- 1Laboratorio de Bioquímica, Facultad de Agronomía, Universidad de la República, Montevideo, Uruguay
- 2Laboratorio de Microbiología de Suelos, Facultad de Ciencias, Universidad de la República, Montevideo, Uruguay
- 3Laboratorio Microbiología Molecular, Departamento Bioquímica y Genómica Microbianas (BIOGEM), Instituto de Investigaciones Biológicas Clemente Estable (IIBCE), Montevideo, Uruguay
- 4Laboratorio de Genómica Microbiana, Instituto Pasteur, Montevideo, Uruguay
- 5Plant Energy Biology, School of Molecular Science, The University of Western Australia, Crawley, WA, Australia
In acid soils, Rhizobium favelukesii strains, known as Oregon-like strains, are a potential risk for alfalfa production given their parasitic behaviour. In this study, we isolated five parasitic strains (ORY1 to ORY5) from alfalfa nodules grown in Uruguayan acid soils, with a 99.7% and a 100% 16S rRNA gene sequence identity to R. favelukesii type strain of LUP83. The BOX profiles of the five isolates showed two different patterns, suggesting some diversity among these acid-tolerant isolates. The genome sequence analysis of R. favelukesii strains ORY1, LPU83, and Or191 showed that they have around 87.5% of common coding genes, including the symbiotic genes. Moreover, the phylogenetic analysis of ORY1 symbiotic genes nifH, nifD, nifK, nodA, nodB, and nodD were related to the symbiotic genes of E. meliloti. We teste ORY1 competitiveness by inoculating seeds with 99:1 and 1:99 ratios of ORY1::gusA/E. meliloti U143. In both treatments, ORY1::gusA occupied more than 50% of nodules, evidencing its high competitiveness. However, the aerial biomass in these treatments was remarkably different, suggesting that the nodules induced by the efficient strain are essential to provide enough N for optimal plant growth. These findings support the needing of inoculating in areas where inefficient strains are likely to be present. Finally, we found three genes that encode amino acid sequences for domains of M16 peptidases (with homology to bacterial hrrP and sapA genes), two of them were contiguous and located in an accessory plasmid, whereas the other one was a chromosomal gene. These genes are likely to be involved in the parasitic behaviour of ORY1 strain.
Introduction
Failures in alfalfa (Medicago sativa) production have been attributed to soil acid pH, aluminium toxicity (Bouton, 2012; Jaiswal et al., 2018; Shi et al., 2022) and the presence of parasite rhizobia strains that inefficiently nodulate alfalfa (Torres Tejerizo et al., 2011; Eardly et al., 2022). These parasite strains, known as Oregon-like, have tolerance to acidic conditions, a host broad range (Eardly et al., 1992; Del Papa et al., 1999), and its presence has also been confirmed in USA and Canada (Bromfield et al., 2010). Likewise, Oregon strain LPU83 has been isolated from alfalfa root nodules in Argentina and was assigned to the novel species Rhizobium favelukesii (Torres Tejerizo et al., 2016). Oregon strains are competitive for the nodulation of alfalfa in acid soils and have low symbiotic efficiency in this legume (Del Papa et al., 2003; Torres Tejerizo et al., 2011; Eardly et al., 2022). For this reason, it is important to know the presence of this type of strain in places where alfalfa is grown, as well as their competitiveness respect to the inoculant used or native efficient strains present in the soil.
Furthermore, the underling mechanism explaining the lack of nitrogen fixation in the presence of either parasite or inefficient strains remains largely unexplored. However, in the Ensifer meliloti -Medicago truncatula symbiosis, where E. meliloti inefficient strains were identified, the mechanism by which inefficiency was achieved was explained by the presence of rhizobium peptidases that negatively affect the symbiotic communication (Price et al., 2015). In particular, Crook et al. (2012) demonstrated that the inefficient E. meliloti strains were able to gain compatibility when they lose the Host Range (HR) accessory plasmid. In turn, efficient strains became inefficient when they acquired the HR plasmid. Around 10% of Ensifer sp. isolates were estimated to carry on this accessory plasmid a gene, named host range restriction peptidase (hrrP), coding for a M16 metallopeptidase that hydrolyses plant produced nodule rich cysteine (NCR) peptides. In M. truncatula, around 600 NCR peptides were found (Zhou et al., 2013). One of these peptides, NCR169, has been proven to be necessary for bacteroids differentiation in the nodule. M. truncatula KO-mutants for the NCR169 peptide, dnf7, are unable to induce bacteroid development, suggesting that the NCR169 peptide plays a role in the symbiotic communication (Horváth et al., 2015). Likewise, sapA (symbiotic-associated peptidase), a chromosomic gene of E. meliloti was shown to encode a HrrP-like M16 peptidase that modulates symbiosis in a similar way (Benedict et al., 2021). Here, we reported the presence of these genes in Oregon-type strains, which could contribute to the understanding of their poor symbiotic efficiency.
In Uruguayan soils, a competitive and ineffective rhizobium strain that nodulates alfalfa was identified and suggested to be an Oregon-like strain, yet not confirmed (Castro-Sowinsky et al., 2002). These parasite strains constitute a potential risk for alfalfa implantations (Nilsson et al., 2019), even when they are coexisting with the efficient symbiote E. meliloti. Therefore, in this study, we characterized alfalfa inefficient rhizobia strains isolated from plants grown in acid soils, aiming to confirm the presence of Oregon-like strains in Uruguay; evaluated the competitiveness of these strains; and the presence of M16-like peptidases to identify putative mechanisms that could explain the parasite behaviour.
Materials and methods
Bacteria and growth conditions
Acid-tolerant (pH 5.2) rhizobia strains isolated from nodules of Medicago sativa grown in acid soils in Uruguay (Table 1), were obtained from the rhizobia collection of the laboratory of Biochemistry at the School of Agronomy, Universidad de la República, Uruguay. Or191 from Oregon, USA (Eardly and David, 1985) and LPU83 from Argentina (Torres Tejerizo et al., 2016) were used as reference strains of Rhizobium favelukesii. Ensifer meliloti U143 (=MCH3) strain, the inoculant for alfalfa in Uruguay (Altier et al., 2013) was supplied by Microbiology Laboratory (Ministerio de Ganadería Agricultura y Pesca). Rhizobia and E. meliloti were grown at 28°C in YEM (Vincent, 1970). Escherichia coli strain S17-1 ʎpir containing the plasmid pCAM131 with the transposon mTn5SSgusA31 (Wilson et al., 1995) grown at 37°C in LB (Miller, 1972) supplemented with Spectinomycin (Sp) 100 ug/mL and Streptomycin (St) 50 µg/mL. In liquid media the cultures were shaken at 120 rpm. Rhizobia and E. coli strains are stored at 4°C in YEM agar or LB agar Sp 100 µg/mL, respectively.
Plants and growth conditions
Medicago sativa cv. Chaná seeds were superficially sterilized according to (Irisarri et al., 2019). Plants grew in control condition at 23/20°C (day/night), with a 16/8 h photoperiod and 220 µE.m-2.s-1 photosynthetic photon flux density.
Time nodulation assay was performed in tubes with 20 mL Jensen medium (Jensen, 1942) N-free. Competitiveness, symbiotic efficiency and relative symbiotic effectiveness assays were performed in 350 mL pots with sterile vermiculite - sand (1:1), watered alternatively with distilled water and with Fahraeus medium (Vincent, 1970). Distilled water pH and Fahraeus medium were adjusted to 5.5 with MES 10 mM. Inoculation was carried out as described below in each assay.
Tagging rhizobia ORY1 strain with gusA gene
The conjugation was carried out using the rhizobium strain ORY1 as receptor and E. coli strain S17-1 λ-pir containing pCAM131 that carries transposon mTn5SSgusA31 as donor, generally following the procedure used by Irisarri et al. (2019). The mixture remained at 28°C overnight in plate with TY (Beringer, 1974). Transconjugants were selected in plate with YEM Sp 100 µg/mL and Nitrofurantoin (Ntf) 20 µg/mL to inhibit E. coli growth.
Transconjugants time nodulation assay
Time nodulation assay of three transconjugants respect to the parental strain was evaluated according to time of appearance of the first nodule and total nodules at 21 days. The pre-germinated seeds, sown at a rate of one per tube with Jensen medium and 8 repetitions per treatment, were inoculated with 100 µL of a bacterial suspension with OD620 = 0.9 (108 ufc/mL). The ORY1::gusA clone was selected for the competition assay.
