- 1Vascular Biology Group, Department of Biomedical Sciences, University of Copenhagen, Copenhagen, Denmark
- 2Molecular and Clinical Sciences Institute, St. George’s University of London, London, United Kingdom
Voltage-gated Kv7 potassium channels, encoded by KCNQ genes, have major physiological impacts cardiac myocytes, neurons, epithelial cells, and smooth muscle cells. Cyclic adenosine monophosphate (cAMP), a well-known intracellular secondary messenger, can activate numerous downstream effector proteins, generating downstream signaling pathways that regulate many functions in cells. A role for cAMP in ion channel regulation has been established, and recent findings show that cAMP signaling plays a role in Kv7 channel regulation. Although cAMP signaling is recognized to regulate Kv7 channels, the precise molecular mechanism behind the cAMP-dependent regulation of Kv7 channels is complex. This review will summarize recent research findings that support the mechanisms of cAMP-dependent regulation of Kv7 channels.
cAMP-Dependent Signaling
Regulators of cAMP: G Protein-Coupled Receptors
First discovered by Dr. Earl W. Sutherland in 1958 (Rall and Sutherland, 1958; Sutherland and Rall, 1958), cyclic adenosine monophosphate (cAMP) is well-recognized as an important intracellular secondary messenger molecule that can induce a cascade of events to influence cellular function (Patra and Brady, 2018). cAMP orchestrates numerous signal transduction pathways through the activation of several downstream effector proteins, resulting in a wide variety of cellular processes including gene transcription, cell growth and cell differentiation (Dumont et al., 1989; Sassone-Corsi, 1995; Mayr and Montminy, 2001; Sands and Palmer, 2008; Bacallao and Monje, 2015).
cAMP is created from ATP through the action of Adenylate cyclases (AC), of which there are nine membrane-bound isoforms (AC 1-9) and one soluble isoform (sASC). The characteristics of each isoform are summarized effectively in several reviews (Taussig and Gilman, 1995; Simonds, 1999; Hanoune and Defer, 2001; Sadana and Dessauer, 2009; Tresguerres et al., 2011; Halls and Cooper, 2017). Membrane-bound ACs are stimulated downstream of Gs-specific G protein-coupled receptor (GPCR) activation, while the sAC is insensitive to GPCR-dependent regulation (Braun and Dods, 1975; Braun et al., 1977; Forte et al., 1983). When a GPCR is activated by its extracellular ligand, it causes a conformational change in the receptor which activates the associated heteromeric G protein complex, consisting of an α, ß and γ subunit. Subsequently, the Gsα dissociates from the receptor and Gßγ subunits, activating AC and increasing cAMP levels (see Pierce et al., 2002 for a detailed review of this signaling pathway). ACs can also be regulated by other intracellular signals, including Ca2+ and PKC (see Halls and Cooper, 2017 for a detailed review).
Phosphodiesterase’s (PDEs) remain the only known route of cAMP degradation. The expression and localization of PDEs within a cell controls the magnitude and duration of cAMP-dependent events, as well as the compartmentalization and intracellular gradients of cAMP (Baillie, 2009). Thus, the expression and localization of PDEs in a cell contributes to the specificity of the cAMP-response following activation.
Effectors of cAMP: PKA and EPAC
cAMP mediates its effect by activation of effector proteins including protein kinase A (PKA), Epac (Exchange protein directly activated by cAMP), or cyclic nucleotide-gated channels (CNGCs) (Shabb, 2001; Bos, 2006; Biel and Michalakis, 2009). For many years it was believed that the effects of cAMP were mediated exclusively through the activation of intracellular PKA. PKA is a heterotetrameric holoenzyme consisting of two regulatory (R) subunits and two catalytic (C) subunits (Krebs and Beavo, 1979; Taylor et al., 2013; Turnham and Scott, 2016). There are two types of regulatory subunits, RI and RII, each consisting of two isoforms, RIα, RIβ, RIIα, RIIβ, each having different tissue expression, subcellular localization and cAMP binding affinity (Bechtel et al., 1977; Corbin et al., 1977, 1978; Zoller et al., 1979; Rannels and Corbin, 1980; Ringheim and Taylor, 1990; Taylor et al., 2012; Weigand et al., 2017). PKA that contains either RI or RII, form the two classes of PKA termed type I or type II, respectively. Binding of four cAMP molecules to each R subunit induces a conformational change and activates the kinase, resulting in the free catalytic subunits phosphorylating serine and threonine residues in specific substrate proteins (Welch et al., 2010).
Scaffolding proteins, such as A Kinase Anchoring Proteins (AKAPs; reviewed in Rubin, 1994; Scott et al., 2013; Dema et al., 2015) bind to the PKA-R subunits at the dimerization/docking domain at the N-terminus and targets PKA to specific subcellular locations to ensure specificity in signal transduction by placing PKA close to its appropriate substrate target, allowing compartmentalization of PKA (Scott et al., 1990; Carr et al., 1991; Bauman et al., 2006; Gold et al., 2006). AKAPs also bind other proteins such as protein kinase C and PDEs (Moleschi and Melacini, 2014). The AKAP family has more than 50 members and can be classified according to their binding specificity for the PKA-R subunits (Wong and Scott, 2004). The majority of the AKAPs bind to PKA-RII, however, several AKAPs bind to PKA-RI (Kinderman et al., 2006; Sarma et al., 2010). Only a few AKAPs have dual-specificity and can bind to both PKA-RI and PKA-RII, although with lower affinity for PKA-RI (Burns et al., 2003). This lower affinity for PKA-RI is due to the structural difference in the dimerization/docking domain in the N-terminal of RI (Huang et al., 1997; Gold et al., 2006). AKAPs are expressed in different tissues of the body and can facilitate tissue-specific signaling in many cells types including neurons (Glantz et al., 1992; Moita et al., 2002; Dell’Acqua et al., 2006), heart (Fink et al., 2001; Marx et al., 2002; Ruehr et al., 2004), and pancreas (Lester et al., 1997).
cAMP also activates Epac (Exchange protein directly activated by cAMP) (de Rooij et al., 1998; Kawasaki et al., 1998). Epac proteins, stimulated by cAMP binding, activate the Ras superfamily of small GTPases, Rap1 and Rap2. There are two known Epac proteins, Epac1 and Epac2, which are both present in almost all tissues but have different expression levels (Roscioni et al., 2008). Epac acts as guanine-nucleotide exchange factors and catalyzes the exchange of GDP for GTP on Rap1 and Rap2 with subsequent activation of these two GTPases (Cheng et al., 2008). This leads to activation of further downstream pathways, which will result in a variety of cellular functions, depending on specific tissue and cell type. In cardiac myocytes, for example, Epac plays a role in the cardiac Ca2+ regulation by increasing Ca2+ release from the sarcoplasmic reticulum (SR) via ryanodine receptor 2 (RyR2) and thereby effecting contraction (Fujita et al., 2017). Furthermore, a signaling pathway in which cAMP acts via Epac to modulate ion channel function has been identified (Renström et al., 1997; Kang et al., 2006; Stott et al., 2016). Additionally, Epac enhances the synaptic release of neurotransmitters following cAMP elevation in neurons (Shariati et al., 2016).
Cyclic nucleotide-gated channels (CNGCs) are also activated directly by cAMP signaling (Ludwig et al., 1998; Santoro et al., 1998). CNGCs are non-selective cation channels expressed in many tissues, including the heart, lung, kidney, pancreas, liver, spleen, testis and various neuronal systems (Kaupp and Seifert, 2002; Biel and Michalakis, 2007; Biel, 2009). CNGCs are activated by direct binding of cAMP or cGMP to the channel and activation of the channel results in the influx of extracellular cations causing depolarization of the cell membrane. One example of a CNGC is the hyperpolarization-activated and cyclic nucleotide–gated channels (HCN), which are expressed in the heart and the nervous system. These channels mainly conduct Na+ and K+ and are activated by a hyperpolarized membrane potential and stimulated by intracellular cyclic nucleotides. HCN channels are responsible for the “funny current” (If) in the heart and neurons, where they regulate pacemaker activity and neuronal firing (Difrancesco, 1986; DiFrancesco and Tortora, 1991; Moosmang et al., 1999; DiFrancesco, 2020).
KCNQ-Encoded Potassium Channels
There are 12 sub-families of voltage-gated potassium channels (Kv1-Kv12), within which the Kv7 potassium channel family consists of five isoforms encoded by the KCNQ1-5 genes (Kv7.1-Kv7.5) (Robbins, 2001; Gutman et al., 2005). Like all Kv channels, Kv7 channels are voltage-sensing channels and open and close upon changes in transmembrane potential to selectively let K+ ions pass through the channel. The Kv7 channels are comprised of four α-subunits, with each α-subunit consisting of six transmembrane (TM) spanning domains (S1-S6). S1-S4 form the voltage-sensing domain, while S5-S6 form the pore domain. Each α-subunit contains cytoplasmic amino (N) – and carboxyl (C) – termini, which are essential for channel tetramerization and channel regulation (Barros et al., 2012). In the C-terminus of the channel, a coiled-coil sequence motif is the major determinant of channel assembly (Jenke et al., 2003; Schwake et al., 2003, 2006). Assembly of all four α-subunits placed in a cylindrical arrangement creates a pore through which the K+ ions are conducted selectively (Heginbotham et al., 1992; Doyle et al., 1998). This assembly can be done with four identical α-subunits from the same Kv7 isoform to form homomeric channels, or by combining at least two types of α-subunits with other Kv7 isoforms to form heteromeric channels. Kv7.1 was thought to only form as a homotetrameric channel (Schwake et al., 2000); however, heterotetrameric assembly with Kv7.5 has been suggested (Oliveras et al., 2014). Kv7.3 homomers are weakly effective but form functional heterotetramers with Kv7.2 (Schroeder et al., 1998; Wang et al., 1998), Kv7.4 (Kubisch et al., 1999) and Kv7.5 (Schroeder et al., 2000a) subunits, and Kv7.4 heterotetramizes with Kv7.5 (Bal et al., 2008; Brueggemann et al., 2014b; Chadha et al., 2014; Jepps et al., 2015). Kv7 channels also interact with KCNE-encoded single transmembrane-spanning auxiliary protein subunits [KCNE1-5; also called MinK and MinK-related peptides (MiRPs)] that function as β or ancillary subunits (Abbott and Goldstein, 1998; McCrossan and Abbott, 2004; Abbott, 2016; Jepps, 2017). These KCNE proteins cannot form functional channels themselves but coassemble with Kv7 channels to modulate several functional properties of the channel, including membrane trafficking, voltage dependence, and kinetics of channel opening and closing (McCrossan and Abbott, 2004; Kanda and Abbott, 2012). Finally, Kv7 channels have a large cytoplasmic C-terminal composed of four segments (helix A-D) (Haitin and Attali, 2008; Xu et al., 2013; Barrese et al., 2018). In addition to coordinating channel tetramerization, several regulatory molecules interact with the C-terminal region to modulate Kv7 channel function, including calmodulin (CaM), phosphatidylinositol 4,5-biphosphate (PIP2), protein kinase C (PKC), protein kinase A (PKA), A-kinase-anchoring protein (AKAP), ankyrin G (Ank-G) and Nedd4-2 (summarized by Haitin and Attali (2008) and Barrese et al., 2018).
This review will now focus on the mechanisms of cAMP-dependent regulation of Kv7 channels and explore a possible role for this mechanism for therapeutic targeting in diseases associated with Kv7 channel dysfunction.
cAMP Regulation of Kv7 Channels
Kv7.1
Kv7.1 channels are expressed in several tissues throughout the body including the heart, uterus, and epithelial cells of the inner ear, pancreas, airways, gastrointestinal tract and kidneys. In the heart, Kv7.1 associates with the ancillary subunit KCNE1 to constitute the channel responsible for the late repolarizing current termed IKs (Barhanin et al., 1996; Sanguinetti et al., 1996). Kv7.1 also associates with KCNE1 in the inner ear, where they regulate auditory function and pancreatic acinar cells where activation of the Kv7.1-KCNE1 channel provides the driving force for Cl– secretion (Kim and Greger, 1999; Köttgen et al., 1999; Warth et al., 2002). In addition, Kv7.1 channels in the pancreatic ß-cells contribute to the regulation of insulin secretion, and loss of function mutations in the KCNQ1 gene leads to impaired ß-cell insulin secretion, which is associated with type 2 diabetes (Ullrich et al., 2005; Unoki et al., 2008; Yasuda et al., 2008; Yamagata et al., 2011; Liu et al., 2014; Torekov et al., 2014; Min Lee et al., 2017; Zhang et al., 2020). Furthermore, association of Kv7.1 with KCNE3 in epithelial tissues of the colon, small intestine and airways regulates the transport of water and salts (Schroeder et al., 2000b; Grahammer et al., 2001a, b; Vallon et al., 2005).
