- 1University Hospital Coventry & Warwickshire, Coventry, United Kingdom
- 2Aintree University Hospital, Liverpool, United Kingdom
Three-dimensional (3D) printing allows rapid prototyping of novel equipment as well as the translation of medical imaging into tangible replicas of patient-specific anatomy. The technology has emerged as a versatile medium for innovation in medicine but with ever-expanding potential uses, does 3D printing represent a valuable adjunct to urological practice? We present a concise systematic review of articles on 3D printing within urology, outlining proposed benefits and the limitations in evidence supporting its utility. We review publications prior to December 2019 using guidelines outlined by the Preferred Reporting Items for Systematic Reviews and Meta-Analysis (PRISMA) statement. Of 117 identified articles, 67 are included highlighting key areas of research as the use of patient-specific models for patient education, surgical planning, and surgical training. Further novel applications included printed surgical tools, patient-specific surgical guides, and bioprinting of graft tissues. We conclude to justify its adoption within standard practice, further research is required demonstrating that use of 3D printing can produce; direct and measurable improvements in patient experience, consistent evidence of superior surgical outcomes or simulation which surpasses existing means' both in fidelity and enhancement of surgical skills. Although exploration of 3D printing's urological applications remains nascent, the seemingly limitless scope for innovation and collaborative design afforded by the technology presents undeniable value as a resource and assures a place at the forefront of future advances.
Introduction
Stereolithography or additive manufacturing, describes forming three-dimensional (3D) objects by step-wise layered addition of material. 3D printing allows successive production of structurally distinct objects instead of the mass production of identical items typically achieved by subtractive manufacturing (1).
This underpins the primary rationale for 3D printings' increasing utilization within urology: As every patient is unique, both their surgical treatment and adjuncts to it should be similarly individualized. 3D printing can utilize data from medical imaging to produce structures customized from and for a patients' individual anatomy (2). Furthermore, products can be functionally tested then quickly adapted in the next iteration.
Three-dimensional printing has the potential to enhance patient-specificity of pre-operative counseling, surgical simulation, implantable prostheses and even transplant organs (3). Customizable surgical instruments could be shared digitally, adjusted to preference and produced as required (3, 4). However, with printers used in surgical research ranging in price from $2000 to $900,000 this resource requires investment (5). Maintenance, cartridge consumables, specialist software for converting medical imaging into printable format and computer hardware further add to setup costs.
With current literature detailing diverse urological applications, distinguishing potential from proven advantage is key to guiding future practice and research alike. We therefore aim to elucidate 3D printing's confirmed benefits to patient and clinician whilst highlighting those requiring further evidence. Please refer to the Supplementary Material for details on review methodology (6). Figures 1 and 2 Illustrate products of 3D printing and a range of 3D printers.
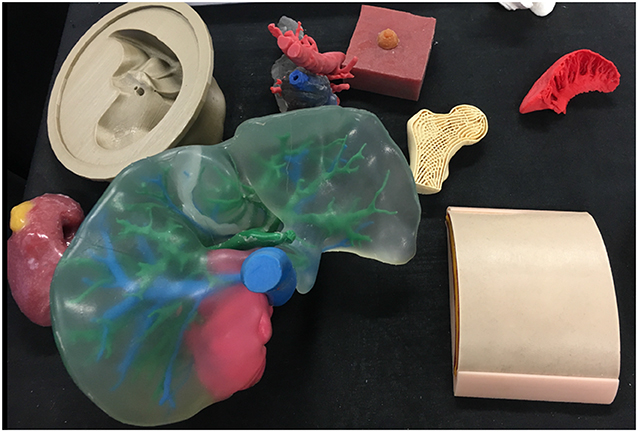
Figure 1. A selection of models made via 3D printing or 3D printed molds including a renal tumor model (left), liver (center), femoral head (right), and skin (right).
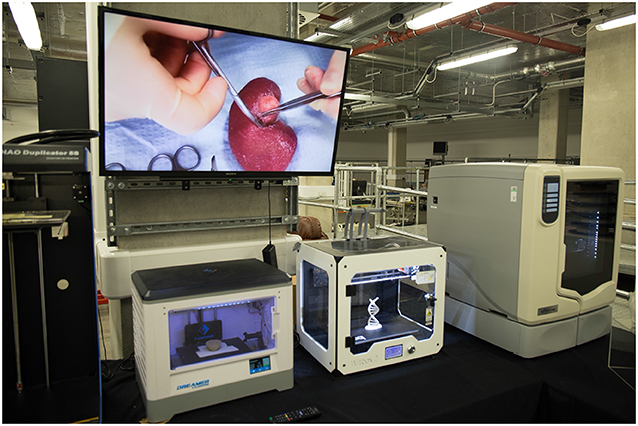
Figure 2. A selection of three-dimensional printers in use at 3D LifePrints hub at Alder Hey Childrens Hospital, Liverpool.
Patient Education
Coupling medical 3D imaging with additive manufacturing allows accurate modeling of individual patients' anatomy by converting DICOM (digital imaging and communications in medicine) data to stereolithography (STL) format (2). Bernard created kidney models with transparent resin for renal parenchyma showing tumor, vasculature, and collecting system in patients awaiting partial nephrectomy. These reportedly improved patients understanding of kidney physiology, anatomy, tumor characteristics, and the planned procedure (7). Altahay used 3D-printed models prior to percutaneous nephrolithotomy (PCNL), reporting similar improvements in patient understanding as assessed by questionnaires before and after counseling with the 3D model (8).
Teishima demonstrated superior questionnaire-assessed understanding in both patients and family members prior to partial nephrectomy when comparing explanation with 3D-printed models to using computerized tomography (CT) alone (9). Whereas Schmit reported no statistically significant improvement in patient understanding from explanation of cryoablation with 3D models compared to 2D imaging after correcting for different counseling physicians (10).
