding sites will be invaluable for identifying essential functional domains or conserved sequences to target with CRISPR deletion. Additionally, further development of methods for the targeted delivery of CRISPR reagents to specific cells or tissues in vivo will prove vital for successfully editing the allergen genes in adult animals. While the value of CRISPR gene editing as a revolutionary therapeutic approach has only recently been established, the technology is poised to transform the management and treatment of allergic disease.
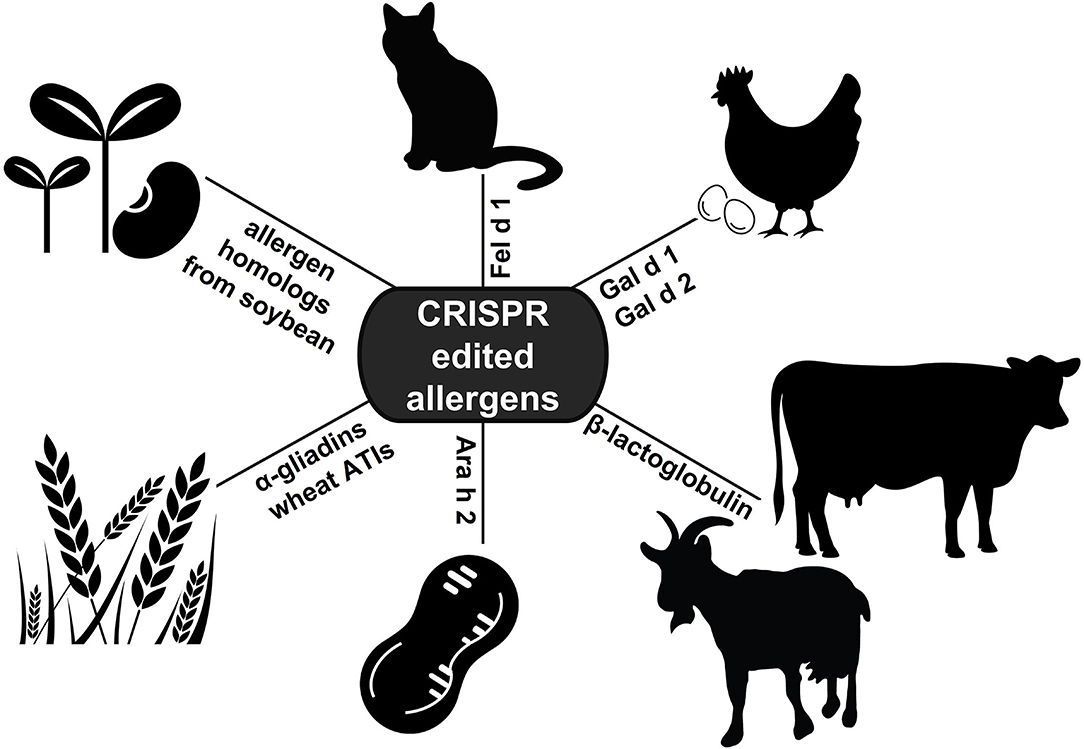
Figure 2. Applications of CRISPR editing in allergy research. To date, CRISPR technology has been applied to edit allergen genes in cat, hen's egg, soybean, wheat, peanut, and cow's and goat's milk (ATIs: α-amylase/trypsin inhibitors).
Author Contributions
NB wrote the manuscript. All of the authors critically reviewed and revised the manuscript.
Funding
This study received funding from INDOOR Biotechnologies Inc.
Conflict of Interest
MC is a co-owner and founder of INDOOR Biotechnologies Inc. NB and AP are employees of INDOOR Biotechnologies Inc.
Publisher's Note
All claims expressed in this article are solely those of the authors and do not necessarily represent those of their affiliated organizations, or those of the publisher, the editors and the reviewers. Any product that may be evaluated in this article, or claim that may be made by its manufacturer, is not guaranteed or endorsed by the publisher.