GUS activity in alfalfa nodules
Nodulated roots were washed with distilled water and with 0.1 M phosphate buffer pH=7, incubated 16 h at 37°C in darkness in solution type containing 1% SDS, EDTA 0.5 M pH 8 and 1 mM 5-bromo-4-cloro-3indolil-β-D-glucuronide (X-gluc) in 50 mM sodium phosphate buffer pH 7.5 (Wilson et al., 1995). Nodules occupied by ORY1::gusA were visually identified by blue staining, in contrast to those infected by non-tagged strains which remained unstained (Irisarri et al., 2019).
Competitiveness and biomass production assay
Competitiveness of U143 and ORY1::gusA strains was carried out in pots with sand-vermiculite (1:1), with 5 plants per pot, and 4 replicates per treatment. Three assays with a n = 4 were conducted and the 12 resulting replicates were pooled for the statistical analysis. Pots were rinsed with water or Fahraeus medium at pH 5.5, as indicated above. Strains grew in YEM medium up to an OD620 = 0.9. Competitiveness experiments included ORY1::gusA and U143 in a 99:1 and 1:99 ratio (named treatment 99% and 1% respectively). Bacterial concentration was determined according to Riviezzi et al. (2020). Plants were harvested 21 days after inoculation and washed root nodules were stained as described above. To assess symbiotic efficiency, biomass production was determined as shoot dry weight at 60°C for 72 h, including two controls: U143 strain and uninoculated treatment.
Relative symbiotic effectiveness assay
Seeds were sown at the rate of 5 per pots and inoculated with ORY1 and U143 strains as described previously, and harvested 35 days after inoculation. Each treatment included 5 replicates. Relative symbiotic effectiveness (%) is based on ORY1 parasitic strain shoot dry weight production relative to effective U143 strain.
Genomic DNA extraction
Genomic DNA extraction from different rhizobial strains were extracted with DNA Extraction kit (Qiagen, Alemania). DNA quality and concentration were determined with Nanodrop 2000 (Thermo Scientific, USA), and its integrity was visualized in agarose gel 1.2%.
16sRNA gene amplification
16S rRNA partial gene was amplified using the primers 27f and 1525r and conditions described by Lane (1991). PCR products were run at 100 V in Tris-acetate buffer pH 8.2 and visualized in 1.2% agarose gel stained with SYBR Safe DNA Gel Stain (Thermo Fisher Scientific, USA). Accuruler 1 kb DNA Ladder (Maestrogen Inc, Taiwan) was used as molecular weight marker. Sequencing was performed in Macrogen Inc. (Korea).
BOX genomic fingerprinting
Total genomic DNA was amplified with BOXA1 primer (Koeuth et al., 1995). Amplification condition used was 1 cycle of 2 min at 95°C, 30 cycles of 45 seg at 93°C, 1 min at 50°C and 6 min at 65°C, and a final extension of 8 min at 68°C. PCR products were visualized as described previously.
Genome sequencing
Genomic sequencing was obtained using Illumina Technology (Macrogen, Korea) yielding 11.1 million paired-end reads with a length of 151 bp. Low quality reads were filtered out using Trimmomatic software with parameters 2:30:10 LEADING:3TRAILING:3 SLIDINGWINDOW:4:15 MINLEN:75 (Bolger et al., 2014). SPAdes software was used to perform a de novo assembly using the remaining paired reads (Bankevich et al., 2012). The quality of the assembly was assessed with QUAST software using LPU83 genome as reference (Gurevich et al., 2013). Plasmid contigs were detected using plaSquid software (Giménez et al., 2022) with the option –minidist and using LPU83 plasmids as reference database. Genomic annotation of all the analysed genomes was done using prokka software with default parameters (Seemann, 2014).
The calculation of the Average Nucleotide Index (ANI), correlation indexes of tetra-nucleotide signatures, and tetra correlation search (TCS) were performed using the JSpeciesWSserver (Richter et al., 2016). The genome sequence data were uploaded to the Type Genome Server (TYGS), for a whole genome-based taxonomic analysis (Meier-Kolthoff and Göker, 2019).
Phylogenetic analysis
Symbiotic gene nifH, nifD, nifK, nodA, nodB and nodD, sequences were extracted from the ORY1 genome. The obtained sequences were compared with reference strains sequences available in public National Center for Biotechnology Information (NCBI) database. Sequences were aligned with the ClustalW algorithm and Maximum likelihood phylogenomic tree (Tamura and Nei, 1993) were constructed for each multilocus sequence analysis (MLSA). Alignments and trees were constructed with MEGA 11 software (Tamura et al., 2021). Statistical support for tree nodes was evaluated by bootstrap analyses using 1000 replicates (Felsenstein, 1985).
Nucleotide sequence accession numbers
The GenBank accession numbers for 16S rRNA sequences used in phylogenetic analysis are: Rhizobium favelukesii ORY1 (OP294988), ORY2 (OP294989), ORY3 (OP294990), ORY4 (OP294991), ORY5 (OP294992), Or191 (EU928874.1), Rhizobium anhuiense PVPR1(MT476932.1), Rhizobium indigoferae NAC94(MK872365.1), Bradyrhizobium yuanmingense VAFW14 (LC585439.1), Rhizobium croatiense 9T (MK753104.1), Rhizobium altiplani BR 10423 (NR 152084.1), Rhizobium tibeticum CCBAU85039 (NR116254.1) and Sinorhizobium meliloti 2011 (CP004140.1).
The GenBank accession numbers for genomes and plasmids from which the symbiotic genes and some partial 16S sequences were extracted are indicated in brackets: Rhizobium favelukesii ORY1 (JAIRAY000000000.1); LPU83 (HG916852.1) and Or191 (GCA_000419725.1); Rhizobium tibeticum CCBAU85039 (GCF_900108425.1); Rhizobium altiplani BR 10423 (GCA001542405.1); Rhizobium grahamii BG7 (CP043498.1); and Sinorhizobium meliloti 2011 (CP004140.1); Rhizobium tropici CIAT 899 (NC 020061.1); Rhizobium esperanzae N561 (CP013501.1); Rhizobium phaseoli R650 (CP013533.1); Rhizobium gallicum sp. R602 (CP006878.1); Rhizobium etli NXC12 (CP020907.1).
Rhizobium plasmidic peptidase analysis
Ensifer meliloti USDA1963 peptidase HrrP was retrieved from NCBI reference proteins database and used to detect its domains in Pfam web server (Bateman et al., 2004). Hidden Markov models from M16 Peptidase (PF00675) and M16_C Peptidase (PF05193) were downloaded from Pfam database. To understand the presence of potential peptidases in plasmids obtained from rhizobium genus, 461 plasmids were downloaded from PLSDB database (V. 2021_06_23_v2) (Galata et al., 2019). All encoded proteins were predicted using prokka software. HMMER 3.0 software was used to scan for M16 peptidase domains using the option –cut_ga as filtering threshold (Eddy, 2011). All predicted proteins were clustered to 98% of identity, using CD-HIT software, to reduce sequence redundancy (Fu et al., 2012). R. favelukesii predicted M16 peptidases were added to the phylogenetic analysis. Sequences (available at: https://doi.org/10.6084/m9.figshare.21641816.v1) were aligned using muscle algorithm through msa R package (Bodenhofer et al., 2015). Phylogenetic trees were computed using neighbor-joining algorithm with ape R package (Paradis et al., 2004) and drawn using ggtree (Yu et al., 2017). Domain organization of each sequence was manually obtained from Pfam database search and added to the analysis.
Statistical analysis
ANOVA was performed in R software to identify statistical significance and a post hoc test (Tukey´s test) was used for pairwise comparison to determine the P-values.
Results
ORY1 alfalfa-parasite rhizobia strain is a Rhizobium favelukesii
To characterize the M. sativa-parasite rhizobia ORY1, ORY2, ORY3, ORY4, and ORY5 strains, isolated from Uruguayan acidic soils, we analysed BOX profile, the 16S rRNA sequences, and Relative symbiotic effectiveness.
BOX profiles for the five autochthonous isolates and the Argentinian and USA R. favelukesii strains showed that ORY1 and ORY5 strains had the same profile as the foreign strains, whereas ORY2, ORY3, and ORY4 strains had the same profile, but different to the foreign strains (Figure 1A).