In patients undergoing partial nephrectomy or radical prostatectomy, Wake et al. compared counseling with standard imaging to imaging plus either a 3D printed model, augmented reality model or 3D computer model (11). Patient understanding was assessed via Likert scale survey with 3D-printed models and 3D computer models receiving higher scores than the control group. Only the 3D-printed model showed statistically significant improvements, including in patient-rated comfort with the surgical plan thus establishing a link between patients understanding and anxiety. A summary of reviewed articles relating to patient education is provided in Table 1.
Surgical Planning
In Zhang's study, models of patients' kidneys with T1 tumors received positive face validation scores by experienced Urologists for representation of tumor size and inter-related structures but details of renal vasculature and the collecting system were scored less favorably (12). Silberstein presented cases of T1 renal tumors where 3D-printed models were used as a real-time reference for surgeons when performing reconstruction and assessing resection in relation to the hilar vessels and collecting system (13). Surgical outcomes were reported but with no control arm for comparison. Komai reported outcomes for partial nephrectomies with nephrometry scores ≥8 where a “4D” printed model was used in surgical planning (14). The proposed 4th dimension was time as the model included a removable tumor with a 2–5 mm margin to assess the defect in relation to renal structures but similarly no control arm was included to indicate superior outcomes.
Wake et al demonstrated 3D models could (a) change experienced urologist's planned surgical approach for treatment of a renal tumor and (b) the planned approach based on a 3D-printed model was more likely to be followed than one based on 2D imaging. (15).
Maddox used patient-specific models to simulate robot-assisted partial nephrectomies prior to the actual procedure and outcomes were compared to the Tulane Urology prospectively maintained database. Cases using the surgical models had larger tumors (4.3 vs. 3.4 cm, p = 0.4), fewer complications (0 vs. 20%), longer warm ischemic time (25 vs. 21.6 min, p = 0.9), fewer positive margins (0 vs. 7.4%) and shorter hospitalization time (1.86 vs. 2.4 days, p−0.12). The only statistically significant difference however was a lower estimated blood loss (185.7 vs. 235.6 ml, p = 0.01) (16). Kyung et al. compared the outcomes for partial nephrectomy aided by prior inspection of a 3D-printed model against a control cohort and similarly the only significant difference was reduced estimated blood loss (17) whereas, Fan reported a significant reduction in warm ischemic time (18).
Twelve studies described using 3D-printing in prostate cancer diagnosis and management but only 2 addressed patient-tailored pre-operative planning; Shin used pre-biopsy prostatic MRI's of patients to construct translucent models of the prostate including the biopsy-proven malignant lesion and neurovascular bundles (NVB). The relationship between prostate, lesion and NVB was assessed using these before performing nerve-sparing radical robotic prostatectomy. A wider (1 mm) area of periprostatic tissue was dissected at regions high risk for extra-capsular extension based on the models. Although high risk cases (pT2c [n = 1], pT3a [n = 2], and pT3b [n = 2]), histopathology confirmed all having negative surgical margins (19). Wang used translucent prostate models with visible MRI-identified lesions to aid cognitive prostate biopsy. Standard systematic biopsies were taken, followed by 2–3 targeted biopsies. A higher positive biopsy rate was reported in the targeted biopsies and higher-grade disease was identified (20).
We identified 3 studies using 3D-printing for patient-specific aids to percutaneous nephrolithotomy (PCNL). Atalay constructed anatomically accurate models of patients with complex staghorn calculi and presented these to surgical residents before PCNL. Their understanding of the collecting system anatomy, stone location and optimal entry calyx was assessed via questionnaire with reported improvement in all categories (21). Xu created copies of patients renal anatomy, containing 3D-printed facsimiles of their stones and simulated the procedure using different puncture sites to determine the optimal approach. CT-assessed stone clearance with the models correlated well with procedures using the same puncture sites (22). Golab used a virtual 3D model of a patient with a horseshoe kidney and bilateral stones to determine the optimal puncture site and angle for PCNL, then printed a sterilisable surgical guide (23). The guide was positioned using vertebral spinous processes as markers and achieving nephroscopic access in this challenging case reportedly took 3 min.
This method of surgical guide has been utilized to implant tined leads for sacral nerve modulation with Zhang et al. reporting reduced punctures, procedural time and fluoroscopy use (24). A statistically significant reduction in effective voltage after using the surgical guide also indicated a more optimally positioned lead. An expert consensus guideline by Shaito and Ye detail potential use of 3D-printed templates for brachytherapy seed implantation (25). A summary of reviewed articles relating to surgical planning is provided in Table 2.
Surgical Training
Gasior detailed 3D-printings use in improving understanding of complex cloacal anomalies for trainees and faculty surgeons alike (26). Tangible 3D-printed model demonstrated improved questionnaire-assessed understanding compared to 2D cloacagrams, rotatable 3D virtual models and 3D video animations.
Simulation with 3D-printed anatomical models has been a focus of research, offering patient-specific representation of pathology and tactile feedback. Simulation utilizing 3D-printing has been described for partial nephrectomy, pyeloplasty, PCNL, robot-assisted prostatectomy, robot-assisted kidney transplant, vasectomy reversal, and transurethral resection of the prostate (TURP).