References
1. Bousquet J, Anto JM, Bachert C, Baiardini I, Bosnic-Anticevich S, Walter Canonica G, et al. Allergic rhinitis. Nat Rev Dis Primers. (2020) 6:95. doi: 10.1038/s41572-020-00227-0
2. Bousquet PJ, Leynaert B, Neukirch F, Sunyer J, Janson CM, Anto J, et al. Geographical distribution of atopic rhinitis in the European Community Respiratory Health Survey I. Allergy. (2008) 63:1301–9. doi: 10.1111/j.1398-9995.2008.01824.x
3. Gotoh M, Yonekura S, Imai T, Kaneko S, Horikawa E, Konno A, et al. Long-term efficacy and dose-finding trial of Japanese Cedar Pollen sublingual immunotherapy tablet. J Allergy Clin Immunol Pract. (2019) 7:1287–97 e8. doi: 10.1016/j.jaip.2018.11.044
4. Virchow JC, Backer V, Kuna P, Prieto L, Nolte H, Villesen HH, et al. Efficacy of a house dust mite sublingual allergen immunotherapy tablet in adults with allergic asthma: a randomized clinical trial. J Am Med Assoc. (2016) 315:1715–25. doi: 10.1001/jama.2016.3964
5. Gupta R, Holdford D, Bilaver L, Dyer A, Holl JL, Meltzer D. The economic impact of childhood food allergy in the United States. J Am Med Assoc Pediatr. (2013) 167:1026–31. doi: 10.1001/jamapediatrics.2013.2376
6. Yu W, Freeland DMH, Nadeau KC. Food allergy: immune mechanisms, diagnosis and immunotherapy. Nat Rev Immunol. (2016) 16:751–65. doi: 10.1038/nri.2016.111
7. du Toit G, Sayre PH, Roberts G, Lawson K, Sever ML, Bahnson HT, et al. Allergen specificity of early peanut consumption and effect on development of allergic disease in the Learning Early About Peanut Allergy study cohort. J Allergy Clin Immunol. (2018) 141:1343–53. doi: 10.1016/j.jaci.2017.09.034
8. Sicherer SH, Sampson HA. Food allergy: a review and update on epidemiology, pathogenesis, diagnosis, prevention, and management. J Allergy Clin Immunol. (2018) 141:41–58. doi: 10.1016/j.jaci.2017.11.003
9. Burks AW, Sampson HA, Plaut M, Lack G, Akdis CA. Treatment for food allergy. J Allergy Clin Immunol. (2018) 141:1–9. doi: 10.1016/j.jaci.2017.11.004
10. Jinek M, Chylinski K, Fonfara I, Hauer M, Doudna JA, Charpentier E, et al. Programmable dual-RNA-guided DNA endonuclease in adaptive bacterial immunity. Science. (2012) 337:816–21. doi: 10.1126/science.1225829
11. Wiedenheft B, Sternberg SH, Doudna JA. RNA-guided genetic silencing systems in bacteria and archaea. Nature. (2012) 482:331–8. doi: 10.1038/nature10886
12. Barrangou R, Fremaux C, Deveau H, Richards M, Boyaval P, Moineau S, et al. CRISPR provides acquired resistance against viruses in prokaryotes. Science. (2007) 315:1709–12. doi: 10.1126/science.1138140
13. Horvath P, Barrangou R. CRISPR/Cas, the immune system of bacteria and archaea. Science. (2010) 327:167–70. doi: 10.1126/science.1179555
14. Fineran PC, Charpentier E. Memory of viral infections by CRISPR-Cas adaptive immune systems: acquisition of new information. Virology. (2012) 434:202–9. doi: 10.1016/j.virol.2012.10.003
15. Cong L, Ran FA, Cox D, Lin S, Barretto R, Habib N, et al. Multiplex genome engineering using CRISPR/Cas systems. Science. (2013) 339:819–23. doi: 10.1126/science.1231143
16. Lieber MR. The mechanism of double-strand DNA break repair by the nonhomologous DNA end-joining pathway. Annu Rev Biochem. (2010) 79:181–211. doi: 10.1146/annurev.biochem.052308.093131
17. San Filippo J, Sung P, Klein H. Mechanism of eukaryotic homologous recombination. Annu Rev Biochem. (2008) 77:229–57. doi: 10.1146/annurev.biochem.77.061306.125255
18. Sentmanat MF, Peters ST, Florian CP, Connelly JP, Pruett-Miller SM. A survey of validation strategies for CRISPR-Cas9 editing. Sci Rep. (2018) 8:888. doi: 10.1038/s41598-018-19441-8
19. Brinkman EK, Chen T, Amendola M, van Steensel B. Easy quantitative assessment of genome editing by sequence trace decomposition. Nucleic Acids Res. (2014) 42:e168. doi: 10.1093/nar/gku936
20. Brinkman EK, van Steensel B. Rapid quantitative evaluation of CRISPR genome editing by TIDE and TIDER. Methods Mol Biol. (2019) 1961:29–44. doi: 10.1007/978-1-4939-9170-9_3