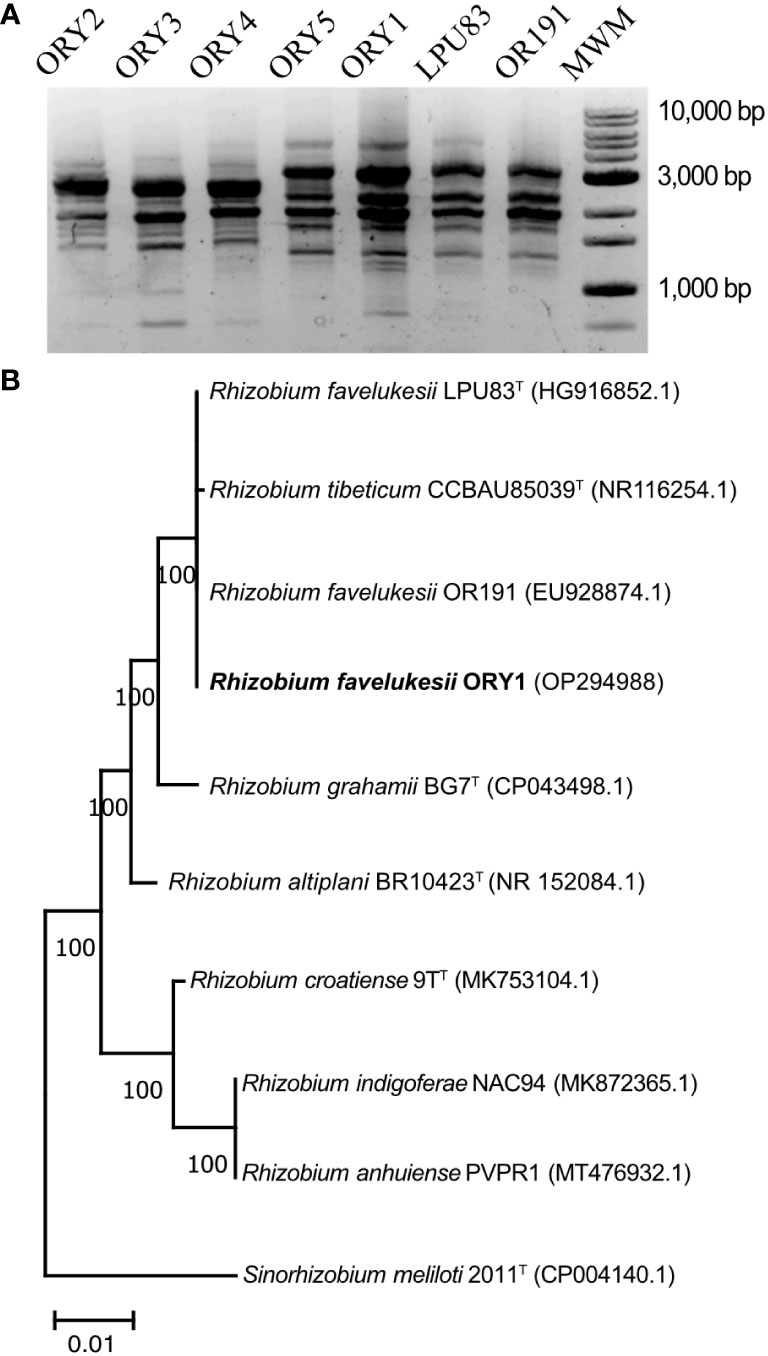
Figure 1 Diversity and phylogenetic relations of R. favelukesii strains. (A) BOX profiles of R. favelukesii strains. ORY1, ORY2, ORY3, ORY4 and ORY5 strains were collected in Uruguayan acids soils and the reference strains LUP83 and Or191 from acid Argentinan and USA soils respectively. MWM (Molecular weight marker). (B) Phylogenetic tree inferred using Maximum likelihood method based on partial sequences alignments of 16S rRNA genes (1.342nt). With bold letters is indicated the strain isolated from Uruguayan soil. The genome of the other strains was obtained from the NCBI. Asterisk (*) indicates the Type strain. Bootstrap values calculated for 1000 replications are indicated. Bar, 1 nt substitution per 100 nt.
The partial 16S rRNA sequence gene of ORY1, ORY4 and ORY5 were 100% identical to the R. favelukesii strains, LPU83 and Or191, isolated from Argentinian and USA acid soils respectively. ORY2 and ORY3 strains showed respectively a 99.9% and 99.7% identity to ORY1, LPU83, and Or191. Next, we performed a phylogenetic analysis of the different strains isolated from Uruguayan soils (ORY) among other related species. All ORY strains clustered together with R. favelukesii LPU83 and Or191 strains, and closely related to R. tibeticum (Figure 1B). Hereafter, ORY1 strain was used as the strain of study for all further assays. The parasitic behaviour of ORY1 was confirmed by shoot dry weight production in presence of this strain as the sole inoculant. This parameter was only 15% of such obtained with the commercial inoculant strain U143, evidencing a very low symbiotic efficiency.
Genome sequencing and phylogenetic analysis of the ORY1 strain
The analysis of the draft genome of R. favelukesii ORY1 strain revealed that this genome is 7.44 Mb in size; has 59.7% GC content; containing 7,509 coding and 51 RNA genes. Five replicons were identified corresponding to the chromosome and four plasmids. The analysis of plasmids revealed that 151 contigs covered 96% of the total length of Rhizobium favelukesii LPU83 plasmids. This represents a total length of 3.25 Mb which encode 3,349 ORFs. Based on TCS or tetra-nucleotide signature, only R. favelukesii and R. tibeticum were found closely related with z-scores > 0.999. ANI values of > 96% consistently group genomes from strains of R. tibeticum and R. favelukesii together with ORY1, but ANI values of > 99% identify ORY1 as R. favelukesii. According to TYGS analysis ORY1 belongs to R. favelukesii. The phylogenetic analysis of R. favelukesii ORY1, Or191, and LPU83 strains showed that they clustered together, separated from R. tibeticum, when using the complete genomes (Figure 2A). In addition, R. favelukesii and R. tibeticum also grouped separated from R. grahamii and R. altiplani (Figure 2A). Likewise, R. favelukesii strains does not group with R. tibeticum when a MLSA of symbiotic genes, nifD, nifK, nifH, nodA, nodB, and nodD, was considered. In addition, these concatenated genes are related to E. meliloti symbiotic genes (Figure 2B). To better understand the similitude between the three R. favelukesii strains, ORY1, Or191, and LPU83, we analyse the overlap between the three complete genomes by identifying the homologous genes. We observed that most of the coding genes are common to all strains (around 87.5%, relative to ORY1), Or191 had a greater overlap with ORY1 than LPU83, and around 2.7% coding genes were unique for ORY1 (Figure 2C).