Kv7.1-KCNE1 channels have been studied extensively in cardiac myocytes where the channel is important for delayed cardiac repolarization and shapes action potential duration (Terrenoire et al., 2005). In addition, it is enhanced by sympathetic nerve activity through activation of β-adrenergic receptors (Bennett and Begenisch, 1987; Yazawa and Kameyama, 1990). Stimulation of the β-adrenergic receptor by noradrenaline released from the cardio-accelerator nerve causes an elevation of cAMP that increases IKs via PKA-dependent phosphorylation (Yazawa and Kameyama, 1990).
As mentioned previously, PKA actions are regulated by AKAPs, by positioning PKA and other cAMP-responsive enzymes in proximity to their substrates. In the heart, several AKAPs are expressed, including AKAP79/150 (human AKAP79/murine AKAP150), AKAP15/18δ and AKAP9. AKAP9, better known as yotiao, facilitates PKA-phosphorylation of serine 27 in the Kv7.1 N-terminus required for the proper functioning of the channel (Walsh et al., 1988; Walsh and Kass, 1991; Potet et al., 2001; Marx et al., 2002). Yotiao also associates with protein phosphatase 1 (PP1), phosphodiesterase (PDE) and AC to control IKs phosphorylation status (Terrenoire et al., 2009; Li et al., 2012). Yotiao binds directly to Kv7.1 C-terminus via a leucine zipper motif, an amino acid sequence in the C terminus of Kv7.1, and disruption of the complex by mutations in yotiao or KCNQ1 are associated with Long QT Syndrome (Kass et al., 2003; Fodstad et al., 2004; Chen et al., 2007). Besides acting as a scaffold protein, yotiao has a functional role that occurs after IKs phosphorylation. Studies revealed that cAMP-dependent activation of Kv7.1-KCNE1 depends not only on serine 27 phosphorylation but also requires the direct binding of yotiao to the Kv7.1 at the leucine zipper motif (Kurokawa et al., 2004; Chen et al., 2005; Chen and Kass, 2006). Furthermore, it was revealed that yotiao itself is a substrate for PKA phosphorylation and can be phosphorylated upon β adrenoceptor stimulation (Chen et al., 2005). Serine 43 was identified as target residue for PKA phosphorylation on yotiao, with mutations of this residue dampening cAMP effects on the IKs current, even though yotiao binding and phosphorylation of Kv7.1 channels were not affected. Taken together, these studies conclude that AKAP protein yotiao has a crucial and complex regulatory role on the cAMP-dependent activity of IKs channels (see Figure 1A).
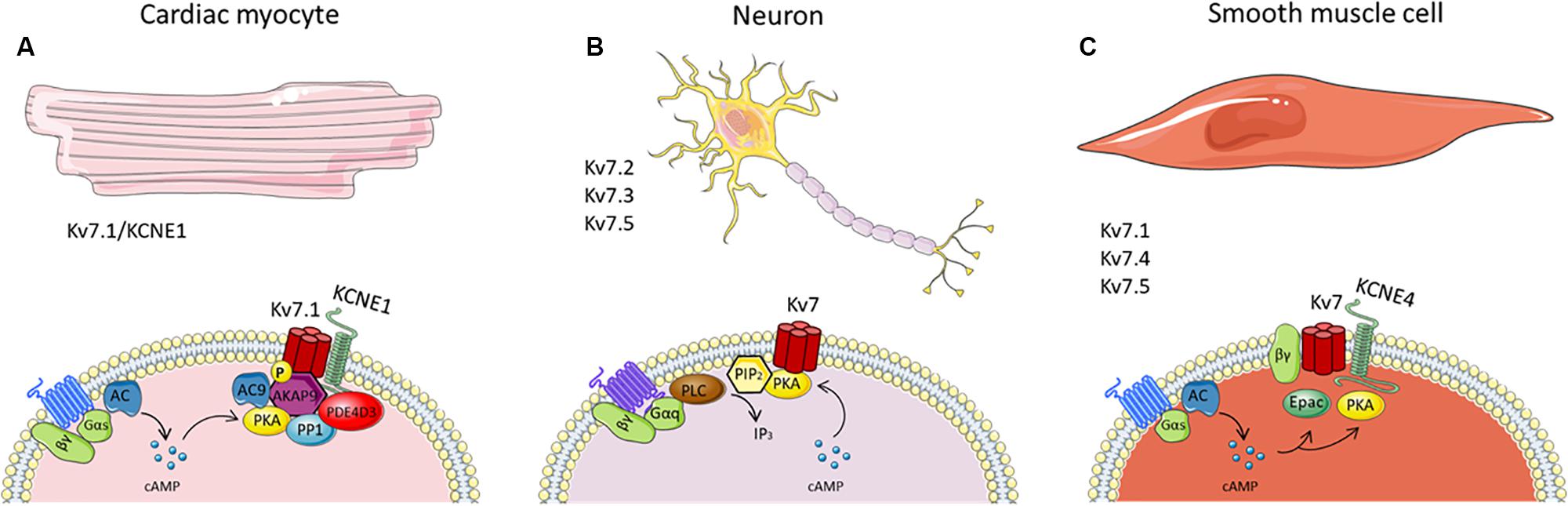
Figure 1. Schematics of known cAMP-dependent Kv7 channel regulation in panel cardiac (A), neurones (B) and smooth muscle cells (C). The figure summarizes many of the known interaction partners involved in the cAMP-mediated regulation of Kv7 channels in the different cells types, thereby highlighting the heterogeneity of cAMP-Kv7 channel signaling. It should be noted that, although AKAP79/150 is associated with Kv7.2 channels, it has not been included in the figure since there is no evidence of its involvement in PKA phosphorylation of the channel.
Although PKA activation of Kv7.1 following β adrenergic stimulation can increase IKs, chronic β adrenergic stimulation downregulates the IKs response (Mona et al., 2014). Chronic β adrenergic stimulation led to a significant decrease in KCNE1 but not KCNQ1 mRNA expression in guinea pig cardiomyocytes. Furthermore, chronic in vitro and in vivo isoprenaline stimulation reduced KCNE1 protein expression and KCNE1 membrane expression in guinea pig cardiomyocytes. This effect was mediated via Epac1 and not PKA activation, leading to increased translocation of nuclear factor of activated T cell (NFAT), which was responsible for KCNE1 downregulation (Mona et al., 2014).
In the inner ear, cAMP activates Kv7.1-KCNE1 channels, which results in the secretion of K+, necessary for normal hearing (Wang et al., 1996; Neyroud et al., 1997). However, the precise mechanisms underlying the cAMP-regulated increase in K+ conductance in the inner ear are unclear (Sunose et al., 1997). In the intestine, cAMP enhances Kv7.1-KCNE3 currents and stimulates Cl– secretion by hyperpolarizing the cell membrane and thereby amplifying the driving force for Cl– exit through cystic fibrosis transmembrane conductance regulator Cl– channels (Lohrmann et al., 1995; Diener et al., 1996; Suessbrich et al., 1996; Devor et al., 1997; Rufo et al., 1997; Schroeder et al., 2000b; Bajwa et al., 2007). An essential role for KCNE3 in cAMP-driven Cl– secretion has been suggested from the observation that KCNE3 knockdown reduced cAMP-mediated Cl– secretion across tracheal and intestinal epithelia without altering Kv7.1 expression (Preston et al., 2010) but the precise mechanism by which cAMP stimulates Kv7.1-KCNE3 channels in these cells is still unknown. Like for intestine epithelial cells, airway epithelial cells secrete Cl– stimulated by the cAMP-signaling pathway, where blockade of Kv7.1 channels suppress the cAMP-mediated Cl– secretion (Mall et al., 2000; Grahammer et al., 2001b; MacVinish et al., 2001; Cowley and Linsdell, 2002; Kim et al., 2007). However, the direct mechanism how cAMP mediates Kv7.1 channel activation responsible for Cl– secretion needs to be further investigated. In pancreatic β-cells, Kv7.1 channels contribute to insulin secretion. Besides, a link between cAMP and insulin secretion has established (Malaisse and Malaisse-Lagae, 1984; Seino and Shibasaki, 2005). However, it remains to be determined if Kv7.1 channels play a role in this cAMP-dependent mechanism.
Modifiers of cAMP-Mediated Regulation of Kv7.1 Channels
Although the direct regulation of cAMP signaling on Kv7.1 channel activity is well described, several factors can modulate this interaction indirectly. For instance, Nicolas et al. (2008) determined that the PKA-dependent regulation of IKs was microtubule-dependent (Nicolas et al., 2008). Cytoskeletal microtubules are essential for proper trafficking of cardiac ion channels to the plasma membrane (Steele and Fedida, 2014), and although a physical interaction between Kv7.1 and the microtubule forming protein, β-tubulin, was shown, disruption of the microtubules did not modify the Kv7.1-KCNE1 channel membrane density or baseline currents in transfected COS-7 cells or cardiomyocytes. However, microtubule disruption decreased the IKs response to PKA-mediated stimulation. This was not due to altered channel phosphorylation, yotiao phosphorylation or the interaction between both proteins, suggesting that microtubules play an important role in the coupling of PKA-dependent Kv7.1 phosphorylation and its channel activation (Nicolas et al., 2008).
The effect of cAMP on the trafficking of Kv7.1 channels has also been investigated. PKA inhibition in Madin-Darby canine kidney (MDCK) cells reduces Kv7.1 channel membrane expression and increases intracellular accumulation of the Kv7.1 protein in late endosomes/lysosomes (Andersen et al., 2015). This suggests a role for PKA-mediated trafficking of Kv7.1 channels, although this was not a result of channel phosphorylation. A previous study by the same group found that two cAMP phosphorylation residues on Kv7.1, serine 27 and serine 92, were not crucial for trafficking Kv7.1 (Lundby et al., 2013). Instead the E3 ubiquitin ligase Nedd4-2 is required in this PKA-dependent trafficking pathway (Andersen et al., 2015). Nedd4-2 is an ubiquitin-protein ligase that binds to ion channels containing a C-terminal proline-rich segment (PY motif) (Manning and Kumar, 2018). Among the Kv7 channels, Kv7.1 is the only isoform containing this sequence motif. Binding of Nedd4-2 to the C-terminal PY motif in Kv7.1 channels regulates the ubiquitylation of the channel and its internalization (Jespersen et al., 2007). Andersen et al. (2015) concluded that PKA regulated Nedd4-2-dependent trafficking of Kv7.1 but the precise mechanism how PKA influences Nedd4-2 needs to be determined (Andersen et al., 2015).
Kv7.2 and Kv7.3
Kv7.2 and Kv7.3 channels are widely expressed in neuronal tissues, where they form a heteromeric channel that generates the “M-current” (Brown and Adams, 1980; Wang et al., 1998; Wickenden et al., 2001; Roche et al., 2002; Shah et al., 2002). Kv7.5 channels are also a component of the M-channel in heteromeric formation with Kv7.3 (Lerche et al., 2000; Schroeder et al., 2000a; Tzingounis et al., 2010). The M-current in neurons maintains the negative resting membrane potential to limit neuronal firing and excitability (Marrion, 1997; Jentsch, 2000). M-currents are inhibited by Gq/11 coupled GPCRs such as muscarinic acetylcholine receptors M1, M3 and M5, or bradykinin B2 receptors. Stimulation of these receptors decreases M-current density resulting in depolarization, which subsequently increases neuronal excitability (Brown and Selyanko, 1985; Cruzblanca et al., 1998). It is well established that disruption of the M-current due to mutations in either KCNQ2 or KCNQ3 genes causes excessive neuronal excitability, leading to neuronal diseases, such as benign familial neonatal convulsions and epileptic encephalopathy (Biervert et al., 1998; Charlier et al., 1998; Singh et al., 1998; Jentsch, 2000; Castaldo et al., 2002; Borgatti et al., 2004; Weckhuysen et al., 2012, 2013).