21. Mashal RD, Koontz J, Sklar J. Detection of mutations by cleavage of DNA heteroduplexes with bacteriophage resolvases. Nat Genet. (1995) 9:177–83. doi: 10.1038/ng0295-177
22. Vouillot L, Thelie A, Pollet N. Comparison of T7E1 and surveyor mismatch cleavage assays to detect mutations triggered by engineered nucleases. G3. (2015) 5:407–15. doi: 10.1534/g3.114.015834
23. Sander JD, Joung JK. CRISPR-Cas systems for editing, regulating and targeting genomes. Nat Biotechnol. (2014) 32:347–55. doi: 10.1038/nbt.2842
24. Chakrabarti AM, Henser-Brownhill T, Monserrat J, Poetsch AR, Luscombe NM, Scaffidi P. Target-specific precision of CRISPR-mediated genome editing. Mol Cell. (2019) 73:699–713 e6. doi: 10.1016/j.molcel.2018.11.031
25. Jiang F, Doudna JA. CRISPR-Cas9 structures and mechanisms. Annu Rev Biophys. (2017) 46:505–29. doi: 10.1146/annurev-biophys-062215-010822
26. Hu JH, Miller SM, Geurts MH, Tang W, Chen L, Sun N, et al. Evolved Cas9 variants with broad PAM compatibility and high DNA specificity. Nature. (2018) 556:57–63. doi: 10.1038/nature26155
27. Tsai SQ, Joung JK. Defining and improving the genome-wide specificities of CRISPR-Cas9 nucleases. Nat Rev Genet. (2016) 17:300–12. doi: 10.1038/nrg.2016.28
28. Haeussler M, Schonig K, Eckert H, Eschstruth A, Mianne J, Renaud JB, et al. Evaluation of off-target and on-target scoring algorithms and integration into the guide RNA selection tool CRISPOR. Genome Biol. (2016) 17:148. doi: 10.1186/s13059-016-1012-2
29. Tsai SQ, Zheng Z, Nguyen NT, Liebers M, Topkar VV, Thapar V, et al. GUIDE-seq enables genome-wide profiling of off-target cleavage by CRISPR-Cas nucleases. Nat Biotechnol. (2015) 33:187–97. doi: 10.1038/nbt.3117
30. Tsai SQ, Nguyen NT, Malagon-Lopez J, Topkar VV, Aryee MJ, Joung JK. CIRCLE-seq: a highly sensitive in vitro screen for genome-wide CRISPR-Cas9 nuclease off-targets. Nat Methods. (2017) 14:607–14. doi: 10.1038/nmeth.4278
31. Cameron P, Fuller CK, Donohoue PD, Jones BN, Thompson MS, Carter MM, et al. Mapping the genomic landscape of CRISPR-Cas9 cleavage. Nat Methods. (2017) 14:600–6. doi: 10.1038/nmeth.4284
32. Kim D, Bae S, Park J, Kim E, Kim S, Yu HR, et al. Digenome-seq: genome-wide profiling of CRISPR-Cas9 off-target effects in human cells. Nat Methods. (2015) 12:237–43. doi: 10.1038/nmeth.3284
33. Ran FA, Hsu PD, Lin CY, Gootenberg JS, Konermann S, Trevino AE, et al. Double nicking by RNA-guided CRISPR Cas9 for enhanced genome editing specificity. Cell. (2013) 154:1380–9. doi: 10.1016/j.cell.2013.09.040
34. Komor AC, Kim YB, Packer MS, Zuris JA, Liu DR. Programmable editing of a target base in genomic DNA without double-stranded DNA cleavage. Nature. (2016) 533:420–4. doi: 10.1038/nature17946
35. Gaudelli NM, Komor AC, Rees HA, Packer MS, Badran AH, Bryson DI, et al. Programmable base editing of A*T to G*C in genomic DNA without DNA cleavage. Nature. (2017) 551:464–71. doi: 10.1038/nature24644
36. Anzalone AV, Randolph PB, Davis JR, Sousa AA, Koblan LW, Levy JM, et al. Search-and-replace genome editing without double-strand breaks or donor DNA. Nature. (2019) 576:149–57. doi: 10.1038/s41586-019-1711-4
37. Yan WX, Hunnewell P, Alfonse LE, Carte JM, Keston-Smith E, Sothiselvam S, et al. Functionally diverse type V CRISPR-Cas systems. Science. (2019) 363:88–91. doi: 10.1126/science.aav7271
38. Abudayyeh OO, Gootenberg JS, Essletzbichler P, Han S, Joung J, Belanto JJ, et al. RNA targeting with CRISPR-Cas13. Nature. (2017) 550:280–4. doi: 10.1038/nature24049
39. Maeder ML, Stefanidakis M, Wilson CJ, Baral R, Barrera LA, Bounoutas GS, et al. Development of a gene-editing approach to restore vision loss in Leber congenital amaurosis type 10. Nat Med. (2019) 25:229–33. doi: 10.1038/s41591-018-0327-9
40. den Hollander AI, Koenekoop RK, Yzer S, Lopez I, Arends ML, Voesenek KE, et al. Mutations in the CEP290 (NPHP6) gene are a frequent cause of Leber congenital amaurosis. Am J Hum Genet. (2006) 79:556–61. doi: 10.1086/507318
41. Editas Medicine Inc. Single Ascending Dose Study in Participants With LCA10. ClinicalTrials.gov Identifier NCT03872479. Cambridge, MA (2019).
