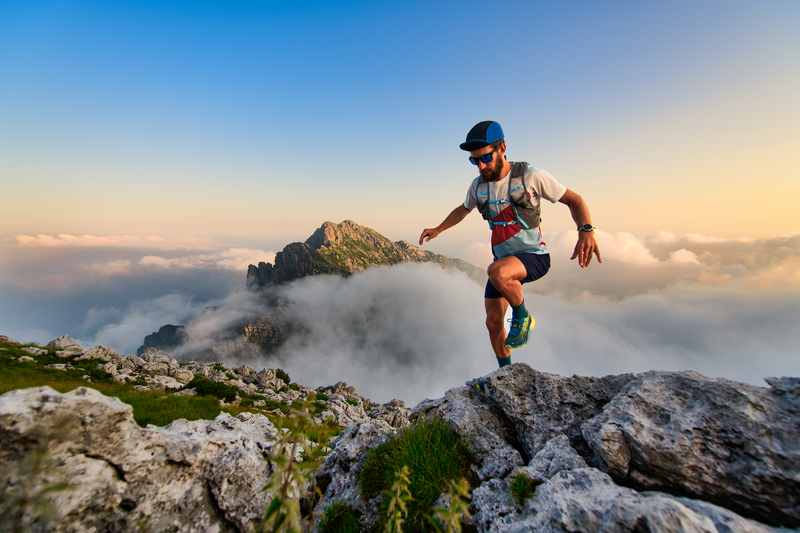
94% of researchers rate our articles as excellent or good
Learn more about the work of our research integrity team to safeguard the quality of each article we publish.
Find out more
REVIEW article
Front. Pharmacol. , 12 October 2018
Sec. Experimental Pharmacology and Drug Discovery
Volume 9 - 2018 | https://doi.org/10.3389/fphar.2018.01151
This article is part of the Research Topic Molecular Mechanisms and New Therapeutic Targets in Epithelial to Mesenchymal Transition (EMT) and Fibrosis View all 18 articles
P311 was first identified by the group of Studler et al. (1993) in the developing brain. In healthy, but mainly in pathological tissues, P311 is implicated in cell migration and proliferation. Furthermore, evidence in models of tissue fibrosis points to the colocalization with and the stimulation of transforming growth factor β1 by P311. This review provides a comprehensive overview on P311 and discusses its potential as an anti-fibrotic target.
Neuronal protein 3.1 (P311) is a small intracellular protein from an unknown family, whose transcript was first identified by Studler et al. (1993) in a differential screening comparing striatal cells from two different stages of brain development. P311 knockout mice have learning and memory deficiency and a disturbed pain affection (Sun et al., 2008; Taylor et al., 2008). During the past years, light has been shed on the broader expression pattern of P311 and its function in both health and pathology. P311 has proven to be a multifunctional protein with important implications in development, disease, and regeneration. For this review, we explore P311 functions and discuss possible implications of P311 in the development of tissue fibrosis by highlighting its role in tissue regeneration, cell migration, and its interaction with the pro-fibrotic cytokines transforming growth factor β1 and 2 (TGFβ1 and 2).
Tissue fibrosis can be defined as the hardening of epithelial tissues due to accumulation of secreted collagens. Initially it starts as a wound healing response, but upon repeated insults, the scarring increases and becomes pathogenic (Friedman et al., 2013). Under normal conditions, wound healing undergoes three phases; an inflammatory, a proliferative, and a regenerative phase. These phases are well characterized for liver fibrosis (Figure 1), while lung and kidney present a similar fibrotic process (Friedman et al., 2013).
FIGURE 1. The development of tissue fibrosis progresses in three phases: inflammatory phase, proliferation phase, and regeneration phase. Upon repeated injury, the process continuously repeats phase one and two, without resolution of the scar tissue. The example represents the activation mechanism of hepatic stellate cells during the development of liver fibrosis. HSC, hepatic stellate cell.
Upon tissue injury, we can distinguish different origins of pro-fibrogenic stimuli. Damaged endothelium and epithelium as well as activated platelets will secrete pro-fibrogenic and pro-inflammatory cytokines. Furthermore, they also attract extra mesenchymal cells, inter-organ and organ resident cells to the site of injury by secreting chemokines (Dranoff et al., 2004; Armulik et al., 2005; Darby et al., 2014).
Due to the inflammatory environment, fibroblasts will activate and differentiate into myofibroblasts. These will proliferate, gain tensile forces, and will secrete chemokines to attract other fibroblasts. Myofibroblasts at the site of injury will secrete a massive amount of matrix proteins, replacing the fibrin matrix with a dense collagen-rich one (Darby et al., 2014; Simone et al., 2015).
Once the insult is ceased, damage and inflammation is diminished and collagens will be degraded, mainly by matrix metalloproteinases. This will allow re-epithelialization. Most of the myofibroblasts present undergo apoptosis during healing or revert to an inactive phenotype as was shown in the liver (Desmouliere et al., 1995; Kisseleva et al., 2012; Darby et al., 2014).
When the injury is chronic, the first two phases are continuously repeated and the matrix resolution and epithelial restoration do not occur. The fibrotic site will expand, the organ tissue will continue to harden, leading to cellular dysfunction and eventually organ failure (Figure 1). This pathological process has been described in several organs, but is most intensely studied in liver, kidney, and lung. Hepatic stellate cells, renal mesangial cells, and resident lung fibroblasts, respectively, are the organ-specific resident cells that transdifferentiate to myofibroblasts and contribute to the scarring (Ardaillou et al., 1999; Friedman, 2008; Barkauskas and Noble, 2014). During the proliferative phase, activation of these cells is triggered by different pathways; damaged epithelial cells will stimulate fibroblast activation either by their released nucleotides or by expressing integrins, which will then mechanically activate TGFβ1 that is transiently stored in the extracellular matrix (Munger et al., 1999; Dranoff et al., 2004; Zhan et al., 2006; Giacomini et al., 2012). Platelets present at the site of injury will recruit fibroblasts as well as inflammatory cells, which secrete pro-fibrogenic cytokines like tumor necrosis factor α, interleukin (IL) 1β, IL13, and IL17 (Friedman et al., 2013; Darby et al., 2014). Upon activation, the myofibroblasts themselves will secrete chemokines, cytokines, as well as oxygen radicals, leading again to increased stress, immune response, and myofibroblast activation (Friedman et al., 2013). Once fibrosis is initiated, it is a self-stimulating system; matrix-stored TGFβ1 is activated by the epithelial cells, this active TGFβ1 in turn activates fibroblasts, which will then recruit and activate even more fibroblasts, which will produce TGFβ1 and so on (Ignotz and Massague, 1987; Wang et al., 1996; Giacomini et al., 2012). In parallel, more myofibroblasts means more collagen secretion and crosslinking, finally leading to stiffening of the extracellular matrix. A stiff matrix in turn stimulates proliferation, drives cells to activate, inhibits the production of matrix digestive enzymes, and facilitates the activation of TGFβ1 in the matrix (Li et al., 2007, Liu et al., 2010; Olsen et al., 2011; Huang et al., 2012).
The organ resident stromal cells are important targets to attenuate fibrosis development. Interfering with their recruitment, proliferation, TGFβ1 response, or collagen secretion already greatly delays or sometimes even stops fibrosis progression in animal models of fibrosis (Munger et al., 1999; Liu et al., 2009a; Kuroda et al., 2015; Nayak et al., 2016). Unfortunately, these approaches have not yet evolved in clinically approved therapeutics (Schuppan and Kim, 2013; Schon et al., 2016). P311 is involved in several of these fibrosis stimulating pathways and might represent an interesting target for the development of anti-fibrotic drugs. We will discuss its involvement in cell migration, regeneration, and development in different tissues.
The first studies on P311 reported the transcript in the germinal zones of the brain at embryonic day 17, the transcript was observed in the striatum and the superficial cortical layers at E20, suggesting that the gene was expressed in cells from the second germinal migration wave. The expression persists during adulthood, where it is present in several brain regions with active postnatal neurogenesis like the cerebellum, hippocampus, and the olfactory bulb (Studler et al., 1993). P311 has been alternatively named pentylenetetrazole 17, since it potentiates an inward calcium current upon pentylenetetrazole administration in neurons, mimicking epileptic bursting (Kajiwara et al., 1995). The P311 open reading frame is located on chromosome 18 in mice, on chromosome 5 in humans, and has been highly conserved among mice, humans, rhesus monkeys, dogs, etc. This sequence is translated into an 8 kDA protein of 68 amino acids and contains a PEST domain responsible for the fast degradation by ubiquitin-dependent degradation as well as by an unknown metalloprotease, resulting in a half-life of approximately 5 min (Studler et al., 1993; Taylor et al., 2000). Research for P311 binding partners, using a predictor of naturally disordered regions analysis, suggested that P311 is an intrinsically disordered protein that needs an interaction partner to acquire a tertiary structure. Indeed, P311 was shown to interact with several cytoskeletal proteins such as Filamin A and non-muscle myosin heavy chain 9 (MYH9) and with eukaryotic translation initiation factor 3, subunit B (eIF3b), a component of the translation initiation complex (Yue et al., 2014).