Kv7.2/Kv7.3 currents in overexpressed Xenopus oocytes are enhanced by cAMP, which relies on PKA-mediated phosphorylation of serine 52 in the Kv7.2 N-terminus (Schroeder et al., 1998). Furthermore, Cooper et al. (2000) identified an interaction of Kv7.2 and PKA subunits in human brain samples by co-immunoprecipitation and affinity chromatography (Cooper et al., 2000). In addition, AKAP79/150 is associated with Kv7.2 channels (Hoshi et al., 2003, 2005; Zhang and Shapiro, 2012). Although the core AKAP79/150 complex contains PKA (Gold et al., 2006), it has not yet been determined whether AKAP70/150 facilitates PKA phosphorylation of Kv7.2 channel; however, AKAP79/150 is essential for the recruitment and phosphorylation of Kv7.2 channels by PKC (Hoshi et al., 2003; Higashida et al., 2005; Zhang and Shapiro, 2012).
Multiple other phosphorylation sites of Kv7.2/Kv7.3 channels have been identified using mass spectrometry. However, the responsible kinase for this phosphorylation remains elusive as not only PKA but also PKC and src tyrosine kinase can regulate Kv7.2/Kv7.3 channel phosphorylation (Gamper et al., 2003; Hoshi et al., 2003; Li et al., 2004; Surti et al., 2005). A recent study by Salzer et al. (2017) found 13 phosphorylation sites for human Kv7.2 using mass spectrometry, one already identified (serine 52) located at the N-terminus, whereas the remaining 12 were located in the C-terminus. Using in vitro phosphorylation assays the authors identified the protein kinases responsible for C-terminus Kv7.2 phosphorylation. Only two of the 12 residues (serine 438 and serine 455) were phosphorylated by PKA. Inhibition of PKA reduced Kv7.2 phosphorylation, which decreased channel sensitivity to PIP2 depletion, thereby attenuating Kv7 channel regulation via M1 muscarinic receptors. Thus, phosphorylation of the Kv7.2 channel is necessary to maintain a reduced affinity for PIP2 (Salzer et al., 2017; Figure 1B).
Kv7.5 channels are expressed in some regions of the brain, where they coassemble with Kv7.3 channels. Kv7.5/Kv7.3 channels show similar currents to the Kv7.2/Kv7.3 currents and are also inhibited by M1 muscarinic receptor activation (Schroeder et al., 2000a). Kv7.5 can be phosphorylated and stimulated by PKA on serine 53 on the N-terminus (Brueggemann et al., 2018); however, it is still unclear whether the heterotetrameric Kv7.3/Kv7.5 channel found in neurones are regulated in the same way by cAMP.
Kv7.4 and Kv7.5
Kv7.4 channels are expressed in the inner ear and auditory nerves (Kharkovets et al., 2000), the substantia nigra (Hansen et al., 2008), skeletal muscle cells (Iannotti et al., 2010), the mitochondria of cardiac myocytes (Testai et al., 2016) and in smooth muscle cells extensively (see Barrese et al., 2018 for a recent review of the smooth muscle literature). Kv7.5 channels are expressed in the brain, where they play a role in the regulation of neuronal excitability, and in skeletal and smooth muscle (Schroeder et al., 2000a; Roura-Ferrer et al., 2008; Iannotti et al., 2010; Haick and Byron, 2016).
In smooth muscle, there is evidence Kv7.4 and Kv7.5 channels exist as both homomers and heteromers (Brueggemann et al., 2011, 2014b; Chadha et al., 2014; Jepps et al., 2015). The first indication that smooth muscle Kv7 channels were activated by cAMP came from gastric smooth muscle cells. In these cells, β adrenoceptor stimulation with isoprenaline induced a current that resembled the neuronal M current, although at this time the molecular identity of the M current was still unknown (Sims et al., 1988).
In vascular smooth muscle, the use of pharmacological inhibitors and molecular interference has identified a role for Kv7 channels in receptor-mediated relaxations. The first identification was for β adrenoceptor-mediated vasodilatation in the renal vasculature (Chadha et al., 2012). Inhibition of Kv7 channels by XE991 or linopirdine and knockdown of Kv7.4 with KCNQ4-targeted small interfering RNA (siRNA), attenuated arterial relaxations to the β adrenoceptor agonist, isoprenaline (Chadha et al., 2012). More recent, experiments with morpholino-mediated suppression of Kv7.4 translation corroborated these findings (Stott et al., 2018). Furthermore, isoprenaline increased Kv7 currents in vascular smooth muscle cells isolated from rat arteries and in A7r5 cells (a rat aortic smooth muscle cell line) (Chadha et al., 2012; Stott et al., 2015; Mani et al., 2016; Brueggemann et al., 2018).
In hypertension, Kv7.4 protein expression is diminished causing reduced hyperpolarization and relaxation of the smooth muscle cells. Furthermore, in renal arteries from spontaneously hypertensive rats, where Kv7.4 expression is reduced, receptor-mediated relaxations were diminished (Jepps et al., 2011; Chadha et al., 2012). Interestingly, in the cerebral arteries from hypertensive rats, there is no compromise of Kv7.4 channel expression and CGRP receptor-mediated relaxations are unaffected (Chadha et al., 2014).
Although targeting Kv7.4 in isolated vessels with siRNA- and morpholino-induced knockdown inhibits isoprenaline- and CGRP-induced relaxations (Chadha et al., 2014; Stott et al., 2018), there is evidence that Kv7.5 is required to elicit cAMP-dependent relaxations. In A7r5 cells, stimulation of β adrenoceptors and the downstream pathway enhanced the currents produced by Kv7.5 channels and heterotetrameric Kv7.4/Kv7.5 channels expressed exogenously, but not when homomeric Kv7.4 channels were expressed (Mani et al., 2016), suggesting cAMP-mediated regulation of Kv7.4 and Kv7.5 depends on the stoichiometry of the channel.
The precise Kv7 channel stoichiometry eliciting cAMP-dependent relaxations in vascular smooth muscle is still unknown. Kv7.4 channels are important for proper Kv7 channel function in arteries, highlighted by their downregulation in arteries from hypertensive animals, whereas both Kv7.4 and Kv7.5 may play a role in the cAMP-dependent activation. To further complicate this mechanism, KCNE4 co-assembles with Kv7.4 and Kv7.5 in vascular smooth muscle cells to alter the biophysical properties and cellular localization of these channels. Targeted knockdown of Kcne4 in rat arteries depolarized the smooth muscle resting membrane potential and reduced vasorelaxations to Kv7 channel activators. Interestingly, in Kcne4 knockout mice, only the males displayed attenuated Kv7 channel function, but both male and female Kcne4 knockout mice had attenuated responses to isoprenaline. Given that Kcne4 expression has been shown in several arterial beds, it could be facilitating the Kv7 channel-dependent cAMP relaxations (Jepps et al., 2015; Abbott and Jepps, 2016). The expression of KCNE4 in A7r5 cells is yet to be determined but including KCNE4 subunits in future studies investigating the cAMP-dependent Kv7.4/Kv7.5 channel activation could give valuable insight into this mechanism.
Both PKA and Epac enhance Kv7 channel activity in vascular smooth muscle (Khanamiri et al., 2013; Mani et al., 2016; Stott et al., 2016). Isoprenaline-mediated relaxations were attenuated by PKA inhibition in renal arteries, whilst the isoprenaline relaxations in mesenteric arteries were not affected (Stott et al., 2016). In the mesenteric artery, isoprenaline-induced linopirdine-sensitive relaxations were elicited through activation of Epac, which is present in both rat renal and mesenteric arteries, but more predominant in mesenteric arteries. With electrophysiology experiments, Kv7 currents were increased with direct Epac stimulation in smooth muscle cells isolated from renal and mesenteric arteries (Stott et al., 2016). These data highlight that cAMP-dependent stimulation of Kv7 channels in vascular smooth muscle is not only dependent on the Kv7 channel architecture but also the channel’s association with downstream cAMP effector proteins, all of which are likely to be artery specific (see Figure 1C).
Kv7 channels are also expressed in airway smooth muscle cells (ASMCs) of rodents and humans. Pharmacological activators of Kv7 channels, including retigabine, flupirtine and S-1, relax airway smooth muscle cells from guinea pigs and human, and are important regulators of airway diameter (Brueggemann et al., 2012; Evseev et al., 2013). β2-adrenergic receptor agonists are commonly used for the treatment of asthma to relieve hyperconstriction of the airway (Cazzola et al., 2013), and are able to enhance Kv7 currents in ASMCs thereby inducing relaxation of rat airways (Brueggemann et al., 2014a). A later study by Brueggemann et al. (2018) revealed that β adrenoceptor stimulation activated the Kv7.5 channels in cultured human ASMCs, a response which was mediated by the PKA pathway (Brueggemann et al., 2018). This study identified serine 53 on the Kv7.5 N-terminus to be responsible for the increased Kv7.5 currents in response to cAMP elevation, which could be a common mechanism for β adrenoceptor/cAMP activation of Kv7.5 channels in vascular smooth muscle cells. Recent findings show that phosphorylation of serine 53 on the N-terminus of Kv7.5 increases its affinity for PIP2, corresponding to enhanced channel activation (Brueggemann et al., 2020).
Kv7.4 and Kv7.5 are also expressed in the smooth muscle of the gastrointestinal tract (Ohya et al., 2002; Jepps et al., 2009; Ipavec et al., 2011; Adduci et al., 2013), bladder (Streng et al., 2004; Rode et al., 2010; Svalø et al., 2012, 2013; Afeli et al., 2013; Anderson et al., 2013), penis (Jepps et al., 2016) and uterus (McCallum et al., 2009) where important functional roles have been established. Whether Kv7 channels in these tissues are subjected to the same cAMP-dependent regulation as in vascular and airway smooth muscle remains to be determined. Given that, even within the vasculature, there are differences in the cAMP-dependent mechanisms that lead to Kv7 channel stimulation, the Kv7 channels in these smooth muscle tissues cannot be assumed to behave in the same way as has been described in airway and vascular smooth muscle. Future work should investigate how Kv7 channels are subjected to cAMP-dependent regulation in these tissues, which could lead to novel therapeutic strategies for the treatment of various smooth muscle disorders, such as constipation, preeclampsia, erectile dysfunction and incontinence.
Modifiers of cAMP-Mediated Regulation of Kv7.4 Channels
Gβγ subunits have a regulatory role on the modulation of Kv7.4 channels in vascular smooth muscle cells, which is Gβ subunit-specific (Stott et al., 2015, 2018; Greenwood and Stott, 2020). Gβγ subunit binding to Kv7.4 channels enhances the current in both HEK and CHO cells expressing Kv7.4, and in rat renal arterial smooth muscle cells. In addition, Gβγ subunit interaction is critical for the β adrenergic dependent activation of Kv7 channels in rat renal arteries, but not in rat mesenteric arteries. Contrarily, inhibition of Gβγ subunits attenuated the CGRP dependent relaxations in mesenteric arteries, but showed no effect on CGRP relaxation in cerebral arteries, highlighting the role of Gβγ in Kv7-dependent vasorelaxations to be artery specific and dependent on the vasorelaxant (Stott et al., 2018).
The microtubule network has also been implicated in the Kv7 channel-dependent cAMP-mediated relaxations in rat mesenteric and renal arteries (Lindman et al., 2018). Disruption of the microtubule network with colchicine increased the membrane expression of Kv7.4 channels, which enhanced membrane hyperpolarization, decreases in intracellular Ca2+ and vasorelaxation to isoprenaline and forskolin in a Kv7 channel-dependent manner, specifically (Lindman et al., 2018). Further work in this area is required to determine whether the microtubule network regulates cAMP signaling in smooth muscle cells.