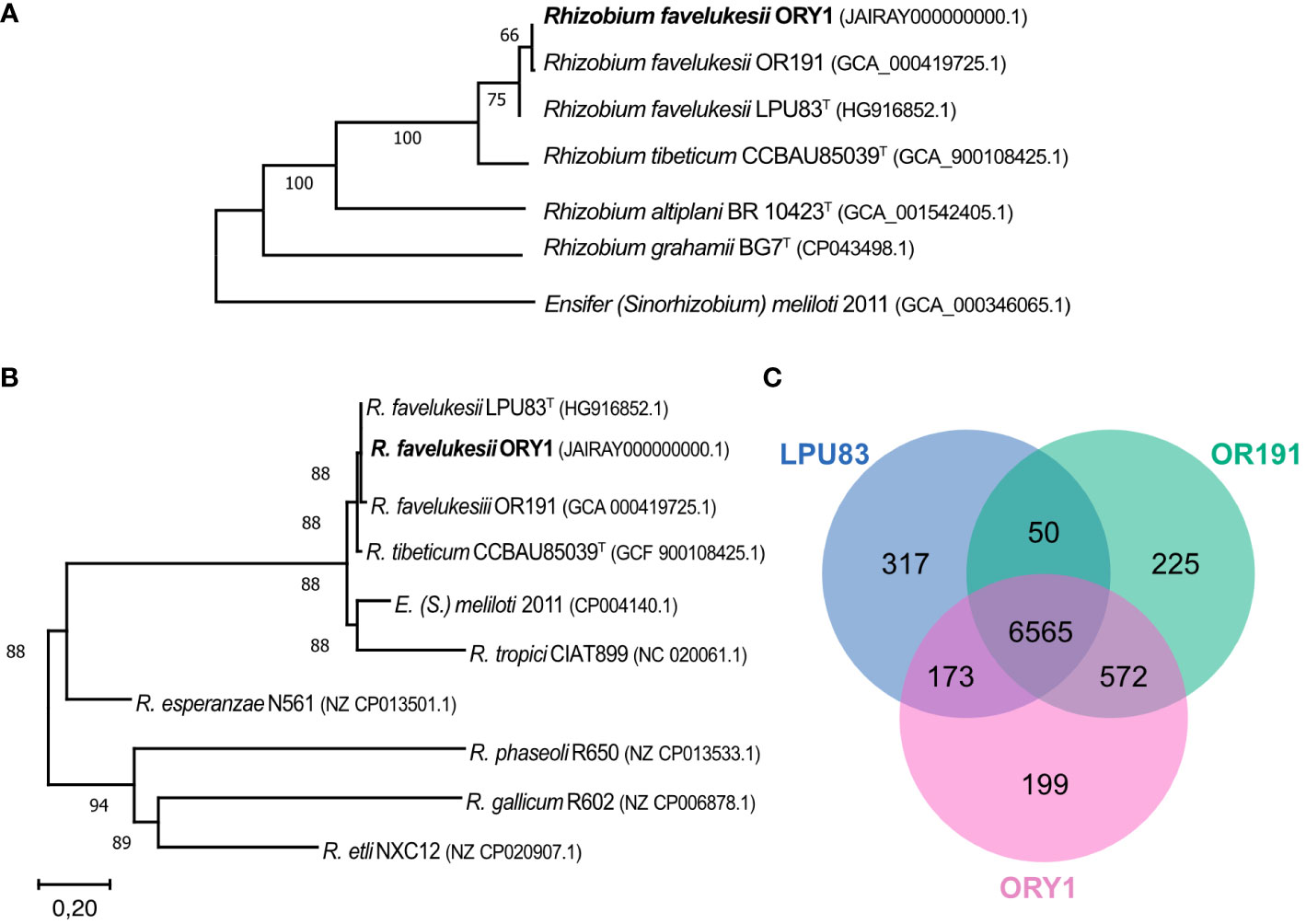
Figure 2 Phylogenetic analysis and Venn diagram of homologous genes in three R. favelukesii strains. (A) Tree inferred from Genome Blast Distance Phylogeny (GBDP) distances calculated from genome sequences with TYGS (https://tygs.dsmz.de/). The branch lengths are scaled in terms of GBDP distance. The numbers above branches are GBDP pseudo-bootstrap support values > 60% from 100 replications, with an average branch support of 83.8%. The tree was rooted at the midpoint. (B) MLSA of symbiotic genes nifD, nifK, nifH, nodA, nodB, and nodD of different rhizobia species. (C) Venn diagram of homologous genes in three R. favelukesii strains. Overlapped regions represent common homologous genes shared between pan-genomes.
Competitiveness and symbiotic efficiency of ORY1 in presence of the efficient U143 strain
To evaluate the competitiveness of ORY1 strain we tagged ORY1 with the reporter gene, gusA. To confirm that the gusA insertion did not affect competitiveness and shoot biomass production, we compared the performance of ORY1::gusA strain for these parameters, to the parental strain ORY1. No differences were observed between these strains in terms of time to observe the first nodule (8 days), number of nodules per plant at 21 days, and shoot biomass.
Two treatments were used to test competitiveness and biomass production, consisting of mixes of ORY1 and the commercial inoculant, E. meliloti U143. One treatment consisted of a 99:1 ratio (ORY1::gusA/U143, hereafter 99% parasite strain treatment), whereas the other treatment was a 1:99 ratio (ORY1::gusA/U143, hereafter 1% parasite strain treatment). Nodule occupation by ORY1::gusA strain in 99% treatment was greater than in 1% treatment (Figure 3A). It is worth noting that even in 1% treatment, the percentage of occupied nodule exceeded the 50% of total nodules, evidencing the great competitiveness of this strain. Despite of the fairly similar occupation of both treatments, a remarkable difference was observed in terms of biomass production between 99% and 1% treatments (Figure 3B). In fact, the biomass production in 99% treatment was very low and not statistically different to the one obtained in the non-inoculated control, whereas the biomass production in the 1% treatment was not statistically different to the one obtained with U143 strain (Figure 3B). Regardless the percentage of parasite occupied nodules, the total number of nodules was much greater in the 99% treatment (Figure 3C). Despite of the greater number of nodules in the 99% treatment, the number of white nodules was significantly greater in the 1% treatment (Figure 3D).
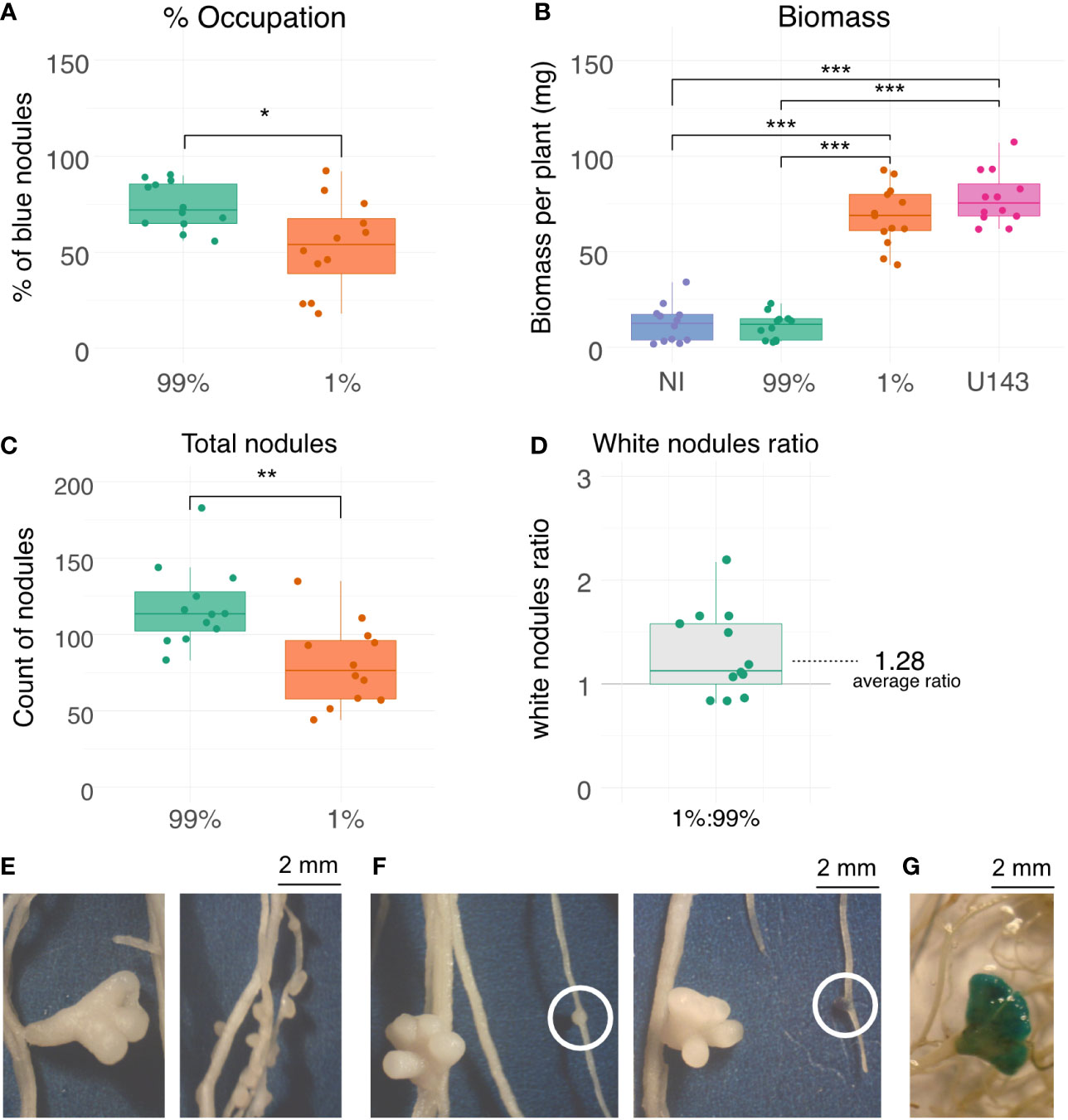
Figure 3 Competitiveness, biomass production and nodule morphology in plants inoculated with parasitic and efficient strains. (A) Nodule occupation percentage by tagged strain ORY1::gusA respect to total nodules. (B) Aerial biomass production. (C) Number of total nodules. (D) White to total nodule ratio. (E) Nodule aspect of those occupied by inefficient and efficient strains (left and right, respectively). (F) GUS staining of nodules (left prior staining, and right after staining). (G) GUS staining of a nodule presenting a different morphology (U143 induced morphology-like). 99% and 1% correspond to co-inoculations with a ratio 99:1 and 1:99 of ORY1::gusA and U143, respectively; NI, non-inoculated control; U143, inoculated with the efficient strain used as commercial inoculant. *, p value < 0.05; **, p value < 0.01; ***, p value < 0.001.