Fibroblast migration is a key event for organ development as well as fibrosis (Shimizu et al., 2001; Hattori et al., 2004; Scholten et al., 2011). P311 is described by different groups to play an important role during chemokine-induced cell migration (Studler et al., 1993; Mariani et al., 2001; McDonough et al., 2005; Guimaraes et al., 2015). The first study ever describing P311 already detected the mRNA in migrating cells in the developing brain (Studler et al., 1993). A study aiming at discovering mechanisms involved in invading glioma cells, determined that P311 is highly expressed in the invasive rim of human glioma tumors, when compared to P311 expression in the tumor core (∼threefold). Furthermore, knockdown of P311 in human glioma cells (SF767) reduced cell migration in vitro, while when cultured on glioma-derived extracellular membrane, glioma cells migrated at a higher rate and expressed higher P311 levels (Mariani et al., 2001). This correlation between P311 and cell migration was also shown during liver fibrosis. Upon liver injury, hepatic stellate cells activate, resulting in contractile and migrating hepatic stellate cells with increased P311 transcription (Guimaraes et al., 2015). When activated chronically, these cells deposit high levels of collagen, resulting in liver fibrosis and finally cirrhosis. Knocking down P311 expression in cultured primary hepatic stellate cells reduced chemokine-dependent cell migration (Guimaraes et al., 2015). Furthermore, immunohistochemical stainings demonstrated that the P311 protein was present in nuclei as well as at leading edges of migrating hepatic stellate cells and human astrocytes (Taylor et al., 2000; Guimaraes et al., 2015). In hepatic stellate cells and glioma cells, less P311 expression also resulted in less lamellipodia, most likely resulting in a reduced migration (Mariani et al., 2001; Guimaraes et al., 2015).
P311-stimulated migration is strongly dependent on the half-life of the protein, which is regulated by constitutive phosphorylation of a serine (Ser59, situated right next to the PEST domain) by protein kinase C δ, ε, and ζ, resulting in a short-lived P311 protein and no migration. β1 integrin signaling on the other hand stimulates its dephosphorylation and consequently cell migration. Upon plating U118 cells on a motility-activating substrate Ser59’s phosphorylation is reduced, P311 is not degraded and indirectly stimulates ras-related C3 botulinum toxin substrate 1 (RAC1) GTPase activity (McDonough et al., 2005). Additionally, mice with RAC1-deficient fibroblasts have impaired cutaneous wound healing and the fibroblasts have a less activated phenotype (Liu et al., 2009b). RAC1 GTPase was also shown to be important for glioma cell migration and is highly expressed in cells performing mesenchymal migration (Shaw et al., 1997; Huang et al., 2014). This type of migration acts “slow” and occurs with a lot of adhesions and integrin/filaminA interactions. On the other hand, amoeboid migration is faster and is characterized by less adhesions and mainly uses Ras-related protein A (RALA) and Ras homolog gene family, member A (RHOA) GTPases instead of RAC1 (Figure 2). P311 does not seem to be specific for one or the other migration mechanism. Fibroblasts overexpressing P311 can migrate in both fashions, depending on the culture dish coating or cytokine treatment, such as TGFβ1 (Shi et al., 2006; Huang et al., 2014). Correspondingly, human epidermal stem cells initiate P311 expression in response to skin damage to promote wound re-epithelialization. This was shown in vitro in human epidermal stem cells in which overexpression of P311 resulted in a faster migration through stimulation of RHOA and RAC1 activity while in P311 knockout mice skin wounds healed slower (Yao et al., 2017).
FIGURE 2. P311 is expressed in migrating cells. P311 can induce RAC1 GTPase to induce slow mesenchymal migration, whether it stimulates RALA GTPase, inducer of amoeboidal migration, is unknown. Nevertheless, independent of the migration type, P311 is expressed in migrating cells. Upon stimulation with HGF/c-Met, cells will initiate migration but they will downregulate P311 expression.
Filamin A, an interconnecting protein between F-actin and β1 integrin binding protein, was identified as a direct binding protein of P311 in glioma cells. Later on, this was confirmed in 3T3 cells overexpressing a Myc-tagged P311 protein. Co-immune precipitation and mass spectrometric analysis demonstrated that P311 interacts with cytoskeletal proteins MYH9, actin β, and filamin A (Figure 3). Filamin A was shown to interact with integrin β1, present in the cell membrane, to activate TGFβ1 and regulate cell motility (McDonough et al., 2005). Actin β is a fundamental cytoskeleton protein that is one of the driving forces for cell protrusions (Bunnell et al., 2011). MYH9 binds transiently to the cytoskeleton, by which it regulates cell spreading, adhesion, and migration (Huang et al., 2009; Liu et al., 2011; Casalou et al., 2014). One study on hepatic stellate cells demonstrated that MYH9 enabled intracellular Ca2+ release, a feature that was also described to P311 when administered to neuronal cells (Kajiwara et al., 1995; Liu et al., 2011). Together with F-actin, MYH9 is also involved in the formation of circular dorsal ruffles upon PDGF-BB stimulation, which recycle integrins, remodel the cytoskeleton during migration, and their presence is enhanced by RAC1 (Casalou et al., 2014; Figure 3). Further research regarding the role of P311’s interaction with these two proteins and the potential effect on circular dorsal ruffle formation still needs to be done (McDonough et al., 2005).
FIGURE 3. P311 has different interacting proteins. Co-immune precipitation analysis determined Filamin A, MYH9, Actin ß, and eIF3b as P311 interacting proteins. Whether they need P311 to correctly perform their function still remains uncertain for most of these binding partners.
In contrast to the P311 overexpression migration studies, the group of Taylor et al. (2000) reported that P311 was decreased upon hepatocyte growth factor/scatter factor (HGF/SF) c-MET induced migration in a human leiomyosarcoma cell line (SK-LMS). The cells became metastatic and obtained increased tumorigenic capacities (Jeffers et al., 1996; Taylor et al., 2000). The fact that migration of these cells was driven by c-Met was not out of the ordinary, since it was already shown that both the mesenchymal and amoeboidal migration pathways in carcinoma cells are stimulated by the c-Met pathway (Huang et al., 2014; Figure 2). While low P311 mRNA levels can be observed in glioma cell lines with a high expression of Met-HGF/SF, a tumor suppressive function for P311 was excluded since P311 overexpression in U118 glioma cells did not interfere with tumor growth in vivo. This suggested that diminished P311 expression is the result of a more transformed and tumorigenic phenotype. A similar observation was made for terminally differentiated human cortical neuronal cells (HCN-1A and HCN-2) where nerve growth factor exposure decreased P311 expression (Taylor et al., 2000).
During fibrosis, organ resident cells transform into myofibroblasts and accumulate at the site of injury due to chemokines. Interfering with P311 expression or function and thus with the migration potential of these myofibroblasts could be an opportunity to dampen scar formation. However, one needs to consider whether this would not lead to an accumulation of “triggered or activated” myofibroblast far from the injury site which might not be able to contribute to the resolution of the fibrosis once the injury subsides.
Tissue development and regeneration, at least in brain, muscle, and lung, can be influenced by P311 expression (Studler et al., 1993; Fujitani et al., 2004; Siller-Lopez et al., 2004; Ooi et al., 2006). The differentiation of a human neuroblastoma cell line (RTBM1), induced by retinoic acid, was accompanied by an increased P311 expression and neurite outgrowth (Ueda, 2001). This is not surprising, since these probing extensions migrate toward the neighboring cells using lamellipodia and filopodia, mediated by the RAC1 pathway (Alberts et al., 2008). Upon neuron axotomy in adult rats, P311 is transiently upregulated between day 3 and day 21 post-surgery (Fujitani et al., 2004). Overexpressing P311 in undifferentiated PC12 cells (rat adrenal gland) or differentiated dorsal root ganglions induced neurite outgrowth, due to the induction of cyclin-dependent kinase inhibitor p21waf1. During neuronal differentiation p21waf1 is up-regulated to prevent cells from entering the cell cycle (Billon et al., 1996; van Grunsven et al., 1996) and can block Rho kinase activity leading to neurite outgrowth (Erhardt and Pittman, 1998; Olson et al., 1998; Yamashita et al., 1999; Tanaka et al., 2002; Fujitani et al., 2004; Tanaka et al., 2004; Figure 4). Moreover, facial neurons that were transfected in vivo with P311 cDNA regenerated almost three times as fast as non-transfected facial neurons. This could suggest that P311 can interfere with Rho signaling by inducing p21waf1 expression through a thus far unknown mechanism (Fujitani et al., 2004). During human and mouse lung morphogenesis, P311 expression peaks during the saccular and alveolar formation. Smokers who develop emphysema express less P311 compared to smokers without emphysema. Mouse pups treated with dexamethasone, an inhibitor of alveolization, showed a decreased P311 expression when compared to the saline-treated littermates (Zhao et al., 2006). Together this suggests that P311 seems to be involved in the alveolar repair upon injury.