Adams created “phantom” models of cadaveric kidneys using 3D-printed molds designed from CT imaging. These were compared to the original in ultrasound and CT appearances as well as hardness, elasticity, and tensile strength. Different materials were trialed and the water-based gel, Agarose proved most similar to human kidney tissue (27). Melnyk recently created poly-vinyl alcohol (PVA) kidney phantoms using 3D-printed casts derived from a patient with a 4.2 cm exophytic renal tumor (28). Renal vasculature and collecting system were constructed from PVA and models were tested against porcine equivalents in mechanical properties & flow characteristics of the simulated vasculature. The group also compared suture tension required to approximate renal parenchymal edges and the maximum tension at which suture tension tore through parenchyma. Testing identified 7% PVA models with a three freeze-thaw cycle as the formula best replicating porcine tissue. The models were set amongst fabricated peritoneum, abdominal fat, spleen, bowel and mesentry to form an immersive simulation of robot-assisted partial nephrectomy. Similarly, Choi created prostate phantoms for simulation of TURP using PVA, agar and hollow glass powder, testing compressive & elastic properties. Models with different agar percentages were compared to normal, cancerous, and hyperplastic prostate tissue (29) with the physical properties of these simulation models being demonstrated as adjustable.
Ghazi's PVA hydrogel models of the kidney and adjacent structures allowed immersion simulation of PCNL with experts (caseload >100) and novices (caseload <20) rating it highly in similarity to the real procedure and usefulness in training. The models' realism was further supported by significant superiority from experts compared to novices in mean fluoroscopy time, number of percutaneous access attempts and stone clearance (30). Cheung et al printed molds of renal anatomy with pelvic ureteric junction obstruction and cast silicone rubber models for low-cost, reusable simulation of laparoscopic pyeloplasty (31). The simulation was validated on a 5-point Likert scale, scoring 4.75 (± 0.29), 4.50 (± 0.41), and 4.38 (± 0.48) in overall impression, realism and handling, respectively. Shee used printed casts to mold a surrogate bladder neck and urethra from silicone for an ex-vivo simulation trainer for robotic vesicourethral anastomosis (32) with an average face validity rating of 8/10 and content validity of 10/10. Experts and trainees rated the simulation superior to digital virtual reality (VR) trainers and experts performed better than residents in the simulation. Uwechue developed a 3D-printed simulation model for vascular anastomosis during robot-assisted kidney transplant, highlighting potential training value even amongst experienced surgeons when learning new surgical techniques (33). Pinto reported an observed improvement in residents microsurgical suture time and quality on a vasectomy reversal model produced via 3D-printing (41). However, as Monda et al commented in their paper on partial nephrectomy simulation, future studies need to establish that improved performance on simulation models is associated with improvements in live surgery (42).
Simulation presents a reproducible and uniform means to assess trainee performance both subjectively and with quantitative metrics. The aforementioned TURP model by Choi used materials with different ultrasound echogenicity for the central and peripheral zones of the prostate so that the resected volume of each zone could be assessed (29). Qiu incorporated tactile sensors into 3D-printed prostate models able to calculate pressure forces applied to the model (43). Witthaus et al. constructed a simulation model for robot-assisted radical prostatectomy using a chemiluminescent dye-impregnated PVA hydrogel model of the prostate and a tension wire sensor incorporated within the neurovascular bundle (44). The tension wire provided quantitative measurement of tension applied to the NVB during nerve-sparing prostatectomy and the dye allowed assessment of the surgical margin. Further metrics included the urethrovesical anastomosis leak test and task-specific times. When compared against assessment via a Global Evaluative Assessment of Robotic Skills (GEARS) and Robotic Anastomosis Competency Evaluation (RACE), higher GEARS score tallied with lower exerted force on the NVB and higher RACE scores correlated with a lower UVA leak rate. Witthaus' paper highlighted novel ways in which 3D-printing and simulation can be designed to objectively assess trainees.
Parkhomenko et al aided surgical simulation in a different way by designing a 3D-printable, portable laparoscopic trainer with a reported production cost of $26.50 and assembly time of <45 min (4). Whilst conventional trainers were scored higher by trainees, all still reported it as useful and this study demonstrated how 3D-printable designs can easily be shared across institutions for immediate production. A summary of reviewed articles relating to surgical training is provided in Table 3.
Patient-Specific Prostheses & Bioprinting
Outside of urology 3D-printing has been used for customized orthopedic plate sizing and molding (45) as well as for titanium and ceramic patient-specific maxillofacial implants (46). 3D-printed implants in urology are limited by the unique mechanical and physiological functions of the genitourinary tract and further complicated by the need to be sterilisable. Patient-specific 3D-printed extravascular stent have been used to treat posterior nutcracker syndrome (47) (48) but these do not emulate any urological structure. CT imaging was used to design a custom stent, 3D-printed from a titanium alloy and laparoscopically sited around the retro-aortic left renal vein to prevent compression.
However, substitutional grafts within the genitourinary tract remain possible via bioprinting. Organ production from native tissue over a scaffold was demonstrated in the Vacanti mouse where a chondrocyte-seeded, ear-shaped scaffold was implanted beneath its skin (49). Atala similarly used urothelial and smooth muscle cells obtained from bladder biopsy to ‘grow’ autologous tissue around a biodegradable bladder-shaped collagen scaffolds which were then successfully used for cystoplasty (50). Huang reported using 3D porous bacterial cellulose scaffolds seeded with rabbit lingual keratinocytes as a material for urethral reconstruction (51). These original cellulose scaffolds were not produced via 3D-printing but an integrated tissue-organ printer (ITOP) system was later used by Khang to print a porous, spiral scaffold dispersed with rabbit urothelial and smooth muscle cells within a fibrin hydrogel. This bioprinted urethra demonstrated mechanical properties equivalent to native rabbit urethra, with cells maintaining 80% viability at 7 days and demonstrating active proliferation (52). Versteegden also reported on collagen scaffolds produced via 3D-printing, reproducing the elasticity and shape-recovery of human urethral tissue (53). Yu investigated 3D-printed polycaprolactone (PCL) scaffolds and culture of human fibroblast cells for potential use as a surrogate for tunica albuginea (54) and Oh et al successfully cultured human aortic smooth muscle and umbilical vein endothelial cells over 3D-printed PCL scaffolds as a potential tissue-engineered corpus cavernosum graft (63).