42. Frangoul H, Altshuler D, Cappellini MD, Chen YS, Domm J, Eustace BK, et al. CRISPR-Cas9 gene editing for sickle cell disease and beta-thalassemia. N Engl J Med. (2021) 384:252–60. doi: 10.1056/NEJMoa2031054
43. Bauer DE, Kamran SC, Lessard S, Xu J, Fujiwara Y, Lin C, et al. An erythroid enhancer of BCL11A subject to genetic variation determines fetal hemoglobin level. Science. (2013) 342:253–7. doi: 10.1126/science.1242088
44. Uda M, Galanello R, Sanna S, Lettre G, Sankaran VG, Chen W, et al. Genome-wide association study shows BCL11A associated with persistent fetal hemoglobin and amelioration of the phenotype of beta-thalassemia. Proc Natl Acad Sci USA. (2008) 105:1620–5. doi: 10.1073/pnas.0711566105
45. Doudna JA. The promise and challenge of therapeutic genome editing. Nature. (2020) 578:229–36. doi: 10.1038/s41586-020-1978-5
46. Ingram JM, Sporik R, Rose G, Honsinger R, Chapman MD, Platts-Mills TA. Quantitative assessment of exposure to dog (Can f 1) and cat (Fel d 1) allergens: relation to sensitization and asthma among children living in Los Alamos, New Mexico. J Allergy Clin Immunol. (1995) 96:449–56. doi: 10.1016/S0091-6749(95)70286-5
47. Perzanowski MS, Ronmark E, Nold B, Lundback B, Platts-Mills TA. Relevance of allergens from cats and dogs to asthma in the northernmost province of Sweden: schools as a major site of exposure. J Allergy Clin Immunol. (1999) 103:1018–24. doi: 10.1016/S0091-6749(99)70173-9
48. Gelber LE, Seltzer LH, Bouzoukis JK, Pollart SM, Chapman MD, Platts-Mills TA. Sensitization and exposure to indoor allergens as risk factors for asthma among patients presenting to hospital. Am Rev Respir Dis. (1993) 147:573–8. doi: 10.1164/ajrccm/147.3.573
49. Platts-Mills TA, Vervloet D, Thomas WR, Aalberse RC, Chapman MD. Indoor allergens and asthma: report of the Third International Workshop. J Allergy Clin Immunol. (1997) 100:S2–24. doi: 10.1016/S0091-6749(97)70292-6
50. Chapman MD, Wood RA. The role and remediation of animal allergens in allergic diseases. J Allergy Clin Immunol. (2001) 107:S414–21. doi: 10.1067/mai.2001.113672
51. Ohman JL Jr, Marsh DG, Goldman M. Antibody responses following immunotherapy with cat pelt extract. J Allergy Clin Immunol. (1982) 69:320–6. doi: 10.1016/S0091-6749(82)80010-9
52. Lowenstein H, Lind P, Weeke B. Identification and clinical significance of allergenic molecules of cat origin. Part of the DAS 76. Study Allergy. (1985) 40:430–41. doi: 10.1111/j.1398-9995.1985.tb02682.x
53. de Groot H, van Swieten P, van Leeuwen J, Lind P, Aalberse RC. Monoclonal antibodies to the major feline allergen Fel d I. I Serologic and biologic activity of affinity-purified Fel d I and of Fel d I-depleted extract. J Allergy Clin Immunol. (1988) 82:778–86. doi: 10.1016/0091-6749(88)90079-6
54. Chapman MD, Aalberse RC, Brown MJ, Platts-Mills TA. Monoclonal antibodies to the major feline allergen Fel d I. II Single step affinity purification of Fel d I, N-terminal sequence analysis, and development of a sensitive two-site immunoassay to assess Fel d I exposure. J Immunol. (1988) 140:812–8.