FIGURE 4. P311 induces the expression of p21Waf1. p21Waf1 stimulates neurite outgrowth by blocking cell proliferation and Rho kinase. This is stimulated by both Ras signaling and P311, although it is not yet known if the latter goes directly or indirectly.
In muscular tissue, P311 expression increases during embryonic pig development and stays active postnatally (Ooi et al., 2006). The opposite was demonstrated for muscle atrophy by two independent studies, one on rats and one on piglets, both looking for molecular patterns that occur during muscle atrophy. Muscle wasting due to skeletal muscle atrophy resulted in a decreased P311 expression, together with other muscle growth stimulating genes, and an increase in E3 ubiquitin ligase enzyme (MAFbx) (Lecker et al., 2004; Ooi et al., 2006). An artificial induction of P311 expression in fibroblasts (3T3 and C3H10) and undifferentiated muscle cell lines (C2C12) on the other hand, induces the expression of muscle-specific transcription factors like myogenic differentiation 1, serum responsive factor (SRF), and myosin heavy chain 4, but less of smooth or skeletal muscle-specific genes. The proliferation rate of these cells increased, but differentiation toward myotubes was attenuated. Myogenic factor 5 (Myf5) was downregulated in all studies, which can be explained by the fact that cell proliferation inhibits Myf5 (Lindon et al., 1998; Pan et al., 2002; Ooi et al., 2006). One of the most important genes that was upregulated is SRF, which interacts with its cofactor myosin light chain kinase 1 to bind to the alpha-smooth muscle actin (α-SMA) promoter in response to matrix stiffness and regulates the expression of other pro-fibrotic genes after TGFβ1 stimulation (Hirschi et al., 2002; Huang et al., 2012). Furthermore, it was shown that SRF has many target genes involved in cytoskeletal organization, migration, and cell proliferation, suggesting that this might be one of P311’s key targets (Soulez et al., 1996; Schratt et al., 2002; Miano et al., 2007; Nolte et al., 2013). Similar to cortical neuron differentiation, when terminal differentiation was induced in C2C12 cells by the broad spectrum inducer of muscle differentiation calcineurin, P311 expression was reduced and accompanied by less cell proliferation (Ooi et al., 2006; Bassel-Duby and Olson, 2003). A correlation between P311 levels and cell proliferation is also described in hepatic stellate cells, invasive glioma cells, and sensory epithelia from the inner ear. Knocking down P311 in hepatic stellate cells reduced cell proliferation in vitro. In glioma cells on the other hand, overexpression of anti-tumorigenic gene, melanoma antigen family D1 (DLXIN-1), resulted in less proliferation due to less matrix metalloproteinases (MMP)2 and 9 activity and through direct interaction with P311, DLXIN-1 blocks P311’s invasive function. Finally, P311 is more expressed in regenerating sensory hair cells compared to hair cells that do not renew (Hawkins et al., 2006; Reddy et al., 2011; Guimaraes et al., 2015).
The TGFβ1 pathway is one of the main pathways involved in tissue fibrosis. TGFβ1 is a pro-fibrogenic agent, whose precursor [TGFβ bound to a latency-associated protein (LAP)] is stored in the matrix and its activated by proteolytic cleavage by MMP-2, MMP-9, or thrombospondin-1 or mechanical cleavage by integrins, upon tissue damage (Leask and Abraham, 2004; Liu et al., 2009a). Active TGFβ1 accumulates and stimulates an epithelial-to-mesenchymal transition (EMT)-like conversion, and the conversion of fibroblasts and mesenchymal cells into myofibroblasts (Leask and Abraham, 2004; Desmouliere et al., 2005; Breitkopf et al., 2006; Bae et al., 2012). Upon stimulation, TGFβ1 binds to the heteromeric TGFβ1 receptor followed by signaling to TGFβ receptor I kinase, which phosphorylates the receptor-activated SMAD family members, SMAD2 and 3, intracellularly. Phosphorylated SMAD2/3 binds to SMAD4, after which the complex translocates to the nucleus, where the complex binds to p300 and stimulates the transcription of collagen and Acta2, as well as other factors involved in cell proliferation and differentiation of mesenchymal cells (Heldin et al., 1997; Leask and Abraham, 2004; Breitkopf et al., 2006; Bae et al., 2012). Apart from its role in migration and proliferation, P311 has been strongly associated with the TGFβ1 pathway.
The mechanisms by which P311 can control the TGFβ1 pathway are numerous and depending on the experimental setup it can be stimulatory or inhibitory. P311-overexpressing 3T3 cells, which do not express endogenous P311, can differentiate into myofibroblasts but have inactive TGFβ proteins. In these cells P311 binds to LAP, rendering TGFβ1 and 2 inactive and preventing auto-induction (Piek et al., 2001; Pan et al., 2002; Paliwal et al., 2004). Consequently, these cells express less TGFβ1 and β2, collagen 1, and MMP 2 and 9, suggesting that P311 might be part of an intrinsic anti-fibrotic mechanism in myofibroblasts (Pan et al., 2002). In a renal tubular epithelial cell line (NRK-52E) P311 can inhibit EMT. TGFβ1 treatment stimulates proliferation and can induce EMT in these cells, accompanied with a downregulation of E-cadherin and an α-SMA upregulation. These effects can be blocked by overexpression of P311 (Qi et al., 2015). These data indicate that overexpression of P311 can reverse or prevent EMT of renal tubular cells and 3T3 fibroblasts in vitro (Pan et al., 2002; Paliwal et al., 2004). On the other hand, several studies demonstrate that P311 stimulates TGFβ1 and 2 expression in vascular and lung smooth muscle cells (Badri et al., 2013; Yue et al., 2014). P311 null mice suffer from vascular hypotension, due to a shortage in TGFβ1 and β2 proteins and the absence of P311 in vascular smooth muscle cells results in less Rho A activity and consequently less contractility (Loirand and Pacaud, 2010). In these P311 null mice there is a striking discrepancy between TGFβ protein levels and mRNA levels, i.e., high Tgfβ1/2 mRNA levels but low protein levels, suggesting that P311 is involved in the translation of Tgfβ1/2. Mice overexpressing P311 on the other hand are hypertensive. This was confirmed in samples from normo- and hypertensive patients. P311, and consequently TGFβ protein levels, are highly expressed in tissues of hypertensive patients when compared to healthy ones (Badri et al., 2013).
P311 also appears to be involved in the formation of hypertrophic scar, which is the result of an imbalanced wound healing and is a fibrosis-like process (Singer and Clark, 1999). P311 is upregulated and co-expressed with α-SMA, collagen 1, and TGFβ1 in the scar tissue, while there is no expression detected in healthy skin. P311 overexpression in healthy skin induces the expression of TGFβ1 and increases cell proliferation, while interfering with P311 in fibroblasts derived from scar tissue reduces TGFβ1 expression and cell contractility (Tan et al., 2010). In P311 knockout mice, scars from excision wounds showed reduced TGFβ1-3 protein levels, less collagen deposition, and consequently reduced tensile strength and scar stiffness (Cheng et al., 2017). Increased P311 expression can also be detected in tubular epithelial cells from patients with chronic kidney disease, colocalized and correlated with TGFβ1, while healthy kidney tissue does not express either P311 or TGFβ1 (Wang et al., 2010; Yao et al., 2015). The latter observation is confirmed in vivo in mice after an unilateral ureteral obstruction, where P311 protein seems to be colocalized with α-SMA and TGFβ1 in acidophilic degeneration regions. Unilateral ureteral obstruction in P311 null mice results in lower levels of collagen type 1 and α-SMA, a reduced expression of TGFβ receptor 1 and 2 (TGFβR1 and 2), and reduced phosphorylation of SMAD2/3. As indicated in earlier reports, Tgfβ1 mRNA levels in these mice did not differ between the wild type and P311 null mice, but they present reduced TGFβ1 protein when P311 was absent (Yao et al., 2015).