Conclusion
The second messenger cAMP regulates Kv7 channel activity in many cell types, including cardiac myocytes, smooth muscle and neurons. However, cAMP-mediated regulation of Kv7 channels is complex and many aspects remain to be elucidated. Generation of cAMP results from stimulation of AC, of which 9 isoforms exist. Depending on the isoforms expressed in a cell type, extracellular signals through GPCR activation can be integrated differently. Identifying how different AC isoforms contribute to cAMP-dependent activation of Kv7 channels in different tissue types could lead to targeting of a particular isoform providing therapeutic benefits with fewer adverse effects. Furthermore, the recent discovery of Epac activation of Kv7.4/Kv7.5 channels in vascular smooth muscle adds more complexity to this pathway. Determining whether this cAMP/Epac pathway plays a role in the activation of other Kv7 channels will be necessary to fully understand the physiological impact of cAMP signaling through Kv7 channels. Finally, the importance of the KCNE subunits in the cAMP-dependent regulation of Kv7 channels has often been overlooked but is likely to have a substantial role. By better defining the cAMP regulation of Kv7 channels in different tissues, it may become possible to exploit subtle tissue-specific differences in the activation pathways in order to generate novel therapeutics.
Author Contributions
All authors contributed to the drafting, revision and final approval of the manuscript.
Funding
TJ received funding from the Lundbeck Foundation (R307-2018-3674). JH received funding from the European Union’s Horizon 2020 Research and Innovation Program under the Marie Skłodowska-Curie grant agreement No. 801199.
Conflict of Interest
The authors declare that the research was conducted in the absence of any commercial or financial relationships that could be construed as a potential conflict of interest.
References
Abbott, G. W. (2016). KCNE4 and KCNE5: K(+) channel regulation and cardiac arrhythmogenesis. Gene 593, 249–260. doi: 10.1016/j.gene.2016.07.069
Abbott, G. W., and Goldstein, S. A. (1998). A superfamily of small potassium channel subunits: form and function of the MinK-related peptides (MiRPs). Q. Rev. Biophys. 31, 357–398. doi: 10.1017/s0033583599003467
Abbott, G. W., and Jepps, T. A. (2016). Kcne4 deletion sex-dependently alters vascular reactivity. J. Vasc. Res. 53, 138–148. doi: 10.1159/000449060
Adduci, A., Martire, M., Taglialatela, M., Arena, V., Rizzo, G., Coco, C., et al. (2013). Expression and motor functional roles of voltage-dependent type 7 K+ channels in the human taenia coli. Eur. J. Pharmacol. 721, 12–20. doi: 10.1016/j.ejphar.2013.09.061
Afeli, S. A. Y., Malysz, J., and Petkov, G. V. (2013). Molecular expression and pharmacological evidence for a functional role of Kv7 channel subtypes in guinea pig urinary bladder smooth muscle. PLoS One 8:e75875. doi: 10.1371/journal.pone.0075875
Andersen, M. N., Hefting, L. L., Steffensen, A. B., Schmitt, N., Olesen, S.-P., Olsen, J. V., et al. (2015). Protein kinase A stimulates Kv7.1 surface expression by regulating Nedd4-2-dependent endocytic trafficking. Am. J. Physiol. Cell Physiol. 309, C693–C706.
Anderson, U. A., Carson, C., Johnston, L., Joshi, S., Gurney, A. M., and McCloskey, K. D. (2013). Functional expression of KCNQ (Kv7) channels in guinea pig bladder smooth muscle and their contribution to spontaneous activity. Br. J. Pharmacol. 169, 1290–1304. doi: 10.1111/bph.12210
Bacallao, K., and Monje, P. V. (2015). Requirement of cAMP signaling for Schwann cell differentiation restricts the onset of myelination. PLoS One 10:e0116948. doi: 10.1371/journal.pone.0116948
Baillie, G. S. (2009). Compartmentalized signalling: spatial regulation of cAMP by the action of compartmentalized phosphodiesterases. FEBS J. 276, 1790–1799. doi: 10.1111/j.1742-4658.2009.06926.x
Bajwa, P. J., Alioua, A., Lee, J. W., Straus, D. S., Toro, L., and Lytle, C. (2007). Fenofibrate inhibits intestinal Cl- secretion by blocking basolateral KCNQ1 K+ channels. Am. J. Physiol. Liver Physiol. 293, G1288–G1299.
Bal, M., Zhang, J., Zaika, O., Hernandez, C. C., and Shapiro, M. S. (2008). Homomeric and heteromeric assembly of KCNQ (Kv7) K+ channels assayed by total internal reflection fluorescence/fluorescence resonance energy transfer and patch clamp analysis. J. Biol. Chem. 283, 30668–30676. doi: 10.1074/jbc.m805216200
Barhanin, J., Lesage, F., Guillemare, E., Fink, M., Lazdunski, M., and Romey, G. (1996). KvLQT1 and IsK (minK) proteins associate to form the IKS cardiac potassium current. Nature 384, 78–80. doi: 10.1038/384078a0
Barrese, V., Stott, J. B., and Greenwood, I. A. (2018). KCNQ-encoded potassium channels as therapeutic targets. Annu. Rev. Pharmacol. Toxicol. 58, 625–648. doi: 10.1146/annurev-pharmtox-010617-052912
Barros, F., Dominguez, P., and de la Peña, P. (2012). Cytoplasmic domains and voltage-dependent potassium channel gating. Front. Pharmacol. 3:49. doi: 10.3389/fphar.2012.00049
Bauman, A. L., Soughayer, J., Nguyen, B. T., Willoughby, D., Carnegie, G. K., Wong, W., et al. (2006). Dynamic regulation of cAMP synthesis through anchored PKA-adenylyl cyclase V/VI complexes. Mol. Cell 23, 925–931. doi: 10.1016/j.molcel.2006.07.025
Bechtel, P. J., Beavo, J. A., and Krebs, E. G. (1977). Purification and characterization of catalytic subunit of skeletal muscle adenosine 3’:5’ monophosphate dependent protein kinase. J. Biol. Chem. 252, 2691–2697.
Bennett, P. B., and Begenisch, T. B. (1987). Catecholamines modulate the delayed rectifying potassium current (IK) in guinea pig ventricular myocytes. Pflugers Arch. 410, 217–219. doi: 10.1007/bf00581919
Biel, M., and Michalakis, S. (2007). Function and dysfunction of CNG channels: insights from channelopathies and mouse models. Mol. Neurobiol. 35, 266–277. doi: 10.1007/s12035-007-0025-y
Biel, M., and Michalakis, S. (2009). Cyclic Nucleotide-Gated Channels. In CGMP: Generators, Effectors and Therapeutic Implications. Berlin: Springer, 111–136.
Biervert, C., Schroeder, B. C., Kubisch, C., Berkovic, S. F., Propping, P., Jentsch, T. J., et al. (1998). A potassium channel mutation in neonatal human epilepsy. Science 279, 403–406. doi: 10.1126/science.279.5349.403
Borgatti, R., Zucca, C., Cavallini, A., Ferrario, M., Panzeri, C., Castaldo, P., et al. (2004). A novel mutation in KCNQ2-associated with BFNC, drug resistant epilepsy, and mental retardation. Neurology 63, 57–65. doi: 10.1212/01.wnl.0000132979.08394.6d
Bos, J. L. (2006). Epac proteins: multi-purpose cAMP targets. Trends Biochem. Sci. 31, 680–686. doi: 10.1016/j.tibs.2006.10.002
Braun, T., and Dods, R. F. (1975). Development of a Mn-2+-sensitive, ‘soluble’ adenylate cyclase in rat testis. Proc. Natl. Acad. Sci. U.S.A. 72, 1097–1101. doi: 10.1073/pnas.72.3.1097
Braun, T., Frank, H., Dods, R., and Sepsenwol, S. (1977). Mn2+-sensitive, soluble adenylate cyclase in rat testis. Differentiation from other testicular nucleotide cyclases. Biochim. Biophys. Acta 481, 227–235. doi: 10.1016/0005-2744(77)90155-3
Brown, D. A., and Adams, P. R. (1980). Muscarinic suppression of a novel voltage-sensitive K+ current in a vertebrate neurone [18]. Nature 283, 673–676. doi: 10.1038/283673a0
Brown, D. A., and Selyanko, A. A. (1985). Membrane currents underlying the cholinergic slow excitatory post-synaptic potential in the rat sympathetic ganglion. J. Physiol. 365, 365–387. doi: 10.1113/jphysiol.1985.sp015777
Brueggemann, L. I., Cribbs, L. L., and Byron, K. L. (2020). Structural determinants of Kv7.5 potassium channels that confer changes in phosphatidylinositol 4,5-bisphosphate (PIP2) affinity and signaling sensitivities in smooth muscle cells. Mol. Pharmacol. 97, 145–158. doi: 10.1124/mol.119.117192
Brueggemann, L. I., Cribbs, L. L., Schwartz, J., Wang, M., Kouta, A., and Byron, K. L. (2018). Mechanisms of PKA-dependent potentiation of Kv7.5 channel activity in human airway smooth muscle cells. Int. J. Mol. Sci. 19:2223. doi: 10.3390/ijms19082223
Brueggemann, L. I., Haick, J. M., Neuburg, S., Tate, S., Randhawa, D., Cribbs, L. L., et al. (2014a). KCNQ (Kv7) potassium channel activators as bronchodilators: combination with a β2-adrenergic agonist enhances relaxation of rat airways. Am. J. Physiol. Lung Cell. Mol. Physiol. 306, L476–L486.
Brueggemann, L. I., Kakad, P. P., Love, R. B., Solway, J., Dowell, M. L., Cribbs, L. L., et al. (2012). Kv7 potassium channels in airway smooth muscle cells: signal transduction intermediates and pharmacological targets for bronchodilator therapy. Am. J. Physiol. Lung Cell. Mol. Physiol. 302, L120–L132.
Brueggemann, L. I., Mackie, A. R., Cribbs, L. L., Freda, J., Tripathi, A., Majetschak, M., et al. (2014b). Differential protein kinase C-dependent modulation of Kv7.4 and Kv7.5 subunits of vascular Kv7 channels. J. Biol. Chem. 289, 2099–2111. doi: 10.1074/jbc.m113.527820
Brueggemann, L. I., Mackie, A. R., Martin, J. L., Cribbs, L. L., and Byron, K. L. (2011). Diclofenac distinguishes among homomeric and heteromeric potassium channels composed of KCNQ4 and KCNQ5 subunits. Mol. Pharmacol. 79, 10–23. doi: 10.1124/mol.110.067496
Burns, L. L., Canaves, J. M., Pennypacker, J. K., Blumenthal, D. K., and Taylor, S. S. (2003). Isoform specific differences in binding of a dual-specificity A-kinase anchoring protein to type I and type II regulatory subunits of PKA. Biochemistry 42, 5754–5763. doi: 10.1021/bi0265729
Carr, D. W., Stofko-Hahn, R. E., Fraser, I. D. C., Bishop, S. M., Acott, T. S., Brennan, R. G., et al. (1991). Interaction of the regulatory subunit (RII) of cAMP-dependent protein kinase with RII-anchoring proteins occurs through an amphipathic helix binding motif. J. Biol. Chem. 266, 14188–14192.
Castaldo, P., Giudice, E. M., del Coppola, G., Pascotto, A., Annunziato, L., and Taglialatela, M. (2002). Benign familial neonatal convulsions caused by altered gating of KCNQ2/KCNQ3 potassium channels. J. Neurosci. 22:RC199.