55. van Ree R, van Leeuwen WA, Bulder I, Bond J, Aalberse RC. Purified natural and recombinant Fel d 1 and cat albumin in in vitro diagnostics for cat allergy. J Allergy Clin Immunol. (1999) 104:1223–30. doi: 10.1016/S0091-6749(99)70017-5
56. Platts-Mills T, Vaughan J, Squillace S, Woodfolk J, Sporik R. Sensitisation, asthma, and a modified Th2 response in children exposed to cat allergen: a population-based cross-sectional study. Lancet. (2001) 357:752–6. doi: 10.1016/S0140-6736(00)04168-4
57. Ukleja-Sokolowska N, Gawronska-Ukleja E, Zbikowska-Gotz M, Socha E, Lis K, Sokolowski L, et al. Analysis of feline and canine allergen components in patients sensitized to pets. Allergy Asthma Clin Immunol. (2016) 12:61. doi: 10.1186/s13223-016-0167-4
58. Smith W, Butler AJ, Hazell LA, Chapman MD, Pomés A, Nickels DG, et al. Fel d 4, a cat lipocalin allergen. Clin Exp Allergy. (2004) 34:1732–8. doi: 10.1111/j.1365-2222.2004.02090.x
59. Morgenstern JP, Griffith IJ, Brauer AW, Rogers BL, Bond JF, Chapman MD, et al. Amino acid sequence of Fel dI, the major allergen of the domestic cat: protein sequence analysis and cDNA cloning. Proc Natl Acad Sci USA. (1991) 88:9690–4. doi: 10.1073/pnas.88.21.9690
60. Griffith IJ, Craig S, Pollock J, Yu XB, Morgenstern JP, Rogers BL. Expression and genomic structure of the genes encoding FdI, the major allergen from the domestic cat. Gene. (1992) 113:263–8. doi: 10.1016/0378-1119(92)90405-E
61. Kaiser L, Velickovic TC, Badia-Martinez D, Adedoyin J, Thunberg S, Hallen D, et al. Structural characterization of the tetrameric form of the major cat allergen Fel d 1. J Mol Biol. (2007) 370:714–27. doi: 10.1016/j.jmb.2007.04.074
62. Kaiser L, Gronlund H, Sandalova T, Ljunggren HG, van Hage-Hamsten M, Achour A, et al. The crystal structure of the major cat allergen Fel d 1, a member of the secretoglobin family. J Biol Chem. (2003) 278:37730–5. doi: 10.1074/jbc.M304740200
63. van Milligen FJ, Vroom TM, Aalberse RC. Presence of Felis domesticus allergen I in the cat's salivary and lacrimal glands. Int Arch Allergy Appl Immunol. (1990) 92:375–8. doi: 10.1159/000235168
64. Charpin C, Mata P, Charpin D, Lavaut MN, Allasia C, Vervloet D. Fel d I allergen distribution in cat fur and skin. J Allergy Clin Immunol. (1991) 88:77–82. doi: 10.1016/0091-6749(91)90303-6
65. De Andrade AD, Birnbaum J, Magalon C, Magnol JP, Lanteaume A, Charpin D, et al. Fel d I levels in cat anal glands. Clin Exp Allergy. (1996) 26:178–80. doi: 10.1111/j.1365-2222.1996.tb00077.x
66. Charpin C, Zielonka TM, Charpin D, Ansaldi JL, Allasia C, Vervloet D. Effects of castration and testosterone on Fel dI production by sebaceous glands of male cats: II–Morphometric assessment. Clin Exp Allergy. (1994) 24:1174–8. doi: 10.1111/j.1365-2222.1994.tb03325.x
67. Scheib H, Nekaris KA, Rode-Margono J, Ragnarsson L, Baumann K, Dobson JS, et al. The toxicological intersection between allergen and toxin: a structural comparison of the cat dander allergenic protein Fel d1 and the slow loris brachial gland secretion protein. Toxins. (2020) 12:20086. doi: 10.3390/toxins12020086
68. Ring PC, Wan H, Schou C, Kroll Kristensen A, Roepstorff P, Robinson C. The 18-kDa form of cat allergen Felis domesticus 1 (Fel d 1) is associated with gelatin- and fibronectin-degrading activity. Clin Exp Allergy. (2000) 30:1085–96. doi: 10.1046/j.1365-2222.2000.00805.x
69. Emara M, Royer PJ, Abbas Z, Sewell HF, Mohamed GG, Singh S, et al. Recognition of the major cat allergen Fel d 1 through the cysteine-rich domain of the mannose receptor determines its allergenicity. J Biol Chem. (2011) 286:13033–40. doi: 10.1074/jbc.M111.220657