Nodule morphology induced by ORY1::gusA and U143 strains was different (Figure 3E). We observed that ORY1::gusA induces the development of a great number small spherical nodules unlike U143, which induces a small number of larger undetermined nodules (Figure 3F). Exceptionally, a few U143-occupied-like nodules were occupied by ORY1::gusA (Figure 3G), a phenomena that we attribute to co-occupation of these strains, given that we never observed this morphology when plants are solely inoculated with ORY1::gusA.
Identification of M16 peptidase domains in ORY1 strain
Given that the bacterium genes hrrP and sapA encode for M16 peptidases that have been related to rhizobia with low symbiotic efficiency, we sought for genes coding M16 peptidase in the ORY1 genome. Bioinformatic analysis of protein domains using HMMER software, together with Pfam proteins database allowed us to evidence in ORY1 genome the presence of three genes encoding aminoacidic sequences for M16 zinc metallopeptidase domains. Two of these genes are contiguous gene located on an accessory plasmid, and the other is a chromosomic gene.
We performed a NCBI protein BLAST search and found that the 448 (ID 07295) and 532 (ID 07296) aminoacidic contiguous sequences encoded on the accessory plasmid of ORY1 strain showed an identity of 22.78 and 26.55%, and a coverage of 50 and 63%, respectively, to the HrrP peptidase of E. meliloti USDA1963 (Table 2). This protein is an homodimeric M16 zinc metallopeptidase involved in the cleavage of NCR peptides. In the chromosome of ORY1 strain, we identified a DNA sequence coding a 432 aminoacid sequence presenting an 82.78% of identity and 98% of coverage to SapA peptidase from E. meliloti 1021 strain (CAC45492.2). This E. meliloti SapA is a member of a conserved M16B subfamily peptidase present in many different rhizobia.

Table 2 Blast analysis of M16 domains identified in ORY1 against reference sequences. E-value corresponds the number of expected hits of similar quality (score) that could be found just by chance.
To assess the presence of M16 genes in rhizobia, we analyzed 461plasmids available at PLSDB database. Only 30 genes encoding this type of protein domains were found, and a few of them corresponded to the same replicons. M16 domain-encoding genes were found in rhizobia plasmids of different species such as R. leguminosarum, R. etli, R. favleukesii and R. gallicum (Figure 4). Additionally, clusters of sequences found in this phylogenetic tree do not show a species-based distribution.
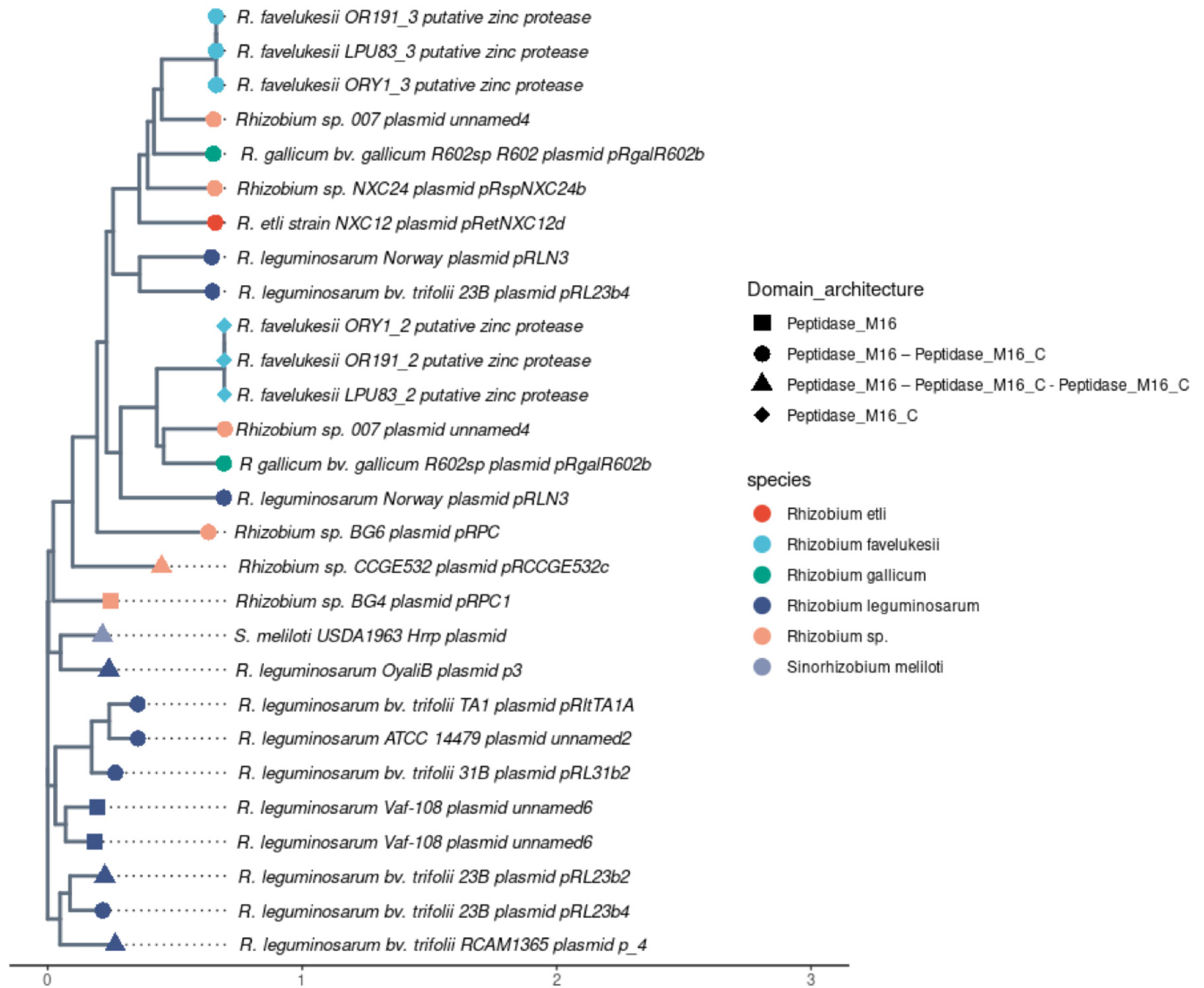
Figure 4 Phylogenetic tree of M16 peptidase sequences encoded in plasmids of different rhizobia species. The phylogenetic tree of peptidase sequences was computed with Neighbourg-Joining algorithm. Tip colours indicate the rhizobium host species. Tip shapes indicate different domain architectures, which were manually browsed in the Pfam web server for each peptidase sequence.
To understand remote homology patterns in these genes we further analyzed domain architecture of the encoded peptidases in rhizobium plasmids (Figure 4). We observed that there are different domain organizations but with a limited number of protein domains; specificallyM16 (PF00675) and M16_C (PF05193). Peptidase M16 followed by Peptidase M16_C is the domain architecture most present in these proteins, at least one gene for the 5 rhizobia species represented in the tree have this domain organization. A reference of an uncharacterized protein having this domain is deposited in UniProt under accession number W2S8M8 with its predicted AlphaFold structure. As a functionally characterized protein we included HrrP of E.meliloti USDA 1963, which resulted in a similar domain architecture only having an extra Peptidase M16_C domain. A reference protein having these domains is also deposited in UniProt under accession W9RIX3.
Discussion
Phylogeny and diversity of Oregon strains isolated in Uruguayan acids soils
Alfalfa parasitic Oregon-like rhizobia are a problem for the implantation and production grasslands of this legume (Torres Tejerizo et al., 2011). These rhizobia had been identified in USA, Canada (Eardly et al., 1985; Bromfield et al., 2010) and in Argentina, where the novel species R. favelukesii was described (Torres Tejerizo et al., 2016). Castro-Sowinsky et al. (2002) purposed the presence of Oregon-like rhizobia in Uruguayan soils. However, the presence of this rhizobium in Uruguayan soils had not been confirmed before. In this study, based on the whole genome sequencing analysis of five isolates obtained from Uruguayan acid soils, where there are yield problems in alfalfa production, we confirmed the presence of Oregon strains (Figure 2A).