Finally, Yue et al. (2014) gave conclusive evidence that P311 is indeed involved in Tgfβ1 translation by showing that it interacts directly with eukaryotic translation initiation factor 3, subunit B (eIF3b), and a protein of the translation initiation complex. In addition, P311 forms a complex with the 5′UTRs of Tgfβ1, 2, and 3 by which it recruits Tgfβ mRNA and stimulates its translation (Yue et al., 2014). 3T3 fibroblasts without P311 do translate Tgfβ1, but an exogenous expression of P311 can increase the levels of total and active TGFβ1 proteins, which is accompanied with increased mRNA levels due to auto-induction (Figure 5). Finally, they also showed that the interaction between P311 and eIF3b occurs on the P311–eIF3b binding motif, which is a highly conserved sequence among different eukaryotes (Yue et al., 2014). These results were confirmed in a study on skin re-epithelialization, where they indicate P311 as a driver of epidermal to mesenchymal transition upon skin damage. Epidermal stem cells expressing exogenous P311 had increased active and LAP-bound TGFβ1 protein levels, increased expression of TgfβR1 and 2 mRNA, and increased SMAD2/3 phosphorylation, while gaining mesenchymal features. The P311-induced transition was blocked when TGFβRI/II kinases were inhibited. Furthermore, in these cells P311-enhanced methylation of the Tgfβ promotor and an increased 5′ and 3′ UTR luciferase activity was observed, indicating that P311 stimulates Tgfβ mRNA transcription (Li et al., 2016).
FIGURE 5. P311 stimulates Tgfβ1 translation. P311 binds to the Tgfß1 mRNA and eukaryotic translation initiation factor 3, facilitating the translation of Tgfß1 mRNA to protein.
Although contradictory results exist, it seems that there is a clear correlation between P311 and modulation of TGFβ1 protein levels and its signaling pathways; in vivo data indicates that P311 stimulates the TGFβ1 pathway, this was confirmed in cultured primary cells and cell lines (Tan et al., 2010; Wang et al., 2010; Badri et al., 2013; Yue et al., 2014; Yao et al., 2015) but contradicted in two cell lines (Pan et al., 2002; Paliwal et al., 2004; Qi et al., 2015). Nevertheless, some plausible mechanisms have been suggested to explain the observed in vivo events, indicating that P311 is an upstream regulator of the TGFβ1 pathway (Yue et al., 2014; Li et al., 2016).
Currently, different strategies are being applied in clinics/clinical trials to treat tissue fibrosis; anti-fibrotic, anti-inflammatory, anti-oxidant therapies, or combinations of the previous (Schuppan and Kim, 2013; Huang et al., 2015; Schon et al., 2016; Wang et al., 2016). Blocking the TGFβ1 pathway with, for example, fresolimumab or lysyl oxidase ligand 2 antibodies, or stimulating the cells with peroxisome proliferator-activated receptor γ agonists like pioglitazone, are both anti-fibrotic strategies (Rice et al., 2015; Wang et al., 2016). Interferon γ and Ribavirin on the other hand are administered to reduce inflammation (Fernandez et al., 2006), while vitamin E and anthocyanin are two anti-oxidation compounds (Sanyal et al., 2010; Wang et al., 2016). These compounds work well to reduce fibrosis, but are, however, hampered by unwanted side effects (Schon et al., 2016). Targets with a wide range of effects like TGFβ1 or interferon inhibition are supposed to be very efficient to reduce fibrosis, but since they are not tissue-specific, they come with systemic complications. Subjects suffer from mild adverse side effects like anemia, depression, rash, fever, myalgia, internal bleeding, and sometimes even major side effects like worsening of the fibrosis or developing cutaneous neoplasms (Fernandez et al., 2006; Lacouture et al., 2015; Rice et al., 2015). A therapeutic target involved specifically in fibrosis development is needed, preferably combined with a precise delivery method (Gandhi, 2017).
The question whether P311 is an interesting therapeutic target to be considered for anti-fibrotic therapy still remains unanswered, but circumstantial evidence indicates it might be a good candidate. Tissue fibrosis is mainly characterized by proliferative and contractile resident myofibroblasts and myofibroblast-like cells (Friedman et al., 2013; Darby et al., 2014). A process in which TGFβ1 is a master regulator that initiates a self-sustaining loop of attracting and activating myofibroblasts (Ignotz and Massague, 1987; Munger et al., 1999; Li et al., 2007; Giacomini et al., 2012). Studies provided evidence that P311 plays a role in different processes that are initiated during fibrosis development and stimulates the translation of Tgfβ1 mRNA (Figures 5, 6). Moreover, in P311 null mice, the TGFβ1 pathway is clearly less active, which results in hypotensive mice and reduces kidney fibrosis (Badri et al., 2013; Yao et al., 2015). With regard to organs like liver and lung, there is not yet much known regarding the interplay of P311 and TGFβ1 pathways (Table 1). It was demonstrated that P311 could induce a myogenic phenotype in fibroblasts and stimulate its migration (Taylor et al., 2000; Pan et al., 2002; McDonough et al., 2005; Shi et al., 2006; Guimaraes et al., 2015; Figure 6). Finally, P311 might have a role in integrin β1 recycling, since P311 interacts with Filamin A and MYH9, while the former is an interconnecting protein between F-actin and integrin β1, the latter is responsible for integrin β1 recycling with F-actin, which is stimulated by the RAC1 pathway (McDonough et al., 2005; Huang et al., 2009; Liu et al., 2011; Casalou et al., 2014; Yue et al., 2014; Figures 3, 6). Silencing P311 in vivo or interference with P311 functionality might hamper the fibrogenic process and could allow the tissue to enter the regeneration phase.
FIGURE 6. Concluding scheme. P311 is involved in a wide range of pathways. Most importantly, it stimulates cell proliferation, migration, and contraction, which are core characteristics of tissue fibrosis.
The safety of P311 inhibition can be estimated as relatively high, since P311-deficient mice do not present a detrimental phenotype, indicating that development and homeostasis of the body might not be influenced by P311 inhibition. However, since P311 stimulates cell migration, proliferation, and regeneration it indicates that untargeted delivery of siRNA or a compound that could block P311 function in vivo might randomly interfere with crucial processes for tissue repair. This can be deduced from studies that showed that patients with less P311 have a decreased alveolar repair after emphysema (Zhao et al., 2006). Therefore, a specific delivery of a P311 siRNA or inhibitory compound to resident myofibroblastic cells in the fibrotic organs would be essential. Local delivery of compounds or siRNA, either free or in nanocomplexes like liposomes, is not always straightforward. While hydrogels and aerosols can achieve this for the treatment of scars or lung fibrosis, respectively (Ivanova et al., 2013; Manosroi et al., 2013; Gumel et al., 2015; Sun, 2017), targeting myofibroblasts in the liver or kidney requires targeted nanomedicine. This can be achieved by using targeted viral vectors (Reetz et al., 2013; Cooney et al., 2018), but over the last decennia a wide array of non-viral delivery systems has been developed for both the delivery of siRNAs and compounds (Table 2). For example, delivery of siRNA loaded in targeted liposomes, as already been shown for hepatic and pancreatic fibrosis (Sato et al., 2008; Davies et al., 2014), or an ultrasound microbubble delivery of shRNA to kidneys (Li et al., 2018). Such a specific inhibition of P311 in resident myofibroblasts might interfere with the fibrotic cascade by decreasing cell migration and proliferation and would interfere with the increased TGFβ1 protein levels. Due to this, the accumulation of myofibroblasts at the site of injury might be attenuated and the tissue would be able to initiate resolution of the extracellular matrix.
TABLE 2. Overview of non-viral delivery systems and targeting molecules that are being used for the delivery of compounds and siRNAs to fibrotic organs.
A large body of evidence suggests that P311 is necessary for correct TGFβ1 production and its inhibition might result in a less myofibroblastic phenotype, decreased TGFβ1 protein expression and collagen deposition. Importantly, few studies have explored the in vivo effects of P311 modulation and its consequence to fibrosis (Yao et al., 2015). Thus, the potential of P311 as an anti-fibrotic target has still to be investigated in other organs, such as lung, liver, and skin, all of which have a high propensity to develop fibrosis. Nevertheless, the possibility of combining specific delivery targeting (i.e., targeted liposomes) and P311 inhibition may prove to be an efficient and safe treatment due to the distinct expression and role of this protein in fibrosis development.
LS and LvG conceived the work and provided the layout for it. LS and IM drafted the work. LvG critically revised the text and figures and approved the final manuscript for publication.
This work has been funded by the Vrije Universiteit Brussel and by the Instituut voor Innovatie door Wetenschap en Technologie (IWT/SB 121416).
The authors declare that the research was conducted in the absence of any commercial or financial relationships that could be construed as a potential conflict of interest.
Alberts, B., Johnson, A., Lewis, J., Raff, M., Roberts, K., and Walter, P. (2008). “Neural development,” in Molecular biology of the cell, eds M. Anderson, and S. Granum (New York, NY: Garland Science), 1383–1397.
Ardaillou, R., Chansel, D., Chatziantoniou, C., and Dussaule, J. C. (1999). Mesangial AT1 receptors: expression, signaling, and regulation. J. Am. Soc. Nephrol. 10(Suppl. 11), S40–S46.