Whilst these studies demonstrate how unique mechanical properties of the urinary tract can be emulated, a more significant development toward future autologous kidney replacement is the recent bioprinting of a renal proximal convoluted tubule. This involved printing a silicone gasket within a perfusable “3D tissue chip” and seeding it with immortalized human proximal tubular cells (61) forming a polarized epithelium, functional as a barrier and damaged by known nephrotoxins. A summary of reviewed articles relating to patient-specific prostheses and bioprinting is provided in Table 4.
Surgical Tools
Park published in vitro test results for a 3D-printed anti-reflux ureteric stent (60) whilst Junco et al created ureteric stents and laparoscopic trocars via 3D-printing, tested in porcine and cadaveric models. Junco was successful in producing 9F and 12F diameter stents which were deployable via a 0.035 guidewire but the smaller 7F stent did not allow passage of a guidewire and they were unable to print a stent with a tapered end. The 3D-printed trocars maintained pneumoperitoneum and allowed instrument passage but formed larger superficial skin defects than Karl Storz and Ethicon equivalents (64). Issues of biocompatibility, sterility, durability, tensile strength, and stent encrustation were not evaluated or addressed in the study.
Canvasser's pilot study of 3D-printed surgical clips proved inefficacious as they broke, failed to close and leaked more than commercially available alternatives (65). Rankin's military skin retractors 3D-printed using polylactic acid (PLA) filament were sterilisable via glutaraldehyde protocols and freshly printed retractors were sterile on bacterial testing via polymerase chain reaction (PCR). When stressed until fracture they could tolerate 13.6 kg of tangential force with no significant change after glutaraldehyde sterilization. Whilst insufficient for retraction of the abdominal wall, skin flaps or for orthopedic procedures this was fit for purpose as a skin retractor and low cost ($2.77 per retractor compared to the $23.48 stainless steel equivalent) (66). The cost of disposable equipment due to packaging, sterilization, transportation & storage could be overcome with on-site printing of tools and advantages would be greater in developing countries, where access to surgical equipment is limited by cost and transport. A summary of reviewed articles relating to surgical tools is provided in Table 5.
Novel Uses
Morimoto described a virtual reality design interface and 3D-printed concentric tube robot produced by this method. The surgeon-designed robot extended to curve beneath the 12th rib, into the kidney and upwards to the tumor (67). This prototyped a potential surgical instrument for tumor ablation but the study's focus was the interface which incorporated patient imaging and allowed the design and 3D-printing of patient-specific equipment by an individual surgeon.
3D-printed molds were used in two studies to standardize histological sampling of malignant renal and prostate specimens against imaging to validate the accuracy of imaging in identifying tumor location and likely histological findings (62, 68, 69). Antonelli used renal models to simulate PCNL and test a new “PercSac” device for capturing stones and fragments during the procedure (70).
Tse et al developed the “Endockscope” incorporating a cordless, light-emitting diode (LED) light source and a 3D-printed attachment for connecting smartphones to the flexible cystoscope eyepiece (71). Testing different smartphones against a Storz HD camera and xenon light, all were inferior to the standard system in image quality, brightness and color but combination with the Samsung S8 was uniformly rated by faculty urologists as acceptable for use.
Future Directions
Proposed applications for 3D printing within urology are expanding, with particular focus on tangible models of patient anatomy to enhance patient understanding, surgical planning and simulation training. The rapid increase in published research has been accompanied by related review articles. Smith and Dasgupta (72) reviewed applications within urological training whilst Checcuchi (73) provides a non-systematic review of both virtual and printed 3D models' utilization in robotic urological surgery. This review gives a concise overview of all reported uses of 3D printing across urology. Both Cacciamani's and Chen's systematic reviews (74, 75) provide a comprehensive exploration of published applications with expanded analyses of setup and manufacturing costs. However, we provide a more contemporary literature search and as a mini-review, a rapidly accessible outline of both the promising existing evidence and shortcomings in linking this to an improved service; Does elevating patient understanding reduce anxiety and litigation or improve recovery? Can inspection of or simulation with a patient-specific model improve surgical outcomes? Does simulation training with tangible facsimiles improve surgical training and with superiority to existing simulation models? These answers remain unproven and should underpin future research.
Regardless of how many prove durably useful, the scope of novel developments within urology showcase the freedom to innovate and rapidly prototype afforded by 3D printing. Whilst bioprinting promises major potential advances in patient-specific grafts in the future, we believe rapid prototyping coupled with network sharing and development of ideas is why 3D-printing will remain pivotal in ongoing surgical innovation. Table 6 provides an overview of all reported clinical applications and evidence limitations in the reviewed articles.
Author Contributions
DM read the referenced articles and wrote the first draft of the manuscript. AB and ML reviewed and edited the manuscript and approved the final version of the manuscript.
Conflict of Interest
The authors declare that the research was conducted in the absence of any commercial or financial relationships that could be construed as a potential conflict of interest.
Acknowledgments
We thank 3D LifePrints for giving permission to use photos taken at their printing hub in Alder Hey Children's Hospital, Liverpool.