Cazzola, M., Page, C. P., Rogliani, P., and Matera, M. G. (2013). β2-agonist therapy in lung disease. Am. J. Respir. Crit. Care Med. 187, 690–696. doi: 10.1164/rccm.201209-1739pp
Chadha, P. S., Jepps, T. A., Carr, G., Stott, J. B., Zhu, H.-L., Cole, W. C., et al. (2014). Contribution of Kv7.4/Kv7.5 heteromers to intrinsic and calcitonin gene-related peptide-induced cerebral reactivity. Arterioscler. Thromb. Vasc. Biol. 34, 887–893. doi: 10.1161/atvbaha.114.303405
Chadha, P. S., Zunke, F., Zhu, H.-L., Davis, A. J., Jepps, T. A., Olesen, S. P., et al. (2012). Reduced KCNQ4-encoded voltage-dependent potassium channel activity underlies impaired β-adrenoceptor-mediated relaxation of renal arteries in hypertension. Hypertension 59, 877–884. doi: 10.1161/hypertensionaha.111.187427
Charlier, C., Singh, N. A., Ryan, S. G., Lewis, T. B., Reus, B. E., Leach, R. J., et al. (1998). A pore mutation in a novel KQT-like potassium channel gene in an idiopathic epilepsy family. Nat. Genet. 18, 53–55. doi: 10.1038/ng0198-53
Chen, L., and Kass, R. S. (2006). Dual roles of the A kinase-anchoring protein Yotiao in the modulation of a cardiac potassium channel: a passive adaptor versus an active regulator. Eur. J. Cell Biol. 85, 623–626. doi: 10.1016/j.ejcb.2006.03.002
Chen, L., Kurokawa, J., and Kass, R. S. (2005). Phosphorylation of the A-kinase-anchoring protein Yotiao contributes to protein kinase A regulation of a heart potassium channel. J. Biol. Chem. 280, 31347–31352. doi: 10.1074/jbc.m505191200
Chen, L., Marquardt, M. L., Tester, D. J., Sampson, K. J., Ackerman, M. J., and Kass, R. S. (2007). Mutation of an A-kinase-anchoring protein causes long-QT syndrome. Proc. Natl. Acad. Sci. U.S.A. 104, 20990–20995. doi: 10.1073/pnas.0710527105
Cheng, X., Ji, Z., Tsalkova, T., and Mei, F. (2008). Epac and PKA: a tale of two intracellular cAMP receptors. Acta Biochim. Biophys. Sin. 40, 651–662. doi: 10.1111/j.1745-7270.2008.00438.x
Cooper, E. C., Aldape, K. D., Abosch, A., Barbaro, N. M., Berger, M. S., Peacock, W. S., et al. (2000). Colocalization and coassembly of two human brain M-type potassium channel subunits that are mutated in epilepsy. Proc. Natl. Acad. Sci. U.S.A. 97, 4914–4919. doi: 10.1073/pnas.090092797
Corbin, J. D., Sugden, P. H., Lincoln, T. M., and Keely, S. L. (1977). Compartmentalization of adenosine 3’:5’ monophosphate and adenosine 3’:5’ monophosphate dependent protein kinase in heart tissue. J. Biol. Chem. 252, 3854–3861.
Corbin, J. D., Sugden, P. H., West, L., Flockhart, D. A., Lincoln, T. M., and McCarthy, D. (1978). Studies on the properties and mode of action of the purified regulatory subunit of bovine heart adenosine 3’:5’-monophosphate-dependent protein kinase. J. Biol. Chem. 253, 3997–4003.
Cowley, E. A., and Linsdell, P. (2002). Characterizatin of basolateral K+ channels underlying anion secretion in the human airway cell line Calu-3. J. Physiol. 538, 747–757. doi: 10.1113/jphysiol.2001.013300
Cruzblanca, H., Koh, D. S., and Hille, B. (1998). Bradykinin inhibits M current via phospholipase C and Ca2+ release from IP3-sensitive Ca2+ stores in rat sympathetic neurons. Proc. Natl. Acad. Sci. U.S.A. 95, 7151–7156. doi: 10.1073/pnas.95.12.7151
Dell’Acqua, M. L., Smith, K. E., Gorski, J. A., Horne, E. A., Gibson, E. S., and Gomez, L. L. (2006). Regulation of neuronal PKA signaling through AKAP targeting dynamics. Eur. J. Cell Biol. 85, 627–633. doi: 10.1016/j.ejcb.2006.01.010
Dema, A., Perets, E., Schulz, M. S., Deák, V. A., and Klussmann, E. (2015). Pharmacological targeting of AKAP-directed compartmentalized cAMP signalling. Cell. Signal. 27, 2474–2487. doi: 10.1016/j.cellsig.2015.09.008
Devor, D. G., Singh, A. K., Gerlach, A. C., Frizzell, R. A., and Bridges, R. J. (1997). Inhibition of intestinal Cl- secretion by clotrimazole: Direct effect on basolateral membrane K+ channels. Am. J. Physiol 273, C531–C540.
Diener, M., Hug, F., Strabel, D., and Scharrer, E. (1996). Cyclic AMP-dependent regulation of K+ transport in the rat distal colon. Br. J. Pharmacol. 118, 1477–1487. doi: 10.1111/j.1476-5381.1996.tb15563.x
Difrancesco, D. (1986). Characterization of single pacemaker channels in cardiac sino-atrial node cells. Nature 324, 470–473. doi: 10.1038/324470a0
DiFrancesco, D. (2020). A brief history of pacemaking. Front. Physiol. 10:1599. doi: 10.3389/fphys.2019.01599
DiFrancesco, D., and Tortora, P. (1991). Direct activation of cardiac pacemaker channels by intracellular cyclic AMP. Nature 351, 145–147. doi: 10.1038/351145a0
Doyle, D. A., Morais Cabral, J., Pfuetzner, R. A., Kuo, A., Gulbis, J. M., Cohen, S. L., et al. (1998). The structure of the potassium channel: molecular basis of K+ conduction and selectivity. Science 280, 69–77. doi: 10.1126/science.280.5360.69
Dumont, J. E., Jauniaux, J. C., and Roger, P. P. (1989). The cyclic AMP-mediated stimulation of cell proliferation. Trends Biochem. Sci. 14, 67–71. doi: 10.1016/0968-0004(89)90046-7
Evseev, A. I., Semenov, I., Archer, C. R., Medina, J. L., Dube, P. H., Shapiro, M. S., et al. (2013). Functional effects of KCNQ K(+) channels in airway smooth muscle. Front. Physiol. 4:277. doi: 10.3389/fphys.2013.00277
Fink, M. A., Zakhary, D. R., Mackey, J. A., Desnoyer, R. W., Apperson-Hansen, C., Damron, D. S., et al. (2001). AKAP-mediated targeting of protein kinase A regulates contractility in cardiac myocytes. Circ. Res. 88, 291–297. doi: 10.1161/01.res.88.3.291
Fodstad, H., Swan, H., Laitinen, P., Piippo, K., Paavonen, K., Viitasalo, M., et al. (2004). Four potassium channel mutations account for 73% of the genetic spectrum underlying long−QT syndrome (LQTS) and provide evidence for a strong founder effect in Finland. Ann. Med. 36, 53–63. doi: 10.1080/17431380410032689
Forte, L. R., Bylund, D. B., and Zahler, W. L. (1983). Forskolin does not activate sperm adenylate cyclase. Mol. Pharmacol. 24, 42–47.
Fujita, T., Umemura, M., Yokoyama, U., Okumura, S., and Ishikawa, Y. (2017). The role of Epac in the heart. Cell. Mol. Life Sci. 74, 591–606. doi: 10.1007/s00018-016-2336-5
Gamper, N., Stockand, J. D., and Shapiro, M. S. (2003). Subunit-specific modulation of KCNQ potassium channels by Src tyrosine kinase. J. Neurosci. 23, 84–95. doi: 10.1523/jneurosci.23-01-00084.2003
Glantz, S. B., Amat, J. A., and Rubin, C. S. (1992). cAMP signaling in neurons: patterns of neuronal expression and intracellular localization for a novel protein, AKAP 150, that anchors the regulatory subunit of cAMP-dependent protein kinase IIβ. Mol. Biol. Cell 3, 1215–1228. doi: 10.1091/mbc.3.11.1215
Gold, M. G., Lygren, B., Dokurno, P., Hoshi, N., McConnachie, G., Taskén, K., et al. (2006). Molecular basis of AKAP specificity for PKA regulatory subunits. Mol. Cell 24, 383–395. doi: 10.1016/j.molcel.2006.09.006
Grahammer, F., Herling, A. W., Lang, H. J., Schmitt-Gräff, A., Wittekindt, O. H., Nitschke, R., et al. (2001a). The cardiac K+ channel KCNQ1 is essential for gastric acid secretion. Gastroenterology 120, 1363–1371. doi: 10.1053/gast.2001.24053
Grahammer, F., Warth, R., Barhanin, J., Bleich, M., and Hug, M. J. (2001b). The small conductance K+ channel, KCNQ1. Expression, function, and subunit composition in murine trachea. J. Biol. Chem. 276, 42268–42275. doi: 10.1074/jbc.m105014200
Greenwood, I. A., and Stott, J. B. (2020). The Gβ1 and Gβ3 subunits differentially regulate rat vascular Kv7 channels. Front. Physiol. 10:1573. doi: 10.3389/fphys.2019.01573
Gutman, G. A., Chandy, K. G., Grissmer, S., Lazdunski, M., McKinnon, D., Pardo, L. A., et al. (2005). International union of pharmacology. LIII. Nomenclature and molecular relationships of voltage-gated potassium channels. Pharmacol. Rev. 57, 473–508. doi: 10.1124/pr.57.4.10
Haick, J. M., and Byron, K. L. (2016). Novel treatment strategies for smooth muscle disorders: targeting Kv7 potassium channels. Pharmacol. Ther. 165, 14–25. doi: 10.1016/j.pharmthera.2016.05.002
Haitin, Y., and Attali, B. (2008). The C-terminus of Kv7 channels: a multifunctional module. J. Physiol. 586, 1803–1810. doi: 10.1113/jphysiol.2007.149187
Halls, M. L., and Cooper, D. M. F. (2017). Adenylyl cyclase signalling complexes – pharmacological challenges and opportunities. Pharmacol. Ther. 172, 171–180. doi: 10.1016/j.pharmthera.2017.01.001
Hanoune, J., and Defer, N. (2001). Regulation and role of adenylyl cyclase isoforms. Annu. Rev. Pharmacol. Toxicol. 41, 145–174.
Hansen, H. H., Waroux, O., Seutin, V., Jentsch, T. J., Aznar, S., and Mikkelsen, J. D. (2008). Kv7 channels: Interaction with dopaminergic and serotonergic neurotransmission in the CNS. J. Physiol. 586, 1823–1832. doi: 10.1113/jphysiol.2007.149450
Heginbotham, L., Abramson, T., and MacKinnon, R. (1992). A functional connection between the pores of distantly related ion channels as revealed by mutant K+ channels. Science 258, 1152–1155. doi: 10.1126/science.1279807
Higashida, H., Hoshi, N., Zhang, J.-S., Yokoyama, S., Hashii, M., Jin, D., et al. (2005). Protein kinase C bound with A-kinase anchoring protein is involved in muscarinic receptor-activated modulation of M-type KCNQ potassium channels. Neurosci. Res. 51, 231–234. doi: 10.1016/j.neures.2004.11.009
Hoshi, N., Langeberg, L. K., and Scott, J. D. (2005). Distinct enzyme combinations in AKAP signalling complexes permit functional diversity. Nat. Cell Biol. 7, 1066–1073. doi: 10.1038/ncb1315
Hoshi, N., Zhang, J.-S., Omaki, M., Takeuchi, T., Yokoyama, S., Wanaverbecq, N., et al. (2003). AKAP150 signaling complex promotes suppression of the M-current by muscarinic agonists. Nat. Neurosci. 6, 564–571. doi: 10.1038/nn1062
Huang, L. J. S., Durick, K., Weiner, J. A., Chun, J., and Taylor, S. S. (1997). Identification of a novel protein kinase A anchoring protein that binds both type I and type II regulatory subunits. J. Biol. Chem. 272, 8057–8064. doi: 10.1074/jbc.272.12.8057
Iannotti, F. A., Panza, E., Barrese, V., Viggiano, D., Soldovieri, M. V., and Taglialatela, M. (2010). Expression, localization, and pharmacological role of Kv7 potassium channels in skeletal muscle proliferation, differentiation, and survival after myotoxic insults. J. Pharmacol. Exp. Ther. 332, 811–820. doi: 10.1124/jpet.109.162800
Ipavec, V., Martire, M., Barrese, V., Taglialatela, M., and Currò, D. (2011). KV7 channels regulate muscle tone and nonadrenergic noncholinergic relaxation of the rat gastric fundus. Pharmacol. Res. 64, 397–409. doi: 10.1016/j.phrs.2011.06.016
Jenke, M., Sánchez, A., Monje, F., Stühmer, W., Weseloh, R. M., and Pardo, L. A. (2003). C-terminal domains implicated in the functional surface expression of potassium channels. EMBO J. 22, 395–403. doi: 10.1093/emboj/cdg035
Jentsch, T. J. (2000). Neuronal KCNQ potassium channels:physislogy and role in disease. Nat. Rev. Neurosci. 1, 21–30. doi: 10.1038/35036198
Jepps, T. A. (2017). Unravelling the complexities of vascular smooth muscle ion channels: fine tuning of activity by ancillary subunits. Pharmacol. Ther. 178, 57–66. doi: 10.1016/j.pharmthera.2017.03.010
Jepps, T. A., Carr, G., Lundegaard, P. R., Olesen, S.-P., and Greenwood, I. A. (2015). Fundamental role for the KCNE4 ancillary subunit in Kv7.4 regulation of arterial tone. J. Physiol. 593, 5325–5340. doi: 10.1113/jp271286
Jepps, T. A., Chadha, P. S., Davis, A. J., Harhun, M. I., Cockerill, G. W., Olesen, S. P., et al. (2011). Downregulation of Kv7.4 channel activity in primary and secondary hypertension. Circulation 124, 602–611.