R. favelukesii and R. tibeticum can nodulate M. sativa (Hou et al., 2009), but R. tibeticum has multiple hosts such as Trigonella archiducis-nicolai, Medicago lupulina, Phaseolous vulgaris, among others (Abd-Alla et al., 2014; Torres Tejerizo et al., 2017). In congruence, our genome based phylogenetic analysis showed that R. favelukesii strains (ORY1, Or191, and LPU83) clustered together among them but separated from R. tibeticum strain CCBAU85039 (Figure 2A). Moreover, the genome comparison based on common genes revealed that ORY1 strain is more similar to Or191 isolated from USA than LPU83 isolated from Argentina (Figure 2C). The limited number of unique genes in ORY1 (2.7%) are mostly uncharacterized genes and we did not identify any symbiotic genes.
Our results also showed a greater diversity than that observed in Argentinian acid soils. In our study, among the five strains isolated from Uruguayan acid soils, two strains presented the same BOX profile as LPU83 and Or191, identified in Argentina and USA respectively, while the other three presented a profile different to those. Unlike this result, twelve isolated from Argentinian acid soils resulted in the very same ERIC fingerprint profile as Or191 from USA (Wegener et al., 2001), representing a very low genetic diversity. The low genetic diversity is different to what is often reported for rhizobia having a wide geographic distribution (Martínez-Romero and Caballero-Mellado, 1996), and to what we observed for clover-nodulating rhizobia (Irisarri et al., Tartaglia et al., 2019) and lotus-nodulating rhizobia (Sotelo et al., 2011; Batista et al., 2013) isolated from Uruguayan soils. Nevertheless, the twelve isolates tested by Wegener et al. (2001) were selected from an initial collection of 465 isolated from Argentinian soils, with pH between 5.5 and 6.2, by growing them at pH 5.0 (Del Papa et al., 1999). This means that the twelve isolated with identical ERIC fingerprint were selected by high tolerance to low pH, and extreme environmental conditions are known to reduce genetic diversity, whereas the pH of Uruguayan soils where our strains were isolated were only slightly acid (pH 5.4 - 5.8), explaining the greater genetic diversity observed in our collection.
Alfalfa co-inoculation with ORY1/U143: Competitiveness and biomass production
The high competitiveness of the parasitic ORY1 strain was evidenced by the occupancy of nodules in the 1% treatment (Figure 3A). Interestingly, the biomass production was high, and not different to that for U143 (Figure 3B), even though more than 50% of nodules were occupied by the parasite strain ORY1.This result suggests that the presence of parasite rhizobia should not be a problem for biomass production if our design (1:99, parasite:efficient rhizobia) represents the situation occurring during the first few days after sowing, where the strain used as commercial inoculants are expected to be at greater concentration than native parasite rhizobia. On the other hand, our results show that if the load of commercial inoculant is very low, or if the load of parasite rhizobia in the soil is high, severe problems in biomass production can be found (Figure 3B).
At first glance, it sounds counterintuitive the fact that we observe a high biomass production when the occupation by the parasite strain, ORY1, is greater than 50%. This can be in part explained by the fact that the 1% treatment had approximately 30% more white (effective) nodules relative to the 99% treatment (Figure 3D). It is worth noting that the incompatible plasmid-carrying strain can induce a higher number of nodules than the effective strains, even though the resulting nodules are less beneficial to the host (Crook et al., 2012). This goes in line with our observations, where we found a greater number of total nodules in the treatment enriched in ORY1 relative to the 1% treatment (Figure 3C). However, the nodules produced by the parasite strain were shown to be less beneficial for the plant compared to those produced by effective strains, which were suggested to produce bigger and more dense nodules (Crook et al., 2012). Therefore, if we only consider the white nodule as nitrogen fixing active nodules, it is not surprising that the 1% treatment had a greater biomass production than the 99% treatment. Another factor that could account for the high biomass production even when the occupation of ORY1 is relatively high, is the possibility of co-occupation with efficient strains. Co-occupation has been reported in M. sativa – E. meliloti symbiosis (Checcucci et al., 2016). Hence, we hypothesize that many of the blue nodules can be also occupied with the effective strain which contributes to nitrogen fixation. In fact, we observed a few well-developed nodules that were marked with the parasite strain (Figure 3G), strongly suggesting the possibility of co-occupation in those nodules. Thus, our observations remark the importance of not only evaluating competitiveness as percentage of occupied nodules, as usually tested (Irisarri et al., 2019), but also in terms of total, well-developed, not developed, and co-occupied nodules, to better understand the consequence at plant level.
Identification of putative hrrP and sapA genes in ORY1 strain
One of the plasmid detected in ORY1 genome encoded contagious putative M16 peptidase, which are highly conserved in the three available genomes of R. favelukesii. The phylogenetic analysis of putative M16 peptidases in a comprehensive set of Rhizobium plasmids showed that the R. favelukesii putatives peptidases do not cluster by species in the phylogenetic tree, similarly to what occurs for Ensifer sequences (Figure 4). This has been proposed as a result of lateral transfer events shaping the evolutionary pathways of these plasmid peptidases (Price et al., 2015).
M16 plasmid peptidases have been previously studied in E. meliloti and could be linked to host restriction functions (Price et al., 2015). The two M16 peptidases encoded in a R. favelukesii plasmid are contiguous and both of them together mimic the domain architecture of E. meliloti HrrP protein, where one sequence encodes for M16 - M16C domains, and the next sequence for another M16C domain. Given the proximity of M16 peptidase-encoding genes we propose that they could be related to hrrP as part of a gene fusion or fission event, as it has been seen in a set of Gram + and Gram – bacterias (Pasek et al., 2006). Additionally, these proteins could be implied in a similar host restriction mechanism as the one previously described in E. meliloti species. In particular, HrrP and SapA peptidases play a role in the digestion of NCR peptides produced by the host, for instance, the indispensable peptide for bacteroid differentiation NCR169 (Horváth et al., 2015; Price et al., 2015; Benedict et al., 2021). These peptides are necessary for the correct establishment of the symbiosis E. meliloti - M. truncatula (Price et al., 2015; Benedict et al., 2021). The plasmidic peptidase HrrP degrades NCR peptides, attributing the rhizobia an active mechanism to control de host (Pan and Wang, 2017; Benedict et al., 2021), and its expression is often associated with a parasite behaviour (Price et al., 2015). The presence of putative hrrP and sapA genes in R. favelukesii Oregon strains, ORY1, Or191, and LPU83 can partially explain the parasitic behaviour of R. favelukesii evidenced by the unusual globular aspect of nodules, hyper-nodulation, and low symbiotic efficiency.
Conclusions
In Uruguay, alfalfa is mainly sowed in acid soils, in which we confirmed the presence of Oregon type parasitic strains, whose BOX profiles allow us to suppose that the diversity of this strains is higher than reported before. Co-inoculation assays with a low parasite/efficient strains ratio evidenced, on the one hand, ORY1 strain is significantly more competitive than the U143 strain, and on the other hand that in the first stages of seedling development the biomass loss is not significative. These findings support the need of inoculating in areas where inefficient strains are likely to be present. Finally, we described for first time the presence of hrrP and sapA genes in R. favelukesii, which can partially explain the parasitic behaviour of Oregon – like strains characterized by the unusual globular aspect of nodules, hyper-nodulation, and low symbiotic efficiency.
Data availability statement
The datasets presented in this study can be found in online repositories. The names of the repository/repositories and accession number(s) can be found below: https://www.ncbi.nlm.nih.gov/, JAIRAY000000000.1.
Author contributions
The authors confirm contribution to the paper as follows: Study conception and design: JM. Data collection: AB-R, MG and MM. Analysis and interpretation of results: SS, AB-R, MG and MM, JM. Draft manuscript preparation: AB-R, SS, JM. All authors reviewed the results and approved the final version of the manuscript. All authors contributed to the article and approved the submitted version.
Funding
Authors are grateful to the funding of grants from INIA L1 Proyecto 4. PEDECIBA, Udelar-CAP (Magister grant for A. Berais-Rubio) and SNI Uruguay.
Conflict of interest
The authors declare that the research was conducted in the absence of any commercial or financial relationships that could be construed as a potential conflict of interest.
Publisher’s note
All claims expressed in this article are solely those of the authors and do not necessarily represent those of their affiliated organizations, or those of the publisher, the editors and the reviewers. Any product that may be evaluated in this article, or claim that may be made by its manufacturer, is not guaranteed or endorsed by the publisher.