Armulik, A., Abramsson, A., and Betsholtz, C. (2005). Endothelial/pericyte interactions. Circ. Res. 97, 512–523. doi: 10.1161/01.RES.0000182903.16652.d7
Badri, K. R., Yue, M., Carretero, O. A., Aramgam, S. L., Cao, J., Sharkady, S., et al. (2013). Blood pressure homeostasis is maintained by a P311-TGF-beta axis. J. Clin. Invest. 123, 4502–4512. doi: 10.1172/JCI69884
Bae, E., Kim, S. J., Hong, S., Liu, F., and Ooshima, A. (2012). Smad3 linker phosphorylation attenuates Smad3 transcriptional activity and TGF-beta1/Smad3-induced epithelial-mesenchymal transition in renal epithelial cells. Biochem. Biophys. Res. Commun. 427, 593–599. doi: 10.1016/j.bbrc.2012.09.103
Barkauskas, C. E., and Noble, P. W. (2014). Cellular mechanisms of tissue fibrosis. 7. New insights into the cellular mechanisms of pulmonary fibrosis. Am. J. Physiol. Cell Physiol. 306, C987–C996. doi: 10.1152/ajpcell.00321.2013
Bassel-Duby, R., and Olson, E. N. (2003). Role of calcineurin in striated muscle: development, adaptation, and disease. Biochem. Biophys. Res. Commun. 311, 1133–1141. doi: 10.1016/j.bbrc.2003.09.020
Billon, N., van Grunsven, L. A., and Rudkin, B. B. (1996). The CDK inhibitor p21WAF1/Cip1 is induced through a p300-dependent mechanism during NGF-mediated neuronal differentiation of PC12 cells. Oncogene 13, 2047–2054.
Blueschke, G., Boico, A., Negussie, A. H., Yarmolenko, P., Wood, B. J., Spasojevic, I., et al. (2018). Enhanced drug delivery to the skin using liposomes. Plast. Reconstr. Surg. Glob. Open 6:e1739. doi: 10.1097/GOX.0000000000001739
Breitkopf, K., Godoy, P., Ciuclan, L., Singer, M. V., and Dooley, S. (2006). TGF-beta/Smad signaling in the injured liver. Z. Gastroenterol. 44, 57–66. doi: 10.1055/s-2005-858989
Bunnell, T. M., Burbach, B. J., Shimizu, Y., and Ervasti, J. M. (2011). Beta-Actin specifically controls cell growth, migration, and the G-actin pool. Mol. Biol. Cell 22, 4047–4058. doi: 10.1091/mbc.e11-06-0582
Casalou, C., Seixas, C., Portelinha, A., Pintado, P., Barros, M., Ramalho, J. S., et al. (2014). Arl13b and the non-muscle myosin heavy chain IIA are required for circular dorsal ruffle formation and cell migration. J. Cell Sci. 127, 2709–2722. doi: 10.1242/jcs.143446
Chai, N. L., Fu, Q., Shi, H., Cai, C. H., Wan, J., Xu, S. P., et al. (2012). Oxymatrine liposome attenuates hepatic fibrosis via targeting hepatic stellate cells. World J. Gastroenterol. 18, 4199–4206. doi: 10.3748/wjg.v18.i31.4199
Chang, M. L., Yeh, C. T., Chang, P. Y., and Chen, J. C. (2005). Comparison of murine cirrhosis models induced by hepatotoxin administration and common bile duct ligation. World J. Gastroenterol. 11, 4167–4172. doi: 10.3748/wjg.v11.i27.4167
Cheng, T., Yue, M., Aslam, M. N., Wang, X., Shekhawat, G., Varani, J., et al. (2017). Neuronal protein 3.1 deficiency leads to reduced cutaneous scar collagen deposition and tensile strength due to impaired transforming growth factor-beta1 to -beta3 translation. Am. J. Pathol. 187, 292–303. doi: 10.1016/j.ajpath.2016.10.004
Cooney, A. L., Singh, B. K., Loza, L. M., Thornell, I. M., Hippee, C. E., Powers, L. S., et al. (2018). Widespread airway distribution and short-term phenotypic correction of cystic fibrosis pigs following aerosol delivery of piggyBac/adenovirus. Nucleic Acids Res. doi: 10.1093/nar/gky773 [Epub ahead of print].
Darby, I. A., Laverdet, B., Bonte, F., and Desmouliere, A. (2014). Fibroblasts and myofibroblasts in wound healing. Clin. Cosmet. Investig. Dermatol. 7, 301–311.
Das, A., Mukherjee, P., Singla, S. K., Guturu, P., Frost, M. C., Mukhopadhyay, D., et al. (2010). Fabrication and characterization of an inorganic gold and silica nanoparticle mediated drug delivery system for nitric oxide. Nanotechnology 21:305102. doi: 10.1088/0957-4484/21/30/305102
Davies, L. A., Nunez-Alonso, G. A., McLachlan, G., Hyde, S. C., and Gill, D. R. (2014). Aerosol delivery of DNA/liposomes to the lung for cystic fibrosis gene therapy. Hum. Gene Ther. Clin. Dev. 25, 97–107. doi: 10.1089/humc.2014.019
Desmouliere, A., Chaponnier, C., and Gabbiani, G. (2005). Tissue repair, contraction, and the myofibroblast. Wound Rep. Regen. 13, 7–12. doi: 10.1111/j.1067-1927.2005.130102.x
Desmouliere, A., Redard, M., Darby, I., and Gabbiani, G. (1995). Apoptosis mediates the decrease in cellularity during the transition between granulation tissue and scar. Am. J. Pathol. 146, 56–66.
Dranoff, J. A., Ogawa, M., Kruglov, E. A., Gaca, M. D., Sevigny, J., Robson, S. C., et al. (2004). Expression of P2Y nucleotide receptors and ectonucleotidases in quiescent and activated rat hepatic stellate cells. Am. J. Physiol. Gastrointest. Liver Physiol. 287, G417–G424. doi: 10.1152/ajpgi.00294.2003
Du, S. L., Pan, H., Lu, W. Y., Wang, J., Wu, J., and Wang, J. Y. (2007). Cyclic Arg-Gly-Asp peptide-labeled liposomes for targeting drug therapy of hepatic fibrosis in rats. J. Pharmacol. Exp. Ther. 322, 560–568. doi: 10.1124/jpet.107.122481
Erhardt, J. A., and Pittman, R. N. (1998). Ectopic p21(WAF1) expression induces differentiation-specific cell cycle changes in PC12 cells characteristic of nerve growth factor treatment. J. Biol. Chem. 273, 23517–23523. doi: 10.1074/jbc.273.36.23517
Fernandez, I., Meneu, J. C., Colina, F., Garcia, I., Munoz, R., Castellano, G., et al. (2006). Clinical and histological efficacy of pegylated interferon and ribavirin therapy of recurrent hepatitis C after liver transplantation. Liver Transpl. 12, 1805–1812. doi: 10.1002/lt.20883
Friedman, S. L. (2008). Hepatic stellate cells: protean, multifunctional, and enigmatic cells of the liver. Physiol. Rev. 88, 125–172. doi: 10.1152/physrev.00013.2007
Friedman, S. L., Sheppard, D., Duffield, J. S., and Violette, S. (2013). Therapy for fibrotic diseases: nearing the starting line. Sci. Transl. Med. 5:167sr1. doi: 10.1126/scitranslmed.3004700
Fujitani, M., Yamagishi, S., Che, Y. H., Hata, K., Kubo, T., Ino, H., et al. (2004). P311 accelerates nerve regeneration of the axotomized facial nerve. J. Neurochem. 91, 737–744. doi: 10.1111/j.1471-4159.2004.02738.x
Gandhi, C. R. (2017). Hepatic stellate cell activation and pro-fibrogenic signals. J. Hepatol. 67, 1104–1105. doi: 10.1016/j.jhep.2017.06.001
Giacomini, M. M., Travis, M. A., Kudo, M., and Sheppard, D. (2012). Epithelial cells utilize cortical actin/myosin to activate latent TGF-beta through integrin alpha(v)beta(6)-dependent physical force. Exp. Cell Res. 318, 716–722. doi: 10.1016/j.yexcr.2012.01.020
Guimaraes, E. L., Stradiot, L., Mannaerts, I., Schroyen, B., and van Grunsven, L. A. (2015). P311 modulates hepatic stellate cells migration. Liver Int. 35, 1253–1264. doi: 10.1111/liv.12691
Gumel, A. M., Razaif-Mazinah, M. R., Anis, S. N., and Annuar, M. S. (2015). Poly (3-hydroxyalkanoates)-co-(6-hydroxyhexanoate) hydrogel promotes angiogenesis and collagen deposition during cutaneous wound healing in rats. Biomed. Mater. 10:045001. doi: 10.1088/1748-6041/10/4/045001
Hattori, T., Shimokawa, H., Higashi, M., Hiroki, J., Mukai, Y., Tsutsui, H., et al. (2004). Long-term inhibition of Rho-kinase suppresses left ventricular remodeling after myocardial infarction in mice. Circulation 109, 2234–2239. doi: 10.1161/01.CIR.0000127939.16111.58
Hawkins, R. D., Helms, C. A., Winston, J. B., Warchol, M. E., and Lovett, M. (2006). Applying genomics to the avian inner ear: development of subtractive cDNA resources for exploring sensory function and hair cell regeneration. Genomics 87, 801–808. doi: 10.1016/j.ygeno.2005.12.014