70. Herre J, Gronlund H, Brooks H, Hopkins L, Waggoner L, Murton B, et al. Allergens as immunomodulatory proteins: the cat dander protein Fel d 1 enhances TLR activation by lipid ligands. J Immunol. (2013) 191:1529–35. doi: 10.4049/jimmunol.1300284
71. Durairaj R, Pageat P, Bienboire-Frosini C. Another cat and mouse game: deciphering the evolution of the SCGB superfamily and exploring the molecular similarity of major cat allergen Fel d 1 and mouse ABP using computational approaches. PLoS ONE. (2018) 13:e0197618. doi: 10.1371/journal.pone.0197618
72. Bienboire-Frosini C, Durairaj R, Pelosi P, Pageat P. The major cat allergen Fel d 1 binds steroid and fatty acid semiochemicals: a combined in silico and in vitro study. Int J Mol Sci. (2020) 21:41365. doi: 10.3390/ijms21041365
73. Karn RC. The mouse salivary androgen-binding protein (ABP) alpha subunit closely resembles chain 1 of the cat allergen Fel dI. Biochem Genet. (1994) 32:271–7. doi: 10.1007/BF00555830
74. Chung AG, Belone PM, Bimova BV, Karn RC, Laukaitis CM. Studies of an androgen-binding protein knockout corroborate a role for salivary ABP in mouse communication. Genetics. (2017) 205:1517–27. doi: 10.1534/genetics.116.194571
75. Dhami S, Agarwal A. Does evidence support the use of cat allergen immunotherapy? Curr Opin Allergy Clin Immunol. (2018) 18:350–5. doi: 10.1097/ACI.0000000000000457
76. Patel D, Couroux P, Hickey P, Salapatek AM, Laidler P, Larche M, et al. Fel d 1-derived peptide antigen desensitization shows a persistent treatment effect 1 year after the start of dosing: a randomized, placebo-controlled study. J Allergy Clin Immunol. (2013) 131:103–9 e1-7. doi: 10.1016/j.jaci.2012.07.028
77. Satyaraj E, Li Q, Sun P, Sherrill S. Anti-Fel d1 immunoglobulin Y antibody-containing egg ingredient lowers allergen levels in cat saliva. J Feline Med Surg. (2019) 21:875–81. doi: 10.1177/1098612X19861218
78. Satyaraj E, Gardner C, Filipi I, Cramer K, Sherrill S. Reduction of active Fel d1 from cats using an antiFel d1 egg IgY antibody. Immun Inflamm Dis. (2019) 7:68–73. doi: 10.1002/iid3.244
79. Thoms F, Jennings GT, Maudrich M, Vogel M, Haas S, Zeltins A, et al. Immunization of cats to induce neutralizing antibodies against Fel d 1, the major feline allergen in human subjects. J Allergy Clin Immunol. (2019) 144:193–203. doi: 10.1016/j.jaci.2019.01.050
80. Thoms F, Haas S, Erhart A, Nett CS, Rufenacht S, Graf N, et al. Immunization of cats against Fel d 1 results in reduced allergic symptoms of owners. Viruses. (2020) 12:30288. doi: 10.3390/v12030288
81. Brackett N, Riedy J, Adli M, Pomés A, Chapman M. Gene editing the major cat allergen, Fel d 1, using CRISPR-Cas9. J Allergy Clin Immunol. (2020) 145:AB156. doi: 10.1016/j.jaci.2019.12.405
82. Brackett N, Riedy J, Adli M, Pomés A, Chapman M. CRISPR gene editing of the major cat allergen, Fel d 1. Allergy. (2020) 75:59–60. doi: 10.1111/all.14506
83. Brackett N, Pomés A, Chapman M. The major cat allergen, Fel d 1, is a viable target for CRISPR gene editing. J Allergy Clin Immunol. (2021) 147:AB175. doi: 10.1016/j.jaci.2020.12.617
84. Sampson HA, Mendelson L, Rosen JP. Fatal and near-fatal anaphylactic reactions to food in children and adolescents. N Engl J Med. (1992) 327:380–4. doi: 10.1056/NEJM199208063270603
85. Sampson HA. Fatal food-induced anaphylaxis. Allergy. (1998) 53:125–30. doi: 10.1111/j.1398-9995.1998.tb04982.x