Supplementary Material
The Supplementary Material for this article can be found online at: https://www.frontiersin.org/articles/10.3389/fsurg.2020.00029/full#supplementary-material
References
1. Pereira T, Kennedy J, Potgieter J. A comparison of traditional manufacturing vs additive manufacturing, the best method for the job. Procedia Manufacturing. (2019) 30:11–8. doi: 10.1016/j.promfg.2019.02.003
2. Wake N, Chandarana H, Huang WC, Taneja SS, Rosenkrantz AB. Application of anatomically accurate patient-specific 3D printed models from MRI data in urological oncology. Clin Radiol. (2016) 71:610–4. doi: 10.1016/j.crad.2016.02.012
3. Aimar A, Palermo A, Innocenti B. The role of 3D printing in medical applications: a State of the art. J Healthc Eng. (2019) 2019:5340616. doi: 10.1155/2019/5340616
4. Parkhomenko E, Yoon R, Okhunov Z, Patel RM, Dolan B, Kaler K, et al. Multi-institutional evaluation of producing and testing a novel 3D-Printed laparoscopic trainer. Urology. (2019) 124:297–301. doi: 10.1016/j.urology.2018.06.034
5. Hoang D, Perrault D, Stevanovic M, Ghiassi A. Surgical Applications for three-dimensional printing: a review of current literature and how to get started. Ann Transl Med. (2016) 4:456. doi: 10.21037/atm.2016.12.18
6. Moher D, Liberati A, Tetzlaff J, Altman DG. Preferred reporting items for systematic reviews and meta-Analyses: the PRISMA statement. Int J Surg. (2010) 8:658. doi: 10.1016/j.ijsu.2010.07.299
7. Bernard JC, Isotani S, Matsugasumi T, Duddalwar V, Hung AJ, Suer E, et al. Personalized 3D printed model of kidney and tumor anatomy: a useful tool for patient education. World J Urol. (2016) 34:337–45. doi: 10.1007/s00345-015-1632-2
8. Atalay HA, Canat HL, Ulker V, Alkan I, Ozkuvanci U, Altunrende F. Impact of personalized three-dimensional (3D) printed pelvicalyceal system models on patient information in percutaneous nephrolithotripsy surgery: a pilot study. Int Brazil J Urol. (2017) 43:470–5. doi: 10.1590/s1677-5538.ibju.2016.0441
9. Teishima J, Takayama Y, Iwaguro S, Hayashi T, Inoue S, Hiedka K, et al. Usefulness of personalised three-dimensional printed model on the satisfaction of preoperative education for patients undergoing robot-assisted partial nephrectomy and their families. Int Urol Neph. (2018) 50:1061–6. doi: 10.1007/s11255-018-1881-2
10. Schmit C, Matsumoto J, Yost K, Alexander A, Ness L, Kurup AN, et al. Impact of a 3D printed model on patients' understanding of renal cryoablation: a prospective pilot study. Abdom Radiol (NY). (2019) 44:304–209. doi: 10.1007/s00261-018-1710-1
11. Wake N, Rosenkrantz AB, Huang R, Park KU, Wysock JS, Samir S, et al. Patient-specific 3D printed and augmented reality kidney and prostate cancer models: impact on patient education. 3D Print Med. (2019) 5:4. doi: 10.1186/s41205-019-0041-3
12. Zhang Y, Ge HW, Li NC, Yu CF, Guo HF, Jin SH, et al. Evaluation of three-dimensional printing for laparoscopic nephrectomy of renal tuours: a preliminary report. World J. Urol. (2016) 34:533–7. doi: 10.1007/s00345-015-1530-7
13. Silberstein JL, Maddox MM, Dorsey P, Feibus A, Thomas R, Lee BR. Physical models of renal malignancies using standard cross-Sectional imaging and 3-Dimensional printers: a Pilot study. Urology. (2014) 84:268–72. doi: 10.1016/j.urology.2014.03.042
14. Komai Y, Sugimoto M, Gotohda N, Matsubara N, Kobayashi T, Sakai Y, et al. Patient-specific 3-dimensional printed kidney designed for “4D” surgical navigation: a Novel aid to facilitate minimally invasive off-clamp partial nephrectomy in complex tumour cases. Urology. (2016) 91:226–33. doi: 10.1016/j.urology.2015.11.060
15. Wake N, Rude T, Kang SK, Stifelman MD, Borin JF, Sodickson DK, et al. 3D printed renal cancer models derived from MRI data: application in pre-surgical planning. Abdom Radiol. (2017) 42:1501–9. doi: 10.1007/s00261-016-1022-2
16. Maddox MM, Feibus A, Liu J, Wang J, Thomas R, Silberstein JL. 3D-printed soft-tissue physical models of renal malignancies for individualized surgical simulation: a feasibility study. J Rob Surg. (2018) 12:27–33. doi: 10.1007/s11701-017-0680-6
17. Kyung YS, Kim N, Jeong IG, Hong JH, Kim CS. Application of 3-D printed kidney model in partial nephrectomy for predicting surgical outcomes: a Feasibility study. Clin Genitourin Cancer. (2019) 17:e878–e84. doi: 10.1016/j.clgc.2019.05.024
18. Fan G, Meng Y, Zhu S, Mingji Y, Mingfeng L, Feiping L, et al. Three-dimensional printing for laparoscopic partial nephrectomy in patients with renal tumours. J Int Med Res. (2019) 47:4324–32. doi: 10.1177/0300060519862058
19. Shin T, Ukimura O, Gill IS. Th1ree-dimensional printed model of prostate anatomy and targeted biopsy-proven index tumor to facilitate nerve-Sparing prostatectomy. Eur Urol. (2016) 69:376–80. doi: 10.1016/j.eururo.2015.09.024
20. Wang Y, Gao X, Yang Q, Wang H, Shi T, Chang Y, et al. Three-dimensional printing technique assisted cognitive fusion in targeted prostate biopsy. Asian J Urol. (2015) 2:214–9. doi: 10.1016/j.ajur.2015.09.002
21. Atalay HA, Ulker V, Alkan I, Canat HL, Ozkuvanci U, Altunrende F. Impact of three-Dimensional-Printed pelvicalyceal system models on residents' understanding of pelvicalyceal system anatomy before percutaneous nephrolithotripsy surgery: a pilot study. J Endourol. (2016) 30:1132–7. doi: 10.1089/end.2016.0307
22. Xu Y, Yuan Y, Cai Y, Li X, Wan S, Xu G. Use 3D printing technology to enhance stone free rate in single tract percutaneous nephrolithotomy for the treatment of staghorn stones. Urolithiasis. (2019). doi: 10.1007/s00240-019-01164-8. [Epub ahead of print].