References
Abd-Alla M. H., Issa A. A., Ohyama T. (2014). “Impact of harsh environmental conditions on nodule formation and dinitrogen fixation of legumes,” in Advances in biology and ecology of nitrogen fixation, (London: IntechOpen) vol. 9. , 1.
Altier N., Beyhaut E., Pérez C. (2013). “Root nodule and rhizosphere bacteria for forage legume growth promotion and disease management,” in Bacteria in agrobiology: Crop productivity (Berlin, Heidelberg: Springer), 167–184.
Bankevich A., Nurk S., Antipov D., Gurevich A. A., Dvorkin M., Kulikov A. S., et al. (2012). SPAdes: A new genome assembly algorithm and its applications to single-cell sequencing. J. Comput. Biol. 19 (5), 455–477. doi: 10.1089/cmb.2012.0021
Bateman A., Coin L., Durbin R., Finn R. D., Hollich V., Griffiths-Jones S., et al. (2004). The pfam protein families database. Nucleic Acids Res. 32 (suppl_1), D138–D141. doi: 10.1093/nar/gkh121
Batista L., Tomasco I., Lorite M. J., Sanjuán J., Monza J. (2013). Diversity and phylogeny of rhizobial strains isolated from Lotus uliginosus grown in Uruguayan soils. Appl. Soil. Ecol. 66, 19–28. doi: 10.1016/j.apsoil.2013.01.009
Benedict A. B., Ghosh P., Scott S. M., Griffitts J. S. (2021). A conserved rhizobial peptidase that interacts with host-derived symbiotic peptides. Sci. Rep. 11 (1), 1–10. doi: 10.1038/s41598-021-91394-x
Beringer J. E. (1974). R factor transfer in Rhizobium leguminosarum. Gen. Microbiol. 84, 188–198. doi: 10.1099/00221287-84-1-188
Bodenhofer U., Bonatesta E., Horejš-Kainrath C., Hochreiter S. (2015). Msa: An r package for multiple sequence alignment. Bioinformatics 31 (24), 3997–3999. doi: 10.1093/bioinformatics/btv494
Bolger A. M., Lohse M., Usadel B. (2014). Trimmomatic: A flexible trimmer for illumina sequence data. Bioinformatics 30 (15), 2114–2120. doi: 10.1093/bioinformatics/btu170
Bouton J. H. (2012). An overview of the role of lucerne (Medicago sativa l.) in pastoral agriculture. Crop Pasture Sci. 63 (9), 734. doi: 10.1071/cp1212
Bromfield E. S. P., Tambong J. T., Cloutier S., Laguerre G., Prevost D., Laguerre G., et al. (2010). Ensifer, Phyllobacterium and Rhizobium species occupy nodules of Medicago sativa (alfalfa) and Melilotus alba (sweet clover) grown at a Canadian site without a history of cultivation. Microbiology. 156 (pt.2), 505–520. doi: 10.1099/mic.0.034058-0
Castro-Sowinsky S., Carrera I., Catalan A. I., Coll J., Martínez-Drets G. (2002). Occurrence, diversity and effectiveness of mid-acid tolerant alfalfa nodulating rhizobia in Uruguay. Symbiosis 32, 105–118.
Checcucci A., Azzarello E., Bazzicalupo M., Galardini M., Lagomarsino A., Mancuso S., et al. (2016). Mixed nodule infection in Sinorhizobium meliloti–Medicago sativa symbiosis suggest the presence of cheating behavior. Front. Plant Sci. 7, 835. doi: 10.3389/fpls.2016.00835
Crook M. B., Lindsay D. P., Biggs M. B., Bentley J. S., Price J. C., Clement S. C., et al. (2012). Rhizobial plasmids that cause impaired symbiotic nitrogen fixation and enhanced host invasion. Mol. Plant-Microbe Interact. 25 (8), 1026–1033. doi: 10.1094/MPMI-02-12-0052-R
Del Papa M. F., Balague L. J., Castro-Sowinski S. C., Wegener C., Segundo E., Abarca F. M., et al. (1999). Isolation and characterization of alfalfa-nodulating rhizobia present in acidic soils of central Argentina and Uruguay. Appl. Environ. Microbiol. 65 (4), 1420–1427. doi: 10.1128/AEM.65.4.1420-1427.1999
Del Papa M. F., Pistorio M., Balagué L. J., Draghi W. O., Wegener C., Perticari A., et al. (2003). A microcosm study on the influence of pH and the host-plant on the soil persistence of two alfalfa-nodulating rhizobia with different saprophytic and symbiotic characteristics. Biol. fertility soils 39 (2), 112–116. doi: 10.1007/s00374-003-0690-6
Eardly B. D., David B. (1985). Characterization of rhizobia from ineffective alfalfa nodules: Ability to nodulate bean plants [Phaseolus vulgaris (L.) savi.] T. Appl. Environ. Microbiol. 50 (6), 1422–1427. doi: 10.1128/aem.50.6.1422-1427.1985
Eardly B. D., Osman W. A. M., Ardley J., Zandberg J., Gollagher M., van Berkum P., et al. (2022). The genome of the acid soil-adapted strain rhizobium favelukesii OR191 encodes determinants for effective symbiotic interaction with both an inverted repeat lacking clade and a phaseoloid legume host. Front. Microbiol. 13. doi: 10.3389/fmicb.2022.735911
Eardly B. D., Young J. P. W., Selander R. K. (1992). Phylogenetic position of rhizobium sp. strain Or191 a symbiont of both Medicago sativa and Phaseolus vulgaris, based on partial sequences of the 16S rRNA and nifH genes. Appl. Enviroment. Microbiol. 58 (6), 1809–1815. doi: 10.1128/aem.58.6.1809-1815.1992
Eddy S. R. (2011). Accelerated profile HMM searches. PloS Comput. Biol. 7 (10), e1002195. doi: 10.1371/journal.pcbi.1002195
Felsenstein J. (1985). Confidence limits on phylogenies: An approach using the bootstrap. evolution 39 (4), 783–791. doi: 10.1111/j.1558-5646.1985.tb00420.x
Fu L., Niu B., Zhu Z., Wu S., Li W. (2012). CD-HIT: Accelerated for clustering the next-generation sequencing data. Bioinformatics 28, 3150–3152. doi: 10.1093/bioinformatics/bts565
Galata V., Fehlmann T., Backes C., Keller A. (2019). PLSDB: A resource of complete bacterial plasmids. Nucleic Acids Res. 47 (D1), D195–D202. doi: 10.1093/nar/gky1050
Giménez M., Ferrés I., Iraola G. (2022). Improved detection and classification of plasmids from circularized and fragmented assemblies. bioRxiv. doi: 10.1101/2022.08.04.502827
Gurevich A., Saveliev V., Vyahhi N., Tesler G. (2013). QUAST: Quality assessment tool for genome assemblies. Bioinformatics 29 (8), 1072–1075. doi: 10.1093/bioinformatics/btt086
Horváth B., Domonkosa Á., Keresztb A., Szucs A., Ábrahámb E., Ayaydinc F., et al. (2015). Loss of the nodule-specific cysteine rich peptide, NCR169 abolishes symbiotic nitrogen fixation in the medicago truncatula dnf7 mutant. Proc. Natl. Acad. Sci. U.S.A. 112, 15232–15237. doi: 10.1073/pnas.1500777112
Hou B. C., Wang E. T., Li Y. Jr., Jia R. Z., Chen W. F., Gao Y., et al. (2009). Rhizobium tibeticum sp. nov., a symbiotic bacterium isolated from Trigonella archiducis -nicolai (Širj.) vassilcz. Int. J. systematic evolutionary Microbiol. 59 (12), 3051–3057. doi: 10.1099/ijs.0.009647-0
Irisarri P., Cardozo G., Tartaglia C., Reyno R., Gutiérrez P., Lattanzi F., et al. (2019). Selection of competitive and efficient rhizobia strains for white clover. Front. Microbiol. 10. doi: 10.3389/fmicb.2019.00768
Jaiswal S. K., Naamala J., Dakora F. D. (2018). Nature and mechanisms of aluminium toxicity, tolerance and amelioration in symbiotic legumes and rhizobia. Biol. Fertil. Soils 54, 309–318. doi: 10.1007/s00374-018-1262-0
Jensen H. L. (1942). Nitrogen fixation in leguminous plants. i. general characters of nodule-bacteria isolated from species of medicago and 95 Trifolium in Australia. Proc. Linn. Soc. New South Wales 66, 98–108.