Heldin, C. H., Miyazono, K., and ten Dijke, P. (1997). TGF-beta signalling from cell membrane to nucleus through SMAD proteins. Nature 390, 465–471. doi: 10.1038/37284
Hirschi, K. K., Lai, L., Belaguli, N. S., Dean, D. A., Schwartz, R. J., and Zimmer, W. E. (2002). Transforming growth factor-beta induction of smooth muscle cell phenotpye requires transcriptional and post-transcriptional control of serum response factor. J. Biol. Chem. 277, 6287–6295. doi: 10.1074/jbc.M106649200
Huang, B., Lu, M., Jolly, M. K., Tsarfaty, I., Onuchic, J., and Ben-Jacob, E. (2014). The three-way switch operation of Rac1/RhoA GTPase-based circuit controlling amoeboid-hybrid-mesenchymal transition. Sci. Rep. 4:6449. doi: 10.1038/srep06449
Huang, H., Dai, H. P., Kang, J., Chen, B. Y., Sun, T. Y., and Xu, Z. J. (2015). Double-blind randomized trial of pirfenidone in chinese idiopathic pulmonary fibrosis patients. Medicine 94:e1600. doi: 10.1097/MD.0000000000001600
Huang, X., Yang, N., Fiore, V. F., Barker, T. H., Sun, Y., Morris, S. W., et al. (2012). Matrix stiffness-induced myofibroblast differentiation is mediated by intrinsic mechanotransduction. Am. J. Respir. Cell Mol. Biol. 47, 340–348. doi: 10.1165/rcmb.2012-0050OC
Huang, Y., Arora, P., McCulloch, C. A., and Vogel, W. F. (2009). The collagen receptor DDR1 regulates cell spreading and motility by associating with myosin IIA. J. Cell Sci. 122, 1637–1646. doi: 10.1242/jcs.046219
Ignotz, R. A., and Massague, J. (1987). Cell adhesion protein receptors as targets for transforming growth factor-beta action. Cell 51, 189–197. doi: 10.1016/0092-8674(87)90146-2
Ishiwatari, H., Sato, Y., Murase, K., Yoneda, A., Fujita, R., Nishita, H., et al. (2013). Treatment of pancreatic fibrosis with siRNA against a collagen-specific chaperone in vitamin A-coupled liposomes. Gut 62, 1328–1339. doi: 10.1136/gutjnl-2011-301746
Ivanova, V., Garbuzenko, O. B., Reuhl, K. R., Reimer, D. C., Pozharov, V. P., and Minko, T. (2013). Inhalation treatment of pulmonary fibrosis by liposomal prostaglandin E2. Eur. J. Pharm. Biopharm. 84, 335–344. doi: 10.1016/j.ejpb.2012.11.023
Jeffers, M., Rong, S., and Vande Woude, G. F. (1996). Enhanced tumorigenicity and invasion-metastasis by hepatocyte growth factor/scatter factor-met signalling in human cells concomitant with induction of the urokinase proteolysis network. Mol. Cell. Biol. 16, 1115–1125. doi: 10.1128/MCB.16.3.1115
Kajiwara, K., Sugaya, E., Kimura, M., Katsuki, M., Nagasawa, H., Yuyama, N., et al. (1995). Cloning and characterization of pentylenetetrazol-related cDNA, PTZ-17. Brain Res. 671, 170–174. doi: 10.1016/0006-8993(94)01308-5
Kisseleva, T., Cong, M., Paik, Y., Scholten, D., Jiang, C., Benner, C., et al. (2012). Myofibroblasts revert to an inactive phenotype during regression of liver fibrosis. Proc. Natl. Acad. Sci. U.S.A. 109, 9448–9453. doi: 10.1073/pnas.1201840109
Kuroda, S., Tashiro, H., Kimura, Y., Hirata, K., Tsutada, M., Mikuriya, Y., et al. (2015). Rho-kinase inhibitor targeting the liver prevents ischemia/reperfusion injury in the steatotic liver without major systemic adversity in rats. Liver Transpl. 21, 123–131. doi: 10.1002/lt.24020
Lacouture, M. E., Morris, J. C., Lawrence, D. P., Tan, A. R., Olencki, T. E., Shapiro, G. I., et al. (2015). Cutaneous keratoacanthomas/squamous cell carcinomas associated with neutralization of transforming growth factor beta by the monoclonal antibody fresolimumab (GC1008). Cancer Immunol. Immunother. 64, 437–446. doi: 10.1007/s00262-015-1653-0
Leask, A., and Abraham, D. J. (2004). TGF-beta signaling and the fibrotic response. FASEB J. 18, 816–827. doi: 10.1096/fj.03-1273rev
Lecker, S. H., Jagoe, R. T., Gilbert, A., Gomes, M., Baracos, V., Bailey, J., et al. (2004). Multiple types of skeletal muscle atrophy involve a common program of changes in gene expression. FASEB J. 18, 39–51. doi: 10.1096/fj.03-0610com
Li, F., Li, Q. H., Wang, J. Y., Zhan, C. Y., Xie, C., and Lu, W. Y. (2012). Effects of interferon-gamma liposomes targeted to platelet-derived growth factor receptor-beta on hepatic fibrosis in rats. J. Control. Release 159, 261–270. doi: 10.1016/j.jconrel.2011.12.023
Li, H., Cai, H., Deng, J., Tu, X., Sun, Y., Huang, Z., et al. (2018). TGF-beta-mediated upregulation of Sox9 in fibroblast promotes renal fibrosis. Biochim. Biophys. Acta 1864, 520–532. doi: 10.1016/j.bbadis.2017.11.011
Li, H., Yao, Z., He, W., Gao, H., Bai, Y., Yang, S., et al. (2016). P311 induces the transdifferentiation of epidermal stem cells to myofibroblast-like cells by stimulating transforming growth factor beta1 expression. Stem cell Res. Ther. 7:175. doi: 10.1186/s13287-016-0421-1
Li, Z., Dranoff, J. A., Chan, E. P., Uemura, M., Sevigny, J., and Wells, R. G. (2007). Transforming growth factor-beta and substrate stiffness regulate portal fibroblast activation in culture. Hepatology 46, 1246–1256. doi: 10.1002/hep.21792
Lindon, C., Montarras, D., and Pinset, C. (1998). Cell cycle-regulated expression of the muscle determination factor Myf5 in proliferating myoblasts. J. Cell Biol. 140, 111–118. doi: 10.1083/jcb.140.1.111
Liu, F., Mih, J. D., Shea, B. S., Kho, A. T., Sharif, A. S., Tager, A. M., et al. (2010). Feedback amplification of fibrosis through matrix stiffening and COX-2 suppression. J. Cell Biol. 190, 693–706. doi: 10.1083/jcb.201004082
Liu, S., Kapoor, M., Denton, C. P., Abraham, D. J., and Leask, A. (2009a). Loss of beta1 integrin in mouse fibroblasts results in resistance to skin scleroderma in a mouse model. Arthritis Rheum. 60, 2817–2821. doi: 10.1002/art.24801
Liu, S., Kapoor, M., and Leask, A. (2009b). Rac1 expression by fibroblasts is required for tissue repair in vivo. Am. J. Pathol. 174, 1847–1856. doi: 10.2353/ajpath.2009.080779
Liu, Z., Van Rossen, E., Timmermans, J. P., Geerts, A., van Grunsven, L. A., and Reynaert, H. (2011). Distinct roles for non-muscle myosin II isoforms in mouse hepatic stellate cells. J. Hepatol. 54, 132–141. doi: 10.1016/j.jhep.2010.06.020
Loirand, G., and Pacaud, P. (2010). The role of Rho protein signaling in hypertension. Nat. Rev. Cardiol. 7, 637–647. doi: 10.1038/nrcardio.2010.136
Luli, S., Di Paolo, D., Perri, P., Brignole, C., Hill, S. J., Brown, H., et al. (2016). A new fluorescence-based optical imaging method to non-invasively monitor hepatic myofibroblasts in vivo. J. Hepatol. 65, 75–83. doi: 10.1016/j.jhep.2016.03.021
Lyon, P. C., Griffiths, L. F., Lee, J., Chung, D., Carlisle, R., Wu, F., et al. (2017). Clinical trial protocol for TARDOX: a phase I study to investigate the feasibility of targeted release of lyso-thermosensitive liposomal doxorubicin (ThermoDox(R)) using focused ultrasound in patients with liver tumours. J. Ther. Ultrasound 5:28. doi: 10.1186/s40349-017-0104-0
Manosroi, A., Chankhampan, C., Manosroi, W., and Manosroi, J. (2013). Transdermal absorption enhancement of papain loaded in elastic niosomes incorporated in gel for scar treatment. Eur. J. Pharm. Sci. 48, 474–483. doi: 10.1016/j.ejps.2012.12.010
Mariani, L., McDonough, W. S., Hoelzinger, D. B., Beaudry, C., Kaczmarek, E., Coons, S. W., et al. (2001). Identification and validation of P311 as a glioblastoma invasion gene using laser capture microdissection. Cancer Res. 61, 4190–4196.