86. Bunyavanich S, Rifas-Shiman SL, Platts-Mills TA, Workman L, Sordillo JE, Gillman MW, et al. Peanut allergy prevalence among school-age children in a US cohort not selected for any disease. J Allergy Clin Immunol. (2014) 134:753–5. doi: 10.1016/j.jaci.2014.05.050
87. Sicherer SH, Munoz-Furlong A, Burks AW, Sampson HA. Prevalence of peanut and tree nut allergy in the US determined by a random digit dial telephone survey. J Allergy Clin Immunol. (1999) 103:559–62. doi: 10.1016/S0091-6749(99)70224-1
88. Bock SA, Atkins FM. The natural history of peanut allergy. J Allergy Clin Immunol. (1989) 83:900–4. doi: 10.1016/0091-6749(89)90103-6
89. Smith SS, Hilas O. Peanut (Arachis hypogaea) allergen powder-dnfp: the first FDA-approved oral immunotherapy for desensitization of peanut allergy in children. J Pediatr Pharmacol Ther. (2021) 26:669–74. doi: 10.5863/1551-6776-26.7.669
90. Zhou Y, Wang JS, Yang XJ, Lin DH, Gao YF, Su YJ, et al. Peanut allergy, allergen composition, and methods of reducing allergenicity: a review. Int J Food Sci. (2013) 2013:909140. doi: 10.1155/2013/909140
91. Pons L, Palmer K, Burks W. Towards immunotherapy for peanut allergy. Curr Opin Allergy Clin Immunol. (2005) 5:558–62. doi: 10.1097/01.all.0000191233.90136.21
92. Burks AW, Williams LW, Connaughton C, Cockrell G, O'Brien TJ, Helm RM. Identification and characterization of a second major peanut allergen, Ara h II, with use of the sera of patients with atopic dermatitis and positive peanut challenge. J Allergy Clin Immunol. (1992) 90:962–9. doi: 10.1016/0091-6749(92)90469-I
93. de Jong EC, Van Zijverden M, Spanhaak S, Koppelman SJ, Pellegrom H, Penninks AH. Identification and partial characterization of multiple major allergens in peanut proteins. Clin Exp Allergy. (1998) 28:743–51. doi: 10.1046/j.1365-2222.1998.00301.x
94. Dodo HW, Konan KN, Chen FC, Egnin M, Viquez OM. Alleviating peanut allergy using genetic engineering: the silencing of the immunodominant allergen Ara h 2 leads to its significant reduction and a decrease in peanut allergenicity. Plant Biotechnol J. (2008) 6:135–45. doi: 10.1111/j.1467-7652.2007.00292.x
95. Dodo H. SBIR Phase II: Development of an Allergen-Free Peanut Using Genome Editing Technology. Alexandria, VA: National Science Foundation (2021).
96. Xepapadaki P, Fiocchi A, Grabenhenrich L, Roberts G, Grimshaw KE, Fiandor A, et al. Incidence and natural history of hen's egg allergy in the first 2 years of life-the EuroPrevall birth cohort study. Allergy. (2016) 71:350–7. doi: 10.1111/all.12801
97. Sicherer SH, Wood RA, Vickery BP, Jones SM, Liu AH, Fleischer DM, et al. The natural history of egg allergy in an observational cohort. J Allergy Clin Immunol. (2014) 133:492–9. doi: 10.1016/j.jaci.2013.12.1041
98. Caubet JC, Wang J. Current understanding of egg allergy. Pediatr Clin North Am. (2011) 58:427–43. doi: 10.1016/j.pcl.2011.02.014
99. Oishi I, Yoshii K, Miyahara D, Kagami H, Tagami T. Targeted mutagenesis in chicken using CRISPR/Cas9 system. Sci Rep. (2016) 6:23980. doi: 10.1038/srep23980
100. Katz Y, Gutierrez-Castrellon P, Gonzalez MG, Rivas R, Lee BW, Alarcon P, et al. comprehensive review of sensitization and allergy to soy-based products. Clin Rev Allergy Immunol. (2014) 46:272–81. doi: 10.1007/s12016-013-8404-9
101. Tsuji H, Bando N, Hiemori M, Yamanishi R, Kimoto M, Nishikawa K, et al. Purification of characterization of soybean allergen Gly m Bd 28K. Biosci Biotechnol Biochem. (1997) 61:942–7. doi: 10.1271/bbb.61.942