Koeuth T., Versalovic J., Lupski J. R. (1995). Differential subsequence conservation of interspersed repetitive Streptococcus pneumoniae BOX elements in diverse bacteria. Genome Res. 5 (4), 408–418. doi: 10.1101/gr.5.4.408.html
Lane D. J. (1991). “16S/23S rRNA sequencing,” in Nucleic acid techniques in bacterial systematics. Eds. Stackebrandt E., Goodfellow M. (New York, NY: John Wiley and Sons).
Martínez-Romero E., Caballero-Mellado J. (1996). Rhizobium phylogenies and bacterial genetic diversity. Crit. Rev. Plant Sci. 15 (2), 113–140. doi: 10.1080/07352689.1996.10393183
Meier-Kolthoff J. P., Göker M. (2019). TYGS is an automated high-throughput platform for state-of-the-art genome-based taxonomy. Nat. Commun. 10 (1), 1–10. doi: 10.1038/s41467-019-10210-3
Miller J. H. (1972). Experiments in molecular genetics (New York: Cold Spring Harbor Laboratory), 466.
Nilsson J. F., Castellani L. G., Draghi W. O., Pérez-Giménez J., Torres Tejerizo G. A., Pistorio M. (2019). Proteomic analysis of rhizobium favelukesii LPU83 in response to acid stress. J. Proteome Res. 18 (10), 3615–3629. doi: 10.1021/acs.jproteome.9b00275
Pan H., Wang D. (2017). Nodule cysteine-rich peptides maintain a working balance during nitrogen-fixing symbiosis. Nat. Plants 3 (5), 1–6. doi: 10.1038/nplants.2017.48
Paradis E., Claude J., Strimmer K. (2004). APE: Analyses of phylogenetics and evolution in R language. Bioinformatics 20 (2), 289–290. doi: 10.1093/bioinformatics/btg412
Pasek S., Risler J. L., Brézellec P. (2006). Gene fusion/fission is a major contributor to evolution of multi-domain bacterial proteins. Bioinformatics 22 (12), 1418–1423. doi: 10.1093/bioinformatics/btl135
Price P. A., Tanner H. R., Dillon B. A., Shabab M., Walker G. C., Griffitts J. S. (2015). Rhizobial peptidase HrrP cleaves host-encoded signaling peptides and mediates symbiotic compatibility. Proc. Natl. Acad. Sci. U.S.A. 112, 15244–15249. doi: 10.1073/2Fpnas.1417797112
Richter M., Rosselló-Móra R., Oliver Glöckner F., Peplies J. (2016). JSpeciesWS: A web server for prokaryotic species circumscription based on pairwise genome comparison. Bioinformatics 32 (6), 929–931. doi: 10.1093/bioinformatics/btv681
Riviezzi B., Cagide C., Pereira A., Herrmann C., Lombide R., Lage M., et al. (2020). Improved nodulation and seed yield of soybean (Glycine max) with a new isoflavone-based inoculant of Bradyrhizobium elkanii. Rhizosphere 15, 100219. doi: 10.1016/j.rhisph.2020.100219
Seemann T. (2014). Prokka: Rapid prokaryotic genome annotation. Bioinformatics 30 (14), 2068–2069. doi: 10.1093/bioinformatics/btu153
Shi H., Sun G., Gou L., Guo Z. (2022). Rhizobia–legume symbiosis increases aluminum Resistance in alfalfa. Plants 11 (10), 1275. doi: 10.3390/plants11101275
Sotelo M., Irisarri P., Lorite M., Casaretto E., Rebuffo M., Sanjuán J., et al. (2011). Diversity of rhizobia nodulating Lotus corniculatus grown in northern and southern regions of Uruguay. Appl. Soil Ecol. 49, 197–207. doi: 10.1016/j.apsoil.2011.05.005
Tamura K., Nei M. (1993). Estimation of the number of nucleotide substitutions in the control region of mitochondrial DNA in humans and chimpanzees. Mol. Biol. Evol. 10 (3), 512–526. doi: 10.1093/oxfordjournals.molbev.a040023
Tamura K., Stecher G., Kumar S. (2021). MEGA11: Molecular evolutionary genetics analysis version 11. Mol. Biol. Evol. 38 (7), 3022–3027. doi: 10.1093/molbev/msab120
Tartaglia C., Azziz G., Lorite M. J., Sanjuán J., Monza J. (2019). Phylogenetic relationships among introduced and autochthonous rhizobia nodulating Trifolium spp. in Uruguayan soils. Appl. Soil Ecol. 139, 40–46. doi: 10.1016/j.apsoil.2019.03.014
Torres Tejerizo G., Rogel M. A., Ormeño-Orrillo E., Althabegoiti M. J., Nilsson J. F., Niehaus K., et al. (2016). Rhizobium favelukesii sp. nov., isolated from the root nodules of alfalfa (Medicago sativa l.). Int. J. @ System. Evolut. Microbiol. 66 (11), 4451–4457. doi: 10.1099/ijsem.0.001373
Torres Tejerizo G. T., Del Papa M. F., Draghi W., Lozano M., Giusti M. de los Á., Martini C., et al. (2011). First genomic analysis of the broad-host-range Rhizobium sp. LPU83 strain, a member of the low-genetic diversity Oregon-like rhizobium sp. group. J. @ Biotechnol. 155 (1), 3–10. doi: 10.1016/j.jbiotec.2011.01.011
Torres Tejerizo G. T., Wibberg D., Winkler A., Ormeño-Orrillo E., Martínez-Romero E., Niehaus K., et al. (2017). Genome sequence of the symbiotic type strain Rhizobium tibeticum CCBAU85039T. Genome Announcements 5 (4), e01513–e01516. doi: 10.1128/genomeA.01513-16
Vincent J. M. (1970). A manual for the practical study of root-nodule bacteria (Oxford: Blackwell Scientific Press).
Wegener C., Schröder S., Kapp D., Pühler A., Segundo López E., Martínez-Abarca F., et al. (2001). A population of twelve acid tolerant alfalfa nodulating Rhizobium strains isolated from different sites in Argentina exhibit the same molecular characteristics as the Rhizobium sp. strain Or191. Symbiosis 30, 141–162.
Wilson K., Sessitsch A., Corbo J., Giller K., Akkermans A., Jefferson R. (1995). B-glucuronidase (GUS) transposons for ecological and genetic studies of rhizobia and other gram-negative bacteria. Microbiology 141 (7), 1691–1705. doi: 10.1099/13500872-141-7-1691
Yu G., Smith D. K., Zhu H., Guan Y., Lam T. T. Y. (2017). Ggtree: an r package for visualization and annotation of phylogenetic trees with their covariates and other associated data. Methods Ecol. Evol. 8 (1), 28–36. doi: 10.1111/2041-210X.12628
Keywords: rhizobia, Oregon-like strain, competitiveness, alfalfa, M16 peptidases
Citation: Berais-Rubio A, Morel Revetria MA, Giménez M, Signorelli S and Monza J (2023) Competitiveness and symbiotic efficiency in alfalfa of Rhizobium favelukesii ORY1 strain in which homologous genes of peptidases HrrP and SapA that negatively affect symbiosis were identified. Front. Agron. 4:1092169. doi: 10.3389/fagro.2022.1092169
Received: 07 November 2022; Accepted: 02 December 2022;
Published: 04 January 2023.
Edited by:
Basharat Ali, Khwaja Fareed University of Engineering and Information Technology (KFUEIT), PakistanReviewed by:
Shahbaz Atta Tung, Pir Mehr Ali Shah Arid Agriculture University, PakistanLing Xu, Zhejiang Sci-Tech University, China
Copyright © 2023 Berais-Rubio, Morel Revetria, Giménez, Signorelli and Monza. This is an open-access article distributed under the terms of the Creative Commons Attribution License (CC BY). The use, distribution or reproduction in other forums is permitted, provided the original author(s) and the copyright owner(s) are credited and that the original publication in this journal is cited, in accordance with accepted academic practice. No use, distribution or reproduction is permitted which does not comply with these terms.
*Correspondence: Jorge Monza, am1vbnphQGZhZ3JvLmVkdS51eQ==
†ORCID: Andrés Berais-Rubio, orcid.org/0000-0002-0259-9112
María A. Morel Revetria, orcid.org/0000-0002-9064-5675
Matías Giménez, orcid.org/0000-0002-6267-9106
Santiago Signorelli, orcid.org/0000-0002-1854-3164
Jorge Monza, orcid.org/0000-0002-4309-7397