Jepps, T. A., Greenwood, I. A., Moffatt, J. D., Sanders, K. M., and Ohya, S. (2009). Molecular and functional characterization of Kv7 K+ channel in murine gastrointestinal smooth muscles. Am. J. Physiol. Gastrointest. Liver Physiol. 297, G107–G115.
Jepps, T. A., Olesen, S. P., Greenwood, I. A., and Dalsgaard, T. (2016). Molecular and functional characterization of Kv7 channels in penile arteries and corpus cavernosum of healthy and metabolic syndrome rats. Br. J. Pharmacol. 173, 1478–1490. doi: 10.1111/bph.13444
Jespersen, T., Membrez, M., Nicolas, C., Pitard, B., Staub, O., Olesen, S., et al. (2007). The KCNQ1 potassium channel is down-regulated by ubiquitylating enzymes of the Nedd4/Nedd4-like family. Cardiovasc. Res. 74, 64–74. doi: 10.1016/j.cardiores.2007.01.008
Kanda, V. A., and Abbott, G. W. (2012). KCNE regulation of K(+) channel trafficking – a sisyphean task? Front. Physiol. 3:231. doi: 10.3389/fphys.2012.00231
Kang, G., Chepurny, O. G., Malester, B., Rindler, M. J., Rehmann, H., Bos, J. L., et al. (2006). cAMP sensor Epac as a determinant of ATP-sensitive potassium channel activity in human pancreatic β cells and rat INS-1 cells. J. Physiol. 573, 595–609. doi: 10.1113/jphysiol.2006.107391
Kass, R. S., Kurokawa, J., Marx, S. O., and Marks, A. R. (2003). Leucine/isoleucine zipper coordination of ion channel macromolecular signaling complexes in the heart. Roles in inherited arrhythmias. Trends Cardiovasc. Med. 13, 52–56. doi: 10.1016/s1050-1738(02)00211-6
Kaupp, U. B., and Seifert, R. (2002). Cyclic nucleotide-gated ion channels. Physiol. Rev. 82, 769–824.
Kawasaki, H., Springett, G. M., Mochizuki, N., Toki, S., Nakaya, M., Matsuda, M., et al. (1998). A family of cAMP-binding proteins that directly activate Rap1. Science 282, 2275–2279. doi: 10.1126/science.282.5397.2275
Khanamiri, S., Soltysinska, E., Jepps, T. A., Bentzen, B. H., Chadha, P. S., Schmitt, N., et al. (2013). Contribution of Kv7 channels to basal coronary flow and active response to ischemia. Hypertens 62, 1090–1097. doi: 10.1161/hypertensionaha.113.01244
Kharkovets, T., Hardelin, J. P., Safieddine, S., Schweizer, M., El-Amraoui, A., Petit, C., et al. (2000). KCNQ4, a K+ channel mutated in a form of dominant deafness, is expressed in the inner ear and the central auditory pathway. Proc. Natl. Acad. Sci. U.S.A. 97, 4333–4338. doi: 10.1073/pnas.97.8.4333
Kim, J. K., Yoo, H. Y., Kim, S. J., Hwang, Y.-S., Han, J., Kim, J. A., et al. (2007). Effects of sevoflurane on the cAMP-induced short-circuit current in mouse tracheal epithelium and recombinant Cl- (CFTR) and K+ (KCNQ1) channels†. Br. J. Anaesth. 99, 245–251. doi: 10.1093/bja/aem123
Kim, S. J., and Greger, R. (1999). Voltage-dependent, slowly activating K+ current (I(Ks)) and its augmentation by carbachol in rat pancreatic acini. Pflugers Arch. Eur. J. Physiol. 438, 604–611. doi: 10.1007/s004240051083
Kinderman, F. S., Kim, C., Daake, S., von Ma, Y., Pham, B. Q., Spraggon, G., et al. (2006). A dynamic mechanism for AKAP binding to RII isoforms of cAMP-dependent protein kinase. Mol. Cell 24, 397–408. doi: 10.1016/j.molcel.2006.09.015
Köttgen, M., Hoefer, A., Kim, S. J., Beschorner, U., Schreiber, R., Hug, M. J., et al. (1999). Carbachol activates a K+ channel of very small conductance in the basolateral membrane of rat pancreatic acinar cells. Pflugers Arch. 438, 597–603. doi: 10.1007/s004240051082
Krebs, E. G., and Beavo, J. A. (1979). Phosphorylation-dephosphorylation of enzymes. Annu. Rev. Biochem. 48, 923–959. doi: 10.1146/annurev.bi.48.070179.004423
Kubisch, C., Schroeder, B. C., Friedrich, T., Lütjohann, B., El-Amraoui, A., Marlin, S., et al. (1999). KCNQ4, a novel potassium channel expressed in sensory outer hair cells, is mutated in dominant deafness. Cell 96, 437–446. doi: 10.1016/s0092-8674(00)80556-5
Kurokawa, J., Motoike, H. K., Rao, J., and Kass, R. S. (2004). Regulatory actions of the A-kinase anchoring protein Yotiao on a heart potassium channel downstream of PKA phosphorylation. Proc. Natl. Acad. Sci. U.S.A. 101, 16374–16378. doi: 10.1073/pnas.0405583101
Lerche, C., Scherer, C. R., Seebohm, G., Derst, C., Wei, A. D., Busch, A. E., et al. (2000). Molecular cloning and functional expression of KCNQ5, a potassium channel subunit that may contribute to neuronal M-current diversity. J. Biol. Chem. 275, 22395–22400. doi: 10.1074/jbc.m002378200
Lester, L. B., Langeberg, L. K., and Scott, J. D. (1997). Anchoring of protein kinase A facilitates hormone-mediated insulin secretion. Proc. Natl. Acad. Sci. U.S.A. 94, 14942–14947. doi: 10.1073/pnas.94.26.14942
Li, Y., Chen, L., Kass, R. S., and Dessauer, C. W. (2012). The A-kinase anchoring protein yotiao facilitates complex formation between adenylyl cyclase type 9 and the IKs potassium channel in heart. J. Biol. Chem. 287, 29815–29824. doi: 10.1074/jbc.m112.380568
Li, Y., Langlais, P., Gamper, N., Liu, F., and Shapiro, M. S. (2004). Dual phosphorylations underlie modulation of unitary KCNQ K+ channels by Src tyrosine kinase. J. Biol. Chem. 279, 45399–45407. doi: 10.1074/jbc.m408410200
Lindman, J., Khammy, M. M., Lundegaard, P. R., Aalkjær, C., and Jepps, T. A. (2018). Microtubule regulation of Kv7 channels orchestrates cAMP-mediated vasorelaxations in rat arterial smooth muscle. Hypertension 71, 336–345. doi: 10.1161/hypertensionaha.117.10152
Liu, L., Wang, F., Lu, H., Ren, X., and Zou, J. (2014). Chromanol 293B, an inhibitor of KCNQ1 channels, enhances glucose-stimulated insulin secretion and increases glucagon-like peptide-1 level in mice. Islets 6:e962386. doi: 10.4161/19382014.2014.962386
Lohrmann, E., Burhoff, I., Nitschke, R. B., Lang, H. J., Mania, D., Englert, H. C., et al. (1995). A new class of inhibitors of cAMP-mediated Cl- secretion in rabbit colon, acting by the reduction of cAMP-activated K+ conductance. Pflügers Arch. Eur. J. Physiol. 429, 517–530. doi: 10.1007/bf00704157
Ludwig, A., Zong, X., Jeglitsch, M., Hofmann, F., and Biel, M. (1998). A family of hyperpolarization-activated mammalian cation channels. Nature 393, 587–591. doi: 10.1038/31255
Lundby, A., Andersen, M. N., Steffensen, A. B., Horn, H., Kelstrup, C. D., Francavilla, C., et al. (2013). In vivo phosphoproteomics analysis reveals the cardiac targets of beta-adrenergic receptor signaling. Sci. Signal. 6:rs11. doi: 10.1126/scisignal.2003506
MacVinish, L. J., Guo, Y., Dixon, A. K., Murrell-Lagnado, R. D., and Cuthbert, A. W. (2001). Xe991 reveals differences in K(+) channels regulating chloride secretion in murine airway and colonic epithelium. Mol. Pharmacol. 60, 753–760.
Malaisse, W. J., and Malaisse-Lagae, F. (1984). The role of cyclic AMP in insulin release. Experientia 40, 1068–1075. doi: 10.1007/bf01971453
Mall, M., Wissner, A., Schreiber, R., Kuehr, J., Seydewitz, H. H., Brandis, M., et al. (2000). Role of K(v)LQT1 in cyclic adenosine monophosphate-mediated Cl- secretion in human airway epithelia. Am. J. Respir. Cell Mol. Biol. 23, 283–289. doi: 10.1165/ajrcmb.23.3.4060
Mani, B. K., Robakowski, C., Brueggemann, L. I., Cribbs, L. L., Tripathi, A., Majetschak, M., et al. (2016). Kv7.5 potassium channel subunits are the primary targets for PKA-dependent enhancement of vascular smooth muscle Kv7 currents. Mol. Pharmacol. 89, 323–334. doi: 10.1124/mol.115.101758
Manning, J. A., and Kumar, S. (2018). Physiological functions of Nedd4-2: lessons from knockout mouse models. Trends Biochem. Sci. 43, 635–647. doi: 10.1016/j.tibs.2018.06.004
Marx, S. O., Kurokawa, J., Reiken, S., Motoike, H., D’Armiento, J., Marks, A. R., et al. (2002). Requirement of a macromolecular signaling complex for beta adrenergic receptor modulation of the KCNQ1-KCNE1 potassium channel. Science 295, 496–499. doi: 10.1126/science.1066843
Mayr, B., and Montminy, M. (2001). Transcriptional regulation by the phosphorylation-dependent factor CREB. Nat. Rev. Mol. Cell Biol. 2, 599–609. doi: 10.1038/35085068
McCallum, L. A., Greenwood, I. A., and Tribe, R. M. (2009). Expression and function of Kv7 channels in murine myometrium throughout oestrous cycle. Pflugers Arch. Eur. J. Physiol. 457, 1111–1120. doi: 10.1007/s00424-008-0567-5
McCrossan, Z. A., and Abbott, G. W. (2004). The MinK-related peptides. Neuropharmacology 47, 787–821. doi: 10.1016/j.neuropharm.2004.06.018
Min Lee, S., Baik, J., Nguyen, D., Nguyen, V., Liu, S., Hu, Z., et al. (2017). Kcne2 deletion impairs insulin secretion and causes type 2 diabetes mellitus. FASEB J. 31, 2674–2685. doi: 10.1096/fj.201601347
Moita, M. A. P., Lamprecht, R., Nader, K., and LeDoux, J. E. (2002). A-kinase anchoring proteins in amygdala are involved in auditory fear memory. Nat. Neurosci. 5, 837–838. doi: 10.1038/nn901
Moleschi, K., and Melacini, G. (2014). Signaling at crossroads: the dialogue between PDEs and PKA is spoken in multiple languages. Biophys. J. 107, 1259–1260. doi: 10.1016/j.bpj.2014.07.051
Mona, A., Xiao-Yan, Q., Ling, X., Balazs, O., Artavazd, T., Xiaobin, L., et al. (2014). Exchange protein directly activated by cAMP mediates slow delayed-rectifier current remodeling by sustained β-adrenergic activation in guinea pig hearts. Circ. Res. 114, 993–1003. doi: 10.1161/circresaha.113.302982
Moosmang, S., Biel, M., Hofmann, F., and Ludwig, A. (1999). Differential distribution of four hyperpolarization-activated cation channels in mouse brain. Biol. Chem. 380, 975–980. doi: 10.1515/bc.1999.121
Neyroud, N., Tesson, F., Denjoy, I., Leibovici, M., Donger, C., Barhanin, J., et al. (1997). A novel mutation in the potassium channel gene KVLQT1 causes the Jervell and Lange-Nielsen cardioauditory syndrome. Nat. Genet. 15, 186–189. doi: 10.1038/ng0297-186
Nicolas, C. S., Park, K.-H., Harchi, A., El Camonis, J., Kass, R. S., Escande, D., et al. (2008). IKs response to protein kinase A-dependent KCNQ1 phosphorylation requires direct interaction with microtubules. Cardiovasc. Res. 79, 427–435. doi: 10.1093/cvr/cvn085
Ohya, S., Asakura, K., Muraki, K., Watanabe, M., and Imaizumi, Y. (2002). Molecular and functional characterization of ERG, KCNQ, and KCNE subtypes in rat stomach smooth muscle. Am. J. Physiol. Gastrointest. Liver Physiol. 282, G277–G287.