102. Ogawa T, Tsuji H, Bando N, Kitamura K, Zhu YL, Hirano H, et al. Identification of the soybean allergenic protein, Gly m Bd 30K, with the soybean seed 34-kDa oil-body-associated protein. Biosci Biotechnol Biochem. (1993) 57:1030–3. doi: 10.1271/bbb.57.1030
103. Sugano S, Hirose A, Kanazashi Y, Adachi K, Hibara M, Itoh T, et al. Simultaneous induction of mutant alleles of two allergenic genes in soybean by using site-directed mutagenesis. BMC Plant Biol. (2020) 20:513. doi: 10.1186/s12870-020-02708-6
104. Samoto M, Akasaka T, Mori H, Manabe M, Ookura T, Kawamura Y. Simple and efficient procedure for removing the 34 kDa allergenic soybean protein, Gly m I, from defatted soy milk. Biosci Biotechnol Biochem. (1994) 58:2123–5. doi: 10.1271/bbb.58.2123
105. Sapone A, Lammers KM, Casolaro V, Cammarota M, Giuliano MT, De Rosa M, et al. Divergence of gut permeability and mucosal immune gene expression in two gluten-associated conditions: celiac disease and gluten sensitivity. BMC Med. (2011) 9:23. doi: 10.1186/1741-7015-9-23
106. Sanchez-Leon S, Gil-Humanes J, Ozuna CV, Gimenez MJ, Sousa C, Voytas DF, et al. Low-gluten, nontransgenic wheat engineered with CRISPR/Cas9. Plant Biotechnol J. (2018) 16:902–10. doi: 10.1111/pbi.12837
107. Bellinghausen I, Weigmann B, Zevallos V, Maxeiner J, Reissig S, Waisman A, et al. Wheat amylase-trypsin inhibitors exacerbate intestinal and airway allergic immune responses in humanized mice. J Allergy Clin Immunol. (2019) 143:201–12 e4. doi: 10.1016/j.jaci.2018.02.041
108. Camerlengo F, Frittelli A, Sparks C, Doherty A, Martignago D, Larré C, et al. CRISPR-Cas9 multiplex editing of the α-amylase/trypsin inhibitor genes to reduce allergen proteins in durum wheat. Front Sustain Food Syst. (2020) 4. doi: 10.3389/fsufs.2020.00104
109. Schaart JG, van de Wiel CCM, Smulders MJM. Genome editing of polyploid crops: prospects, achievements and bottlenecks. Transgenic Res. (2021) 30:337–51. doi: 10.1007/s11248-021-00251-0
110. Host A. Frequency of cow's milk allergy in childhood. Ann Allergy Asthma Immunol. (2002) 89:33–7. doi: 10.1016/S1081-1206(10)62120-5
111. Restani P, Ballabio C, Di Lorenzo C, Tripodi S, Fiocchi A. Molecular aspects of milk allergens and their role in clinical events. Anal Bioanal Chem. (2009) 395:47–56. doi: 10.1007/s00216-009-2909-3
112. Sun Z, Wang M, Han S, Ma S, Zou Z, Ding F, et al. Production of hypoallergenic milk from DNA-free beta-lactoglobulin (BLG) gene knockout cow using zinc-finger nucleases mRNA. Sci Rep. (2018) 8:15430. doi: 10.1038/s41598-018-32024-x
Keywords: CRISPR, gene editing, allergy, cat allergen, Fel d 1
Citation: Brackett NF, Pomés A and Chapman MD (2022) New Frontiers: Precise Editing of Allergen Genes Using CRISPR. Front. Allergy 2:821107. doi: 10.3389/falgy.2021.821107
Received: 23 November 2021; Accepted: 24 December 2021;
Published: 17 January 2022.
Edited by:
Rudolf Valenta, Medical University of Vienna, AustriaReviewed by:
Eva Stoger, University of Natural Resources and Life Sciences, AustriaDaniel P. Potaczek, University of Marburg, Germany
Copyright © 2022 Brackett, Pomés and Chapman. This is an open-access article distributed under the terms of the Creative Commons Attribution License (CC BY). The use, distribution or reproduction in other forums is permitted, provided the original author(s) and the copyright owner(s) are credited and that the original publication in this journal is cited, in accordance with accepted academic practice. No use, distribution or reproduction is permitted which does not comply with these terms.
*Correspondence: Nicole F. Brackett, bmJyYWNrZXR0QGluYmlvLmNvbQ==