McDonough, W. S., Tran, N. L., and Berens, M. E. (2005). Regulation of glioma cell migration by serine-phosphorylated P311. Neoplasia 7, 862–872. doi: 10.1593/neo.05190
Miano, J. M., Long, X., and Fujiwara, K. (2007). Serum response factor: master regulator of the actin cytoskeleton and contractile apparatus. Am. J. Physiol. Cell Physiol. 292, C70–C81. doi: 10.1152/ajpcell.00386.2006
Morry, J., Ngamcherdtrakul, W., Gu, S., Goodyear, S. M., Castro, D. J., Reda, M. M., et al. (2015). Dermal delivery of HSP47 siRNA with NOX4-modulating mesoporous silica-based nanoparticles for treating fibrosis. Biomaterials 66, 41–52. doi: 10.1016/j.biomaterials.2015.07.005
Munger, J. S., Huang, X., Kawakatsu, H., Griffiths, M. J., Dalton, S. L., Wu, J., et al. (1999). The integrin alpha v beta 6 binds and activates latent TGF beta 1: a mechanism for regulating pulmonary inflammation and fibrosis. Cell 96, 319–328. doi: 10.1016/S0092-8674(00)80545-0
Nayak, B. K., Shanmugasundaram, K., Friedrichs, W. E., Cavaglierii, R. C., Patel, M., Barnes, J., et al. (2016). HIF-1 mediates renal fibrosis in OVE26 type 1 diabetic mice. Diabetes Metab. Res. Rev. 65, 1387–1397.
Nolte, A., Aufderklamm, S., Scheu, K., Walker, T., Konig, O., Bottcher, M., et al. (2013). Small interfering RNA transfection against serum response factor mediates growth inhibition of benign prostatic hyperplasia fibroblasts. Nucleic Acid Ther. 23, 62–70. doi: 10.1089/nat.2012.0392
Olsen, A. L., Bloomer, S. A., Chan, E. P., Gaca, M. D., Georges, P. C., Sackey, B., et al. (2011). Hepatic stellate cells require a stiff environment for myofibroblastic differentiation. Am. J. Physiol. Gastrointest. Liver Physiol. 301, G110–G118. doi: 10.1152/ajpgi.00412.2010
Olson, M. F., Paterson, H. F., and Marshall, C. J. (1998). Signals from Ras and Rho GTPases interact to regulate expression of p21Waf1/Cip1. Nature 394, 295–299. doi: 10.1038/28425
Ooi, P. T., da Costa, N., Edgar, J., and Chang, K. C. (2006). Porcine congenital splayleg is characterised by muscle fibre atrophy associated with relative rise in MAFbx and fall in P311 expression. BMC Vet. Res. 2:23. doi: 10.1186/1746-6148-2-23
Otsuka, M., Shiratori, M., Chiba, H., Kuronuma, K., Sato, Y., Niitsu, Y., et al. (2017). Treatment of pulmonary fibrosis with siRNA against a collagen-specific chaperone HSP47 in vitamin A-coupled liposomes. Exp. Lung Res. 43, 271–282. doi: 10.1080/01902148.2017.1354946
Paliwal, S., Shi, J., Dhru, U., Zhou, Y., and Schuger, L. (2004). P311 binds to the latency associated protein and downregulates the expression of TGF-beta1 and TGF-beta2. Biochem. Biophys. Res. Commun. 315, 1104–1109. doi: 10.1016/j.bbrc.2004.01.171
Pan, D., Zhe, X., Jakkaraju, S., Taylor, G. A., and Schuger, L. (2002). P311 induces a TGF-beta1-independent, nonfibrogenic myofibroblast phenotype. J. Clin. Invest. 110, 1349–1358. doi: 10.1172/JCI0215614
Piek, E., Ju, W. J., Heyer, J., Escalante-Alcalde, D., Stewart, C. L., Weinstein, M., et al. (2001). Functional characterization of transforming growth factor beta signaling in Smad2- and Smad3-deficient fibroblasts. J. Biol. Chem. 276, 19945–19953. doi: 10.1074/jbc.M102382200
Qi, F., Cai, P., Liu, X., Peng, M., and Si, G. (2015). Adenovirus-mediated P311 inhibits TGF-beta1-induced epithelial-mesenchymal transition in NRK-52E cells via TGF-beta1-Smad-ILK pathway. Biosci. Trends 9, 299–306. doi: 10.5582/bst.2015.01129
Reddy, E. M., Chettiar, S. T., Kaur, N., Ganeshkumar, R., Shepal, V., Shanbhag, N. C., et al. (2011). Dlxin-1, a member of MAGE family, inhibits cell proliferation, invasion and tumorigenicity of glioma stem cells. Cancer Gene Ther. 18, 206–218. doi: 10.1038/cgt.2010.71
Reetz, J., Genz, B., Meier, C., Kowtharapu, B.S., Timm, F., Vollmar, B., et al. (2013). Development of adenoviral delivery systems to target hepatic stellate cells in vivo. PLoS One 8:e67091. doi: 10.1371/journal.pone.0067091
Rice, L. M., Padilla, C. M., McLaughlin, S. R., Mathes, A., Ziemek, J., Goummih, S., et al. (2015). Fresolimumab treatment decreases biomarkers and improves clinical symptoms in systemic sclerosis patients. J. Clin. Invest. 125, 2795–2807. doi: 10.1172/JCI77958
Sanyal, A. J., Chalasani, N., Kowdley, K. V., McCullough, A., Diehl, A. M., Bass, N. M., et al. (2010). Pioglitazone, vitamin E, or placebo for nonalcoholic steatohepatitis. N. Engl. J. Med. 362, 1675–1685. doi: 10.1056/NEJMoa0907929
Sato, Y., Murase, K., Kato, J., Kobune, M., Sato, T., Kawano, Y., et al. (2008). Resolution of liver cirrhosis using vitamin A-coupled liposomes to deliver siRNA against a collagen-specific chaperone. Nat. Biotechnol. 26, 431–442. doi: 10.1038/nbt1396
Scholten, D., Reichart, D., Paik, Y. H., Lindert, J., Bhattacharya, J., Glass, C. K., et al. (2011). Migration of fibrocytes in fibrogenic liver injury. Am. J. Pathol. 179, 189–198. doi: 10.1016/j.ajpath.2011.03.049
Schon, H. T., Bartneck, M., Borkham-Kamphorst, E., Nattermann, J., Lammers, T., Tacke, F., et al. (2016). Pharmacological intervention in hepatic stellate cell activation and hepatic fibrosis. Front. Pharmacol. 7:33. doi: 10.3389/fphar.2016.00033
Schratt, G., Philippar, U., Berger, J., Schwarz, H., Heidenreich, O., and Nordheim, A. (2002). Serum response factor is crucial for actin cytoskeletal organization and focal adhesion assembly in embryonic stem cells. J. Cell Biol. 156, 737–750. doi: 10.1083/jcb.200106008
Schuppan, D., and Kim, Y. O. (2013). Evolving therapies for liver fibrosis. J. Clin. Invest. 123, 1887–1901. doi: 10.1172/JCI66028
Shaw, L. M., Rabinovitz, I., Wang, H. H., Toker, A., and Mercurio, A. M. (1997). Activation of phosphoinositide 3-OH kinase by the alpha6beta4 integrin promotes carcinoma invasion. Cell 91, 949–960. doi: 10.1016/S0092-8674(00)80486-9
Shi, J., Badri, K. R., Choudhury, R., and Schuger, L. (2006). P311-induced myofibroblasts exhibit ameboid-like migration through RalA activation. Exp. Cell Res. 312, 3432–3442. doi: 10.1016/j.yexcr.2006.07.016
Shimizu, Y., Dobashi, K., Iizuka, K., Horie, T., Suzuki, K., Tukagoshi, H., et al. (2001). Contribution of small GTPase Rho and its target protein rock in a murine model of lung fibrosis. Am. J. Respir. Crit. Care Med. 163, 210–217. doi: 10.1164/ajrccm.163.1.2001089
Siller-Lopez, F., Sandoval, A., Salgado, S., Salazar, A., Bueno, M., Garcia, J., et al. (2004). Treatment with human metalloproteinase-8 gene delivery ameliorates experimental rat liver cirrhosis. Gastroenterology 126, 1122–1133; discussion 949. doi: 10.1053/j.gastro.2003.12.045
Simone, T. M., Longmate, W. M., Law, B. K., and Higgins, P. J. (2015). Targeted inhibition of PAI-1 activity impairs epithelial migration and wound closure following cutaneous injury. Adv. Wound Care 4, 321–328. doi: 10.1089/wound.2014.0611
Singer, A. J., and Clark, R. A. (1999). Cutaneous wound healing. N. Engl. J. Med. 341, 738–746. doi: 10.1056/NEJM199909023411006