23. Golab A, Smektala T, Krolikowski M, slojewski M. Percutaneous nephrolithotomy using an individual 3-dimensionally printed surgical guide. Urol Int. (2018) 100:485–7. doi: 10.1159/000446291
24. Zhang J, Zhang P, Wu L, Su J, Shen J, Fan H, et al. Application of an individualised and reassemblable 3D printing navigation template for accurate puncture during sacral neuromodulation. Neurourol Urodyn. (2018) 37:2776–81. doi: 10.1002/nau.23769
25. Saito S, Ye X. Expert consensus workshop report: guideline for three-dimensional-printing template-assisted computed tomography-guided i seeds interstitial implantation brachytherapy. J.Cancer Res Ther. (2017) 13:605–6. doi: 10.4103/jcrt.JCRT_540_17
26. Gasior AC, Reck C, Lane V, Wood RJ, Patterson J, Strouse R„, et al. Trancending dimensions: a comparative analysis of cloaca imaging in advancing the surgeons understanding of complex anatomy. J Digit Imaging. (2019) 32:761–5. doi: 10.1007/s10278-018-0139-y
27. Adams F, Qiu T, Mark A, Fritz B, Kramer L, Schlager D„ et al. Soft 3D-printed phantom of the human kidney with collecting system. Ann Biomed Eng. (2017) 45:963–72. doi: 10.1007/s10439-016-1757-5
28. Melnyk R, Ezzat B, Belfast E, Saba P, Farooq S, Campbell T„, et al. Mechanical and functional validation of a perfused, robot-assisted partial nephrectomy simulation platform using a combination of 3D printing and hydrogel casting. World J Urol. (2019). doi: 10.1007/s00345-019-02989-z. [Epub ahead of print].
29. Choi E, Adams F, Palagi S, Gengenbacher A, Schlager D, Muller PF, et al. A high-Fidelity phantom for the simulation and quantitative evaluation of transurethral resection of the prostate. Ann Biomed Eng. (2020) 48:437–46. doi: 10.1007/s10439-019-02361-7
30. Ghazi A, Campbell T, Melnyk R, Feng C, Andrusco A, Stone J, Etrurk E. Validation of a full-Immersion simulation platform for percutaneous nephrolithotomy using three-Dimensional printing technology. J Endourol. (2017) 31:1314–20. doi: 10.1089/end.2017.0366
31. Cheung CL, Looi T, Lendvay TS, Drake JM, Farhat WA. Use of 3-dimensional printing technology and silicone modelling in surgical simulation: development and face validation in paediatric laparoscopic pyeloplasty. J Surg Educ. (2014) 71:762–7. doi: 10.1016/j.jsurg.2014.03.001
32. Shee K, Koo K, Wu X, Ghali FM, Halter RJ, Hyams ES. A novel ex vivo trainer for robotic vesicourethral anastomosis. J Robot Surg. (2020) 14:21–7. doi: 10.1007/s11701-019-00926-1
33. Uwechue R, Gogalniceaunu P, Kessaris N, Byrne N, Chandak P, Olsburgh J, et al. A novel 3D-printed hybrid simulation model for robotic-assisted kidney transplantation (RAKT). J Robot Surg. (2018). 12:541–4. doi: 10.1007/s11701-018-0780-y
34. Glybochko PV, Rapoport LM, Alyaev YG, Sirota ES, Bezrukov EA, Fiev DN, et al. Multiple application of three-dimensional soft kidney models with localized kidney cancer: a pilot study. Urologia. (2018) 85:99–105. doi: 10.1177/0391560317749405
35. Libby RS, Silberstein JL. Physical model of clear-Cell renal carcinoma with inferior vena cava extension created from a 3-Dimensional printer to aid in surgical resection: a Case report. Clin Genitourin Cancer. (2017) 15:e867–e9. doi: 10.1016/j.clgc.2017.04.025
36. von Rundstedt FC, Scovell JM, Agrawal S, Zanaveld J, Link RE. Utility of patient-specific silicone renal models for planning and rehearsal of complex tumour resections prior to robot-assisted laparoscopic partial nephrectomy. BJU Int. (2017) 119:598–604. doi: 10.1111/bju.13712
37. Lee H, Nguyen NH, Hwang SI, Lee HJ, Hong SK, Byun SS. Personalized 3D kidney model produced by rapid prototyping method and its usefulness in clinical applications. Int Braz J Urol. (2018) 44:952–7. doi: 10.1590/s1677-5538.ibju.2018.0162
38. Kuroda S, Kawahara T, Teranishi J, Mochizuki T, Ito H, Uemura H. A case of allograft ureteral stone successfully treated with antegrade ureteroscopic lithotripsy: use of a 3D-printed model to determine the ideal approach. Urolithiasis. (2019) 47:467–71. doi: 10.1007/s00240-019-01153-x