Oliveras, A., Roura-Ferrer, M., Solé, L., La Cruz, A., De Prieto, A., Etxebarria, A., et al. (2014). Functional assembly of Kv7.1/Kv7.5 channels with emerging properties on vascular muscle physiology. Arterioscler. Thromb. Vasc. Biol. 34, 1522–1530.
Pierce, K. L., Premont, R. T., and Lefkowitz, R. J. (2002). Seven-transmembrane receptors. Nat. Rev. Mol. Cell Biol. 3, 639–650.
Potet, F., Scott, J. D., Mohammad-Panah, R., Escande, D., and Baró, I. (2001). AKAP proteins anchor cAMP-dependent protein kinase to KvLQT1/IsK channel complex. Am. J. Physiol. Circ. Physiol. 280, H2038–H2045.
Preston, P., Wartosch, L., Günzel, D., Fromm, M., Kongsuphol, P., Ousingsawat, J., et al. (2010). Disruption of the K+ channel beta-subunit KCNE3 reveals an important role in intestinal and tracheal Cl- transport. J. Biol. Chem. 285, 7165–7175. doi: 10.1074/jbc.m109.047829
Rall, T. W., and Sutherland, E. W. (1958). Formation of a cyclic adenine ribonucleotide by tissue particles. J. Biol. Chem. 232, 1065–1076.
Rannels, S. R., and Corbin, J. D. (1980). Studies of functional domains of the regulatory subunit from cAMP-dependent protein kinase isozyme I. J. Cyclic Nucleotide Res. 6, 201–215.
Renström, E., Eliasson, L., and Rorsman, P. (1997). Protein kinase A-dependent and -independent stimulation of exocytosis by cAMP in mouse pancreatic B-cells. J. Physiol. 502(Pt 1), 105–118. doi: 10.1111/j.1469-7793.1997.105bl.x
Ringheim, G. E., and Taylor, S. S. (1990). Dissecting the domain structure of the regulatory subunit of cAMP-dependent protein kinase I and elucidating the role of MgATP. J. Biol. Chem. 265, 4800–4808.
Robbins, J. (2001). KCNQ potassium channels: physiology, pathophysiology, and pharmacology. Pharmacol. Ther. 90, 1–19. doi: 10.1016/s0163-7258(01)00116-4
Roche, J. P., Westenbroek, R., Sorom, A. J., Hille, B., Mackie, K., and Shapiro, M. S. (2002). Antibodies and a cysteine-modifying reagent show correspondence of M current in neurons to KCNQ2 and KCNQ3 K + channels. Br. J. Pharmacol. 137, 1173–1186. doi: 10.1038/sj.bjp.0704989
Rode, F., Svalø, J., Sheykhzade, M., and Rønn, L. C. B. (2010). Functional effects of the KCNQ modulators retigabine and XE991 in the rat urinary bladder. Eur. J. Pharmacol. 638, 121–127. doi: 10.1016/j.ejphar.2010.03.050
de Rooij, J., Zwartkruis, F. J. T., Verheijen, M. H. G., Cool, R. H., Nijman, S. M. B., Wittinghofer, A., et al. (1998). Epac is a Rap1 guanine-nucleotide-exchange factor directly activated by cyclic AMP. Nature 396, 474–477. doi: 10.1038/24884
Roscioni, S. S., Elzinga, C. R. S., and Schmidt, M. (2008). Epac: effectors and biological functions. Naunyn. Schmiedebergs. Arch. Pharmacol. 377, 345–357. doi: 10.1007/s00210-007-0246-7
Roura-Ferrer, M., Solé, L., Martínez-Mármol, R., Villalonga, N., and Felipe, A. (2008). Skeletal muscle Kv7 (KCNQ) channels in myoblast differentiation and proliferation. Biochem. Biophys. Res. Commun. 369, 1094–1097. doi: 10.1016/j.bbrc.2008.02.152
Rubin, C. S. (1994). A kinase anchor proteins and the intracellular targeting of signals carried by cyclic AMP. Biochim. Biophys. Acta 1224, 467–479.
Ruehr, M. L., Russell, M. A., and Bond, M. (2004). A-kinase anchoring protein targeting of protein kinase A in the heart. J. Mol. Cell. Cardiol. 37, 653–665. doi: 10.1016/j.yjmcc.2004.04.017
Rufo, P. A., Merlin, D., Riegler, M., Ferguson-Maltzman, M. H., Dickinson, B. L., Brugnara, C., et al. (1997). The antifungal antibiotic, clotrimazole, inhibits chloride secretion by human intestinal T84 cells via blockade of distinct basolateral K+ conductances: demonstration of efficacy in the intact rabbit colon and in an in vivo mouse model of cholera. J. Clin. Invest. 100, 3111–3120. doi: 10.1172/jci119866
Sadana, R., and Dessauer, C. W. (2009). Physiological roles for G protein-regulated adenylyl cyclase isoforms: insights from knockout and overexpression studies. Neurosignals. 17, 5–22. doi: 10.1159/000166277
Salzer, I., Erdem, F. A., Chen, W.-Q., Heo, S., Koenig, X., Schicker, K. W., et al. (2017). Phosphorylation regulates the sensitivity of voltage-gated Kv7.2 channels towards phosphatidylinositol-4,5-bisphosphate. J. Physiol. 595, 759–776. doi: 10.1113/jp273274
Sands, W. A., and Palmer, T. M. (2008). Regulating gene transcription in response to cyclic AMP elevation. Cell. Signal. 20, 460–466. doi: 10.1016/j.cellsig.2007.10.005
Sanguinetti, M. C., Curran, M. E., Zou, A., Shen, J., Specter, P. S., Atkinson, D. L., et al. (1996). Coassembly of KVLQT1 and minK (IsK) proteins to form cardiac IKS potassium channel. Nature 384, 80–83. doi: 10.1038/384080a0
Santoro, B., Liu, D. T., Yao, H., Bartsch, D., Kandel, E. R., Siegelbaum, S. A., et al. (1998). Identification of a gene encoding a hyperpolarization-activated pacemaker channel of brain. Cell 93, 717–729. doi: 10.1016/s0092-8674(00)81434-8
Sarma, G. N., Kinderman, F. S., Kim, C., Daake, S., von Chen, L., Wang, B. C., et al. (2010). Structure of D-AKAP2:PKA RI complex: insights into AKAP specificity and selectivity. Structure 18, 155–166. doi: 10.1016/j.str.2009.12.012
Sassone-Corsi, P. (1995). Transcription factors responsive to cAMP. Annu. Rev. Cell Dev. Biol. 11, 355–377. doi: 10.1146/annurev.cb.11.110195.002035
Schroeder, B. C., Hechenberger, M., Weinreich, F., Kubisch, C., and Jentsch, T. J. (2000a). KCNQ5, a novel potassium channel broadly expressed in brain, mediates M-type currents. J. Biol. Chem. 275, 24089–24095. doi: 10.1074/jbc.m003245200
Schroeder, B. C., Kubisch, C., Stein, V., and Jentsch, T. J. (1998). Moderate loss of function of cyclic-AMP-modulated KCNQ2/KCNQ3 K+ channels causes epilepsy. Nature 396, 687–690. doi: 10.1038/25367
Schroeder, B. C., Waldegger, S., Fehr, S., Bleich, M., Warth, R., Greger, R., et al. (2000b). A constitutively open potassium channel formed by KCNQ1 and KCNE3. Nature 403, 196–199. doi: 10.1038/35003200
Schwake, M., Athanasiadu, D., Beimgraben, C., Blanz, J., Beck, C., Jentsch, T. J., et al. (2006). Structural determinants of M-type KCNQ (Kv7) K+ channel assembly. J. Neurosci. 26, 3757–3766. doi: 10.1523/jneurosci.5017-05.2006
Schwake, M., Jentsch, T. J., and Friedrich, T. (2003). A carboxy-terminal domain determines the subunit specificity of KCNQ K+ channel assembly. EMBO Rep. 4, 76–81. doi: 10.1038/sj.embor.embor715
Schwake, M., Pusch, M., Kharkovets, T., and Jentsch, T. J. (2000). Surface expression and single channel properties of KCNQ2/KCNQ3, M-type K+ channels involved in epilepsy. J. Biol. Chem. 275, 13343–13348. doi: 10.1074/jbc.275.18.13343
Scott, J. D., Dessauer, C. W., and Taskén, K. (2013). Creating order from chaos: cellular regulation by kinase anchoring. Annu. Rev. Pharmacol. Toxicol. 53, 187–210. doi: 10.1146/annurev-pharmtox-011112-140204
Scott, J. D., Stofko, R. E., McDonald, J. R., Comer, J. D., Vitalis, E. A., and Mangili, J. A. (1990). Type II regulatory subunit dimerization determines the subcellular localization of the cAMP-dependent protein kinase. J. Biol. Chem. 265, 21561–21566.
Seino, S., and Shibasaki, T. (2005). PKA-dependent and PKA-independent pathways for cAMP-regulated exocytosis. Physiol. Rev. 85, 1303–1342. doi: 10.1152/physrev.00001.2005
Shabb, J. B. (2001). Physiological substrates of cAMP-dependent protein kinase. Chem. Rev. 101, 2381–2411.
Shah, M. M., Mistry, M., Marsh, S. J., Brown, D. A., and Delmas, P. (2002). Molecular correlates of the M-current in cultured rat hippocampal neurons. J. Physiol. 544, 29–37. doi: 10.1113/jphysiol.2002.028571
Shariati, B., Thompson, E. L., Nicol, G. D., and Vasko, M. R. (2016). Epac activation sensitizes rat sensory neurons through activation of Ras. Mol. Cell. Neurosci. 70, 54–67. doi: 10.1016/j.mcn.2015.11.005
Simonds, W. F. (1999). G protein regulation of adenylate cyclase. Trends Pharmacol. Sci. 20, 66–73. doi: 10.1016/s0165-6147(99)01307-3
Sims, S. M., Singer, J.J., and Walsh, J. V. Jr. (1988). Antagonistic adrenergic-muscarinic regulation of M current in smooth muscle cells. Science 239, 190–193.