Soulez, M., Rouviere, C. G., Chafey, P., Hentzen, D., Vandromme, M., Lautredou, N., et al. (1996). Growth and differentiation of C2 myogenic cells are dependent on serum response factor. Mol. Cell. Biol. 16, 6065–6074. doi: 10.1128/MCB.16.11.6065
Studler, J. M., Glowinski, J., and Levi-Strauss, M. (1993). An abundant mRNA of the embryonic brain persists at a high level in cerebellum, hippocampus and olfactory bulb during adulthood. Eur. J. Neurosci. 5, 614–623. doi: 10.1111/j.1460-9568.1993.tb00527.x
Sun, G. (2017). Pro-regenerative hydrogel restores scarless skin during Cutaneous wound healing. Adv. Healthc. Mater. 6:1700659 doi: 10.1002/adhm.201700659
Sun, Y. G., Gao, Y. J., Zhao, Z. Q., Huang, B., Yin, J., Taylor, G. A., et al. (2008). Involvement of P311 in the affective, but not in the sensory component of pain. Mol. Pain 4:23. doi: 10.1186/1744-8069-4-23
Tan, J., Peng, X., Luo, G., Ma, B., Cao, C., He, W., et al. (2010). Investigating the role of P311 in the hypertrophic scar. PLoS One 5:e9995. doi: 10.1371/journal.pone.0009995
Tanaka, H., Yamashita, T., Asada, M., Mizutani, S., Yoshikawa, H., and Tohyama, M. (2002). Cytoplasmic p21(Cip1/WAF1) regulates neurite remodeling by inhibiting Rho-kinase activity. J. Cell Biol. 158, 321–329. doi: 10.1083/jcb.200202071
Tanaka, H., Yamashita, T., Yachi, K., Fujiwara, T., Yoshikawa, H., and Tohyama, M. (2004). Cytoplasmic p21(Cip1/WAF1) enhances axonal regeneration and functional recovery after spinal cord injury in rats. Neuroscience 127, 155–164. doi: 10.1016/j.neuroscience.2004.05.010
Taylor, G. A., Hudson, E., Resau, J. H., and Vande Woude, G. F. (2000). Regulation of P311 expression by Met-hepatocyte growth factor/scatter factor and the ubiquitin/proteasome system. J. Biol. Chem. 275, 4215–4219. doi: 10.1074/jbc.275.6.4215
Taylor, G. A., Rodriguiz, R. M., Greene, R. I., Daniell, X., Henry, S. C., Crooks, K. R., et al. (2008). Behavioral characterization of P311 knockout mice. Genes Brain Behav. 7, 786–795. doi: 10.1111/j.1601-183X.2008.00420.x
Togami, K., Miyao, A., Miyakoshi, K., Kanehira, Y., Tada, H., and Chono, S. (2015). Efficient delivery to human lung fibroblasts (WI-38) of pirfenidone incorporated into liposomes modified with truncated basic fibroblast growth factor and its inhibitory effect on collagen synthesis in idiopathic pulmonary fibrosis. Biol. Pharm. Bull. 38, 270–276. doi: 10.1248/bpb.b14-00659
Ueda, K. (2001). Detection of the retinoic acid-regulated genes in a RTBM1 neuroblastoma cell line using cDNA microarray. Kurume Med. J. 48, 159–164. doi: 10.2739/kurumemedj.48.159
van Beuge, M. M., Prakash, J., Lacombe, M., Post, E., Reker-Smit, C., Beljaars, L., et al. (2013). Enhanced effectivity of an ALK5-inhibitor after cell-specific delivery to hepatic stellate cells in mice with liver injury. PLoS One 8:e56442. doi: 10.1371/journal.pone.0056442
van Grunsven, L. A., Billon, N., Savatier, P., Thomas, A., Urdiales, J. L., and Rudkin, B. B. (1996). Effect of nerve growth factor on the expression of cell cycle regulatory proteins in PC12 cells: dissection of the neurotrophic response from the anti-mitogenic response. Oncogene 12, 1347–1356.
Wang, A., Yokosaki, Y., Ferrando, R., Balmes, J., and Sheppard, D. (1996). Differential regulation of airway epithelial integrins by growth factors. Am. J. Respir. Cell Mol. Biol. 15, 664–672. doi: 10.1165/ajrcmb.15.5.8918373
Wang, F., Xie, X., Fan, J., Wang, L., Guo, D., Yang, L., et al. (2010). Expression of P311, a transforming growth factor beta latency-associated protein-binding protein, in human kidneys with IgA nephropathy. Int. Urol. Nephrol. 42, 811–819. doi: 10.1007/s11255-009-9681-3
Wang, P., Koyama, Y., Liu, X., Xu, J., Ma, H. Y., Liang, S., et al. (2016). Promising therapy candidates for liver fibrosis. Front. Physiol. 7 47. doi: 10.3389/fphys.2016.00047
Yamashita, T., Tucker, K. L., and Barde, Y. A. (1999). Neurotrophin binding to the p75 receptor modulates Rho activity and axonal outgrowth. Neuron 24, 585–593. doi: 10.1016/S0896-6273(00)81114-9
Yao, Z., Li, H., He, W., Yang, S., Zhang, X., Zhan, R., et al. (2017). P311 accelerates skin wound reepithelialization by promoting epidermal stem cell migration through RhoA and Rac1 activation. Stem Cells Dev. 26, 451–460. doi: 10.1089/scd.2016.0249
Yao, Z., Yang, S., He, W., Li, L., Xu, R., Zhang, X., et al. (2015). P311 promotes renal fibrosis via TGFbeta1/Smad signaling. Sci. Rep. 5:17032. doi: 10.1038/srep17032
Yue, M. M., Lv, K., Meredith, S. C., Martindale, J. L., Gorospe, M., and Schuger, L. (2014). Novel RNA-binding protein P311 binds eukaryotic translation initiation factor 3 subunit b (eIF3b) to promote translation of transforming growth factor beta1-3 (TGF-beta1-3). J. Biol. Chem. 289, 33971–33983. doi: 10.1074/jbc.M114.609495
Zhan, S. S., Jiang, J. X., Wu, J., Halsted, C., Friedman, S. L., Zern, M. A., et al. (2006). Phagocytosis of apoptotic bodies by hepatic stellate cells induces NADPH oxidase and is associated with liver fibrosis in vivo. Hepatology 43, 435–443. doi: 10.1002/hep.21093
Zhang, S. H., Wen, K. M., Wu, W., Li, W. Y., and Zhao, J. N. (2013). Efficacy of HGF carried by ultrasound microbubble-cationic nano-liposomes complex for treating hepatic fibrosis in a bile duct ligation rat model, and its relationship with the diffusion-weighted MRI parameters. Clin. Res. Hepatol. Gastroenterol. 37, 602–607. doi: 10.1016/j.clinre.2013.05.011
Keywords: P311, fibrosis, proliferation, migration, TGFβ1
Citation: Stradiot L, Mannaerts I and van Grunsven LA (2018) P311, Friend, or Foe of Tissue Fibrosis? Front. Pharmacol. 9:1151. doi: 10.3389/fphar.2018.01151
Received: 27 July 2018; Accepted: 24 September 2018;
Published: 12 October 2018.
Edited by:
Cecilia Battistelli, Università degli Studi di Roma La Sapienza, ItalyReviewed by:
Martina Schmidt, University of Groningen, NetherlandsCopyright © 2018 Stradiot, Mannaerts and van Grunsven. This is an open-access article distributed under the terms of the Creative Commons Attribution License (CC BY). The use, distribution or reproduction in other forums is permitted, provided the original author(s) and the copyright owner are credited and that the original publication in this journal is cited, in accordance with accepted academic practice. No use, distribution or reproduction is permitted which does not comply with these terms.
*Correspondence: Leo A. van Grunsven, TGVvLnZhbi5HcnVuc3ZlbkB2dWIuYWMuYmU=
Disclaimer: All claims expressed in this article are solely those of the authors and do not necessarily represent those of their affiliated organizations, or those of the publisher, the editors and the reviewers. Any product that may be evaluated in this article or claim that may be made by its manufacturer is not guaranteed or endorsed by the publisher.
Research integrity at Frontiers
Learn more about the work of our research integrity team to safeguard the quality of each article we publish.