39. Kusaka M, Sugimoto M, Fukami N, Sasaki H, Takenaka M, Anraku T, et al. Initial experience with a tailor-made simulation and navigation program using a 3-D printer model of kidney transplantation surgery. Transplant Proc. (2015) 47:596–9. doi: 10.1016/j.transproceed.2014.12.045
40. Srougi V, Rocha BA, Tanno FY, Almeida MQ, Baroni RH, Mendonca BB, et al. The use of three-dimensional printers for partial adrenalectomy: estimating the resection limits. Urology. (2016) 90:217–20. doi: 10.1016/j.urology.2015.11.043
41. Pinto L, Villacorta de Barros CA, Bentes de Lima A, Santos DR, Bacelar HPH. Portable model for vasectomy reversal training. Int Braz J Urol. (2019) 45:1013–9. doi: 10.1590/s1677-5538.ibju.2019.0092
42. Monda SM, Weese JR, Anderson BG, Vetter JM, Venkatesh R, Du K„, et al. Development and validity of a silicone renal tumor model for robotic partial nephrectomy training. Urology. (2018) 114:114–20. doi: 10.1016/j.urology.2018.01.030
43. Qiu K, Zhao Z, Haghiashtiani G, Guo SZ, He M, Su R, et al. 3D printed organ models with physical properties of tissue and integrated sensors. Adv Mater Technol. (2018) 3:1700235. doi: 10.1002/admt.201700235
44. Witthaus MW, Farooq S, Melnyk R, Campbell T, Saba P, Mathews E, et al. Incorporation and validation of clinically relevant performance metrics of simulation (CRPMS) into a novel full-immersion simulation platform for nerve-sparing robot-assisted radical prostatectomy (NS-RARP) utilizing three-dimensional printing and hydrogel casting technology. BJU Int. (2019) 125:322–32. doi: 10.1111/bju.14940
45. Duncan JM, Daurka J, Akhtar K. Use of 3D printing in orthopaedic surgery. BMJ. (2014) 9:348. doi: 10.1136/bmj.g2963
46. Zhou LB, Shang HT, He LS, Bo B, Liu GC, Liu YP„, et al. Accurate reconstruction of discontinuous mandible using a reverse engineering/computer-aided design/rapid prototyping technique: a preliminary study. J Oral Maxillofac Surg. (2010) 68:2115–21. doi: 10.1016/j.joms.2009.09.033
47. Wang H, Guo YT, Jiao Y, He DL, Wu B, Yuan LJ, et al. A minimally invasive alternative for the treatment of nutcracker syndrome using individualized three-dimensional printed extravascular titanium stents. Chin Med J (Engl). (2019) 123:1454–60. doi: 10.1097/CM9.0000000000000255
48. Guo YT, Wang He, Wang JP, Zhang B. Two-year follow-up on laparoscopic three-dimensional printed extravascular stent placement for posterior nutcracker syndrome. Chin Med J. (2018). 131:2895–6. doi: 10.4103/0366-6999.246075
49. Cao Y, Vacanti JP, Paige KT, Upton J, Vacanti CA. Transplantation of chondrocytes utilizing a polymer-cell construct to produce tissue-engineered cartilage in the shape of a human ear. Plast Reconstr Surg. (1997) 100:297–302. doi: 10.1097/00006534-199708000-00001
50. Atala A, Bauer SB, Soker S, Yoo JJ, Retik AB. Tissue-engineered autologous bladders for patients needing cystoplasty. Lancet. (2006) 367:1241–6. doi: 10.1016/S0140-6736(06)68438-9
51. Huang JW, Lv XG, Li Z, Song LJ, Feng C, Xie MK, et al. Urethral reconstruction with a 3D porous bacterial cellulose scaffold seeded with lingual keratinocytes in a rabbit model. Biomed Mater. (2015) 10:055005. doi: 10.1088/1748-6041/10/5/055005
52. Khang Z, Fu Q, Yoo J, Chen X, Chandra P, Mo X„, et al. 3D bioprinting of urethra with PCL/PLCL blend and dual autologous cells in fibrin hydrogel: an in vitro evaluation of biomimetic mechanical property and cell growth environment. Acta Biomater. (2017) 50:154–64. doi: 10.1016/j.actbio.2016.12.008
53. Versteegden LR, van Kampen KA, Janke HP, Tiemessen DM, Hoogenkamp HR, Hafmans TG, et al. Tubular collagen scaffolds with radial elasticity for hollow organ regeneration. Acta biomater. (2017). 52:1–8. doi: 10.1016/j.actbio.2017.02.005
54. Yu HS, Park J, Lee HS, Park SA, Lee DW, Park K. Feasibility of polycaprolactone scaffolds fabricated by three-Dimensional printing for tissue engineering of tunica albuginea. World J Mens Health. (2018) 36:66–72. doi: 10.5534/wjmh.17025
55. Smektala T, Golab A, Krolikowski M, Slojewski M. Low cost silicone renal replicas for surgical training - technical note. Arch Esp Urol. (2016) 69:434–6.