Singh, N. A., Charlier, C., Stauffer, D., DuPont, B. R., Leach, R. J., Melis, R., et al. (1998). A novel potassium channel gene, KCNQ2, is mutated in an inherited epilepsy of newborns. Nat. Genet. 18, 25–29. doi: 10.1038/ng0198-25
Steele, D. F., and Fedida, D. (2014). Cytoskeletal roles in cardiac ion channel expression. Biochim. Biophys. Acta Biomembr. 1838, 665–673. doi: 10.1016/j.bbamem.2013.05.001
Stott, J. B., Barrese, V., and Greenwood, I. A. (2016). Kv7 channel activation underpins EPAC-dependent relaxations of rat arteries. Arterioscler. Thromb. Vasc. Biol. 36, 2404–2411. doi: 10.1161/atvbaha.116.308517
Stott, J. B., Barrese, V., Suresh, M., Masoodi, S., and Greenwood, I. A. (2018). Investigating the role of G protein βγ in Kv7-dependent relaxations of the rat vasculature. Arterioscler. Thromb. Vasc. Biol. 38, 2091–2102. doi: 10.1161/atvbaha.118.311360
Stott, J. B., Povstyan, O. V., Carr, G., Barrese, V., and Greenwood, I. A. (2015). G-protein βγ subunits are positive regulators of Kv7.4 and native vascular Kv7 channel activity. Proc. Natl. Acad. Sci. U.S.A. 112, 6497–6502. doi: 10.1073/pnas.1418605112
Streng, T., Christoph, T., and Andersson, K. E. (2004). Urodynamic effects of the K+ channel (KCNQ) opener retigabine in freely moving, conscious rats. J. Urol. 172, 2054–2058. doi: 10.1097/01.ju.0000138155.33749.f4
Suessbrich, H., Bleich, M., Ecke, D., Rizzo, M., Waldegger, S., Lang, F., et al. (1996). Specific blockade of slowly activating I(sK) channels by chromanols - Impact on the role of I(sK) channels in epithelial. FEBS Lett. 396, 271–275. doi: 10.1016/0014-5793(96)01113-1
Sunose, H., Liu, J., and Marcus, D. C. (1997). cAMP increases K+ secretion via activation of apical IsK/KvLQT1 channels in strial marginal cells. Hear. Res. 114, 107–116. doi: 10.1016/s0378-5955(97)00152-4
Surti, T. S., Huang, L., Jan, Y. N., Jan, L. Y., and Cooper, E. C. (2005). Identification by mass spectrometry and functional characterization of two phosphorylation sites of KCNQ2/KCNQ3 channels. Proc. Natl. Acad. Sci. U.S.A. 102, 17828–17833. doi: 10.1073/pnas.0509122102
Sutherland, E. W., and Rall, T. W. (1958). Fractionation and characterization of a cyclic adenine ribonucleotide formed by tissue particles. J. Biol. Chem. 232, 1077–1091.
Svalø, J., Bille, M., Parameswaran Theepakaran, N., Sheykhzade, M., Nordling, J., and Bouchelouche, P. (2013). Bladder contractility is modulated by Kv7 channels in pig detrusor. Eur. J. Pharmacol. 715, 312–320. doi: 10.1016/j.ejphar.2013.05.005
Svalø, J., Hansen, H. H., Rønn, L. C. B., Sheykhzade, M., Munro, G., and Rode, F. (2012). Kv7 positive modulators reduce detrusor overactivity and increase bladder capacity in rats. Basic Clin. Pharmacol. Toxicol. 110, 145–153. doi: 10.1111/j.1742-7843.2011.00765.x
Taussig, R., and Gilman, A. G. (1995). Mammalian membrane-bound adenylyl cyclases. J. Biol. Chem. 270, 1–4. doi: 10.1074/jbc.270.1.1
Taylor, S. S., Ilouz, R., Zhang, P., and Kornev, A. P. (2012). Assembly of allosteric macromolecular switches: lessons from PKA. Nat. Rev. Mol. Cell Biol. 13, 646–658. doi: 10.1038/nrm3432
Taylor, S. S., Zhang, P., Steichen, J. M., Keshwani, M. M., and Kornev, A. P. (2013). PKA: lessons learned after twenty years. Biochim. Biophys. Acta 1834, 1271–1278. doi: 10.1016/j.bbapap.2013.03.007
Terrenoire, C., Clancy, C. E., Cormier, J. W., Sampson, K. J., and Kass, R. S. (2005). Autonomic control of cardiac action potentials. Circ. Res. 96, e25–e34.
Terrenoire, C., Houslay, M. D., Baillie, G. S., and Kass, R. S. (2009). The cardiac IKs potassium channel macromolecular complex includes the phosphodiesterase PDE4D3. J. Biol. Chem. 284, 9140–9146. doi: 10.1074/jbc.m805366200
Testai, L., Barrese, V., Soldovieri, M. V., Ambrosino, P., Martelli, A., Vinciguerra, I., et al. (2016). Expression and function of Kv7.4 channels in rat cardiac mitochondria: possible targets for cardioprotection. Cardiovasc. Res. 110, 40–50. doi: 10.1093/cvr/cvv281
Torekov, S. S., Iepsen, E., Christiansen, M., Linneberg, A., Pedersen, O., Holst, J. J., et al. (2014). KCNQ1 long QT syndrome patients have hyperinsulinemia and symptomatic hypoglycemia. Diabetes 63, 1315–1325. doi: 10.2337/db13-1454
Tresguerres, M., Levin, L. R., and Buck, J. (2011). Intracellular cAMP signaling by soluble adenylyl cyclase. Kidney Int. 79, 1277–1288. doi: 10.1038/ki.2011.95
Turnham, R. E., and Scott, J. D. (2016). Protein kinase A catalytic subunit isoform PRKACA. History, function and physiology. Gene 577, 101–108. doi: 10.1016/j.gene.2015.11.052
Tzingounis, A. V., Heidenreich, M., Kharkovets, T., Spitzmaul, G., Jensen, H. S., Nicoll, R. A., et al. (2010). The KCNQ5 potassium channel mediates a component of the afterhyperpolarization current in mouse hippocampus. Proc. Natl. Acad. Sci. U.S.A. 107, 10232–10237. doi: 10.1073/pnas.1004644107
Ullrich, S., Su, J., Ranta, F., Wittekindt, O. H., Ris, F., Rösler, M., et al. (2005). Effects of IKs channel inhibitors in insulin-secreting INS-1 cells. Pflugers Arch. Eur. J. Physiol. 451, 428–436. doi: 10.1007/s00424-005-1479-2
Unoki, H., Takahashi, A., Kawaguchi, T., Hara, K., Horikoshi, M., Andersen, G., et al. (2008). SNPs in KCNQ1 are associated with susceptibility to type 2 diabetes in East Asian and European populations. Nat. Genet. 40, 1098–1102. doi: 10.1038/ng.208
Vallon, V., Grahammer, F., Volkl, H., Sandu, C. D., Richter, K., Rexhepaj, R., et al. (2005). KCNQ1-dependent transport in renal and gastrointestinal epithelia. Proc. Natl. Acad. Sci. U.S.A. 102, 17864–17869. doi: 10.1073/pnas.0505860102
Walsh, K. B., and Kass, R. S. (1991). Distinct voltage-dependent regulation of a heart-delayed IK by protein kinases A and C. Am. J. Physiol. 261, C1081–C1090.
Walsh, K. B., Kass, R. S., Reiken, S., Motoike, H., D’Armiento, J., Marks, A. R., et al. (1988). Regulation of a heart potassium channel by protein kinase A and C. Science 242, 67–69. doi: 10.1126/science.2845575
Wang, H. S., Pan, Z., Shi, W., Brown, B. S., Wymore, R. S., Cohen, I. S., et al. (1998). KCNQ2 and KCNQ3 potassium channel subunits: molecular correlates of the M-channel. Science 282, 1890–1893. doi: 10.1126/science.282.5395.1890
Wang, Q., Curran, M. E., Splawski, I., Burn, T. C., Millholland, J. M., VanRaay, T. J., et al. (1996). Positional cloning of a novel potassium channel gene: KVLQT1 mutations cause cardiac arrhythmias. Nat. Genet. 12, 17–23. doi: 10.1038/ng0196-17
Warth, R., Garcia Alzamora, M., Kim, J., Zdebik, A., Nitschke, R., Bleich, M., et al. (2002). The role of KCNQ1/KCNE1 K+ channels in intestine and pancreas: lessons from the KCNE1 knockout mouse. Pflügers Arch. Eur. J. Physiol. 443, 822–828. doi: 10.1007/s00424-001-0751-3
Weckhuysen, S., Ivanovic, V., Hendrickx, R., Coster, R., Van Hjalgrim, H., Møller, R. S., et al. (2013). Extending the KCNQ2 encephalopathy spectrum: clinical and neuroimaging findings in 17 patients. Neurology 81, 1697–1703. doi: 10.1212/01.wnl.0000435296.72400.a1
Weckhuysen, S., Mandelstam, S., Suls, A., Audenaert, D., Deconinck, T., Claes, L. R. F., et al. (2012). KCNQ2 encephalopathy: emerging phenotype of a neonatal epileptic encephalopathy. Ann. Neurol. 71, 15–25. doi: 10.1002/ana.22644
Weigand, I., Ronchi, C. L., Rizk-Rabin, M., Dalmazi, G., Di Wild, V., Bathon, K., et al. (2017). Differential expression of the protein kinase A subunits in normal adrenal glands and adrenocortical adenomas. Sci. Rep. 7:49.
Welch, E. J., Jones, B. W., and Scott, J. D. (2010). Network with the AKAPs context-dependent regulation of anchored enzymes. Mol. Interv. 10, 86–97. doi: 10.1124/mi.10.2.6
Wickenden, A. D., Zou, A., Wagoner, P. K., and Jegla, T. (2001). Characterization of KCNQ5/Q3 potassium channels expressed in mammalian cells. Br. J. Pharmacol. 132, 381–384. doi: 10.1038/sj.bjp.0703861
Wong, W., and Scott, J. D. (2004). AKAP signalling complexes: Focal points in space and time. Nat. Rev. Mol. Cell Biol. 5, 959–970. doi: 10.1038/nrm1527
Xu, Q., Chang, A., Tolia, A., and Minor, D. L. (2013). Structure of a Ca2+/CaM:Kv7.4 (KCNQ4) B-helix complex provides insight into M current modulation. J. Mol. Biol. 425, 378–394. doi: 10.1016/j.jmb.2012.11.023
Yamagata, K., Senokuchi, T., Lu, M., Takemoto, M., Fazlul Karim, M., Go, C., et al. (2011). Voltage-gated K+ channel KCNQ1 regulates insulin secretion in MIN6 β-cell line. Biochem. Biophys. Res. Commun. 407, 620–625. doi: 10.1016/j.bbrc.2011.03.083
Yasuda, K., Miyake, K., Horikawa, Y., Hara, K., Osawa, H., Furuta, H., et al. (2008). Variants in KCNQ1 are associated with susceptibility to type 2 diabetes mellitus. Nat. Genet. 40, 1092–1097.
Yazawa, K., and Kameyama, M. (1990). Mechanism of receptor-mediated modulation of the delayed outward potassium current in guinea-pig ventricular myocytes. J. Physiol. 421, 135–150. doi: 10.1113/jphysiol.1990.sp017937
Zhang, J., Juhl, C. R., Hylten-Cavallius, L., Salling-Olsen, M., Linneberg, A., Holst, J. J., et al. (2020). Gain-of-function mutation in the voltage-gated potassium channel gene KCNQ1 and glucose-stimulated hypoinsulinemia – case report. BMC Endocr. Disord. 20:38. doi: 10.1186/s12902-020-0513-x
Zhang, J., and Shapiro, M. S. (2012). Activity-dependent transcriptional regulation of M-type (Kv7) K+ channels by AKAP79/150-mediated NFAT actions. Neuron 76, 1133–1146. doi: 10.1016/j.neuron.2012.10.019
Keywords: Kv7 (KCNQ), cAMP, PKA, EPAC, physiology
Citation: van der Horst J, Greenwood IA and Jepps TA (2020) Cyclic AMP-Dependent Regulation of Kv7 Voltage-Gated Potassium Channels. Front. Physiol. 11:727. doi: 10.3389/fphys.2020.00727
Received: 06 May 2020; Accepted: 04 June 2020;
Published: 30 June 2020.
Edited by:
Mark S. Shapiro, The University of Texas Health Science Center at San Antonio, United StatesReviewed by:
Naoto Hoshi, University of California, Irvine, United StatesMaria Virginia Soldovieri, University of Molise, Italy
Copyright © 2020 van der Horst, Greenwood and Jepps. This is an open-access article distributed under the terms of the Creative Commons Attribution License (CC BY). The use, distribution or reproduction in other forums is permitted, provided the original author(s) and the copyright owner(s) are credited and that the original publication in this journal is cited, in accordance with accepted academic practice. No use, distribution or reproduction is permitted which does not comply with these terms.
*Correspondence: Thomas A. Jepps, dGplcHBzQHN1bmQua3UuZGs=