56. Knoedler M, Feibus AH, Lange A, Maddox MM, Ledet E, Thomas R, Silberstein JL. Individualized physical 3-dimensional kidney tumor models constructed from 3-dimensional printers result in improved trainee anatomic understanding. Urology. (2015) 85:1257–61. doi: 10.1016/j.urology.2015.02.053
57. Porpiglia F, Bertolo R, Checcucci E, Amparore D, Autorino R, Dasgupta P, et al. Development and validation of 3D printed virtual models for robot-assisted radical prostatectomy and partial nephrectomy: urologists' and patients' perception. World J Urol. (2018) 36:201–7. doi: 10.1007/s00345-017-2126-1
58. Sweet RM. The cREST simulation development process: training the next generation. J Endourol. (2017) 31:S69–S75. doi: 10.1089/end.2016.0613
59. Kim MJ, Chi BH, Yoo JJ, Ju YM, Whang YM, Chang IH. Structure establishment of three-dimensional (3D) cell culture printing model for bladder cancer. PLoS ONE. (2019) 14:e0223689. doi: 10.1371/journal.pone.0223689
60. Park CJ, Kim HW, Jeong S, Seo S, Park Y, Moon HS, Lee JH. Anti-Reflux ureteral stent with polymeric flap valve using three-Dimensional printing: an in vitro study. J Endourol. (2015) 29:933–8. doi: 10.1089/end.2015.0154
61. Homan KA, Kolesky DB, Skylar-Scott MA, Hermann J, Obuobi H, Moisan A, Lewis JA. Bioprinting of 3D convoluted renal proximal tubules on perfusable chips. Sci Rep. (2016) 6:34845. doi: 10.1038/srep34845
62. Dwivedi DK, Chatzinoff Y, Zhang Y, Yuan Q, Fulkerson M, Chopra R, et al. Development of a patient-specific tumor mold using magnetic resonance imaging and 3-Dimensional printing technology for targeted tissue procurement and radiomics analysis of renal masses. Urology. (2018) 112:209–14. doi: 10.1016/j.urology.2017.08.056
63. Oh KJ, Yu HS, Park J, Lee HS, Park SA, Park K. Co-culture of smooth muscle cells and endothelial cells on three-dimensional bioprinted polycaprolactone scaffolds for cavernosal tissue engineering. Aging Male. (2019) 9:1–6. doi: 10.1080/13685538.2019.1601175
64. del Junco M, Okhunov Z, Yoon R, Khanipour R, Juncal S, Abedi G, et al. Development and initial porcine and cadaver experience with three-Dimensional printing of endoscopic and laparoscopic equipment. J Endourol. (2015) 29:58–62. doi: 10.1089/end.2014.0280
65. Canvasser NE, De S, Koseoglu E, Lay AH, Sorokin I, Fernandez R. Three-dimensional printing of surgical clips: an in vitro pilot study and trial of efficacy. J Endourol. (2017) 31:930–3. doi: 10.1089/end.2017.0221
66. Rankin TM, Giovinco NA, Cucher DJ, Watts G, Hurwitz B, Armstrong DG. 3D printing surgical instruments: Are we there yet? J Surg Res. (2014) 182:193–7. doi: 10.1016/j.jss.2014.02.020
67. Morimoto TK, Greer JD, Hawkes EW, Hsieh MH, Okamura AM. Toward the design of personalized continuum surgical robots. Ann Biomed Eng. (2018) 46:1522–33. doi: 10.1007/s10439-018-2062-2
68. Rutkowski DR, Wells SA, Johnson B, Huang W, Jarrard DF, Lang JM, et al. Mri-based cancer lesion analysis with 3d printed patient specific prostate cutting guides am j Clin Exp Urol. (2019) 7:215–2.
69. Priester A, Natarajan S, Le JD, Garritano J, Radosavcev B, Grundfest W, et al. A system for evaluating magnetic resonance imaging of prostate cancer using patient-specific 3D printed molds. Am J Clin Exp Urol. (2014) 2:127–35.
70. Antonelli JA, Beardsley H, Faddegon S, Morgan MS, Gahan JC, Pearle MS, Cadeddu JA. A novel device to prevent stone fragment migration during percutaneous lithotripsy: results from an in vitro kidney model. J Endourol. (2016) 30:1239–43. doi: 10.1089/end.2016.0466
71. Tse C, Patel RM, Yoon R, Okhunov Z, Landman J, Clayman RV. The endockscope using next generation smartphones: “A global opportunity”. J Endourol. (2018) 32:765–70. doi: 10.1089/end.2018.0275
72. Smith B, Dasgupta P. 3D printing technology and its role in urological training. World J Urol. (2019). doi: 10.1007/s00345-019-02995-1
73. Checcucchi E, Amparore D, Fiori C, Manfredi M, Ivano M, Di Dio M, et al. 3D imaging applications for robotic surgery: an ESUT YAUWP review. World J Urol. (2019) 38:869–81. doi: 10.1007/s00345-019-02922-4
74. Cacciamani GE, Okhunov Z, Meneses AD, Rodriguez-Socarras ME, Rivas JG, Porpiglia F, et al. Impact of three-dimensional printing in urology: state of the art and future perspectives. A systematic review by ESUT-YAUWP group. Eur Urol. (2019) 76:209–1. doi: 10.1016/j.eururo.2019.04.044
Keywords: additive manufacturing, stereolithography, urology, surgery, three-dimensional, printing
Citation: Mathews DAP, Baird A and Lucky M (2020) Innovation in Urology: Three Dimensional Printing and Its Clinical Application. Front. Surg. 7:29. doi: 10.3389/fsurg.2020.00029
Received: 04 September 2019; Accepted: 23 April 2020;
Published: 02 June 2020.
Edited by:
Khurshid Ghani, University of Michigan, United StatesReviewed by:
Riccardo Campi, Careggi University Hospital, ItalySimone Albisinni, Université libre de Bruxelles, Belgium
Copyright © 2020 Mathews, Baird and Lucky. This is an open-access article distributed under the terms of the Creative Commons Attribution License (CC BY). The use, distribution or reproduction in other forums is permitted, provided the original author(s) and the copyright owner(s) are credited and that the original publication in this journal is cited, in accordance with accepted academic practice. No use, distribution or reproduction is permitted which does not comply with these terms.
*Correspondence: David A. P. Mathews, david.mathews@nhs.net