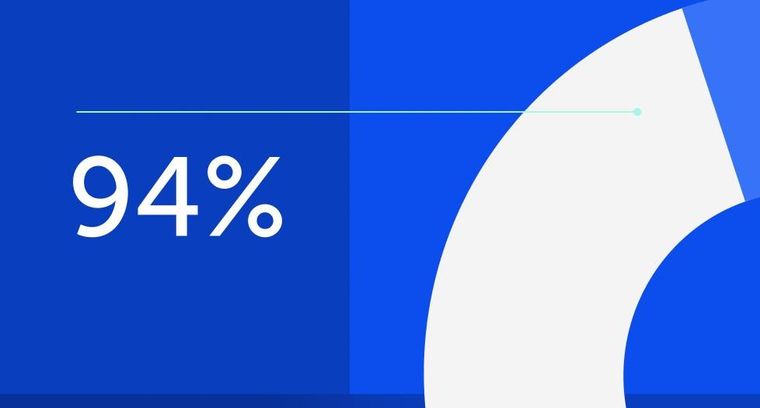
94% of researchers rate our articles as excellent or good
Learn more about the work of our research integrity team to safeguard the quality of each article we publish.
Find out more
REVIEW article
Front. Oncol., 28 July 2020
Sec. Radiation Oncology
Volume 10 - 2020 | https://doi.org/10.3389/fonc.2020.01107
This article is part of the Research TopicNew Approaches to Breast Cancer RadiotherapyView all 6 articles
Current research in radiotherapy (RT) for breast cancer is evaluating neoadjuvant as opposed to adjuvant partial breast irradiation (PBI) with the aim of reducing the volume of breast tissue irradiated and therefore the risk of late treatment-related toxicity. The development of magnetic resonance (MR)–guided RT, including dedicated MR-guided RT systems [hybrid machines combining an MR scanner with a linear accelerator (MR-linac) or 60Co sources], could potentially reduce the irradiated volume even further by improving tumour visibility before and during each RT treatment. In this position paper, we discuss MR guidance in relation to each step of the breast RT planning and treatment pathway, focusing on the application of MR-guided RT to neoadjuvant PBI.
The combination of a worldwide rising incidence of breast cancer together with decreasing mortality following breast cancer treatment has resulted in increasing numbers of breast cancer survivors living with late treatment-related toxicity (1–3). In recent decades, this has led to prioritization of treatment de-escalation aiming to reduce treatment-related toxicity without impeding survival (4). Studies comparing adjuvant whole breast irradiation (WBI) vs. adjuvant partial breast irradiation (PBI) in women with lower-risk breast cancers have demonstrated that PBI is as effective as WBI in terms of 5-years local recurrence rates and survival but with lower rates of late patient-reported and clinician-reported toxicity (5–8). Nonetheless, late treatment-related toxicity remains an issue in a significant proportion of patients (6, 8).
With neoadjuvant PBI, smaller target volumes can be irradiated compared to conventional adjuvant PBI, potentially resulting in less radiotherapy (RT)–related toxicity and therefore a higher quality of life (9–11). This is because, for neoadjuvant PBI, the gross target volume (GTV) is tumour rather than tumour bed, presenting a smaller, more easily definable target. Furthermore, the breast tissue at risk of local relapse remains in the closest possible proximity to the GTV, thereby reducing uncertainty around location of the clinical target volume (CTV). This is increasingly important in the current era of oncoplastic surgery in which the tissue that was adjacent to the tumour, the edge of which is usually marked by titanium surgical clips, may be mobilized and placed at some distance from its original location in order to ensure a good cosmetic result. This can lead to a larger CTV in the adjuvant setting than would have been necessary in the neoadjuvant setting. One problem with irradiating tumours in the neoadjuvant setting using the current standard computed tomography (CT)–based RT planning pathway, however, is that primary breast cancers can be difficult to see on a standard non–contrast-enhanced RT planning CT scan.
The development of magnetic resonance (MR)–guided RT has greatly improved the possibilities for image-guided RT and greater sparing of healthy tissue by providing excellent soft tissue visualization. MR–guided RT can refer to treatment on a conventional linear accelerator (linac) with the use of additional imaging on an MR scanner to plan treatment or to treatment on a hybrid machine. A hybrid machine is an MR scanner combined with a linac (MR-linac, Unity Elekta and MRIdian linac, ViewRay) or with 60Co sources (MRIdian, ViewRay) (12–15). For breast cancer patients, MR-guided RT is expected to be most beneficial in the neoadjuvant setting treating in situ tumours, which can be more clearly visualized on MR images than on CT, both at the time of RT planning and during RT treatment. The latter would facilitate reduction in setup error margins in both the neoadjuvant and adjuvant setting. In addition, administering MR-guided RT on a hybrid machine could reduce the radiation exposure associated with the daily cone-beam CT (CBCT) required during treatment on a conventional linac.
In this position paper, we discuss MR guidance in relation to each step of the breast RT planning and treatment pathway from simulation to contouring, to treatment planning, and then delivery. We review what is already known, what is under evaluation, and potential obstacles to clinical implementation, highlighting where optimization of techniques and/or workflow is still required (Table 1).
Table 1. Overview of challenges for the implementation of MR-guided radiotherapy on a hybrid machine for breast cancer patients.
The main challenge for patient setup in treatment position for breast RT in a magnetic resonance imaging (MRI) scanner or a hybrid machine is the limited MRI bore size (60–70 cm) compared to the CT bore size of 80 to 90 cm (16–18). This limits the size and inclination of a positioning device, as well as the number of possible positions for patient setup.
For patients treated in supine position with arms raised above their head, the elbow span in combination with an inclined position can be problematic. A solution for this is to put the arms closer together and/or to use either a wedge with smaller inclination or no wedge at all. Placement of an anterior receiver coil on a patient in supine position could lead to deformation of the breast (19). However, coil bridges can be used as support for the coil to prevent deformation (Figure 1) (9, 20–22).
Figure 1. Supine patient setup for MRI simulation. In this setup, a 5-degree inclined wedge is used. Height-adjustable coil bridges are used as support for the anterior receiver coil to prevent deformation of the body contour.
In the prone position, the proportion of patients who can fit into the MR scanner bore is limited by the space needed for a pendulous breast to hang freely without touching the table top in combination with the requirement to place an additional receiver coil on the back of the patient (Figure 2). The additional receiver coil is necessary as the full body contour is needed for RT planning purposes, which is not a requirement for diagnostic prone breast imaging.
Figure 2. Patient and receiver coil positioning in prone position, including challenges in this position. The images show three different patients. (A) No space for the receiver coil on the back of the patient if the breast hangs freely without touching the scanner table; (B) the receiver coil fits above the patient while also the breast hangs freely; (C) when the receiver coil is fitted in the MRI bore above the patient, the breast touches the table top and is deformed. Light blue shapes represent the receiver coils (horizontal: receiver coil array; vertical: single flex coil). SNR, signal-to-noise ratio.
Standard RT immobilization equipment may not necessarily be MR-compatible, and standard MR equipment (e.g., the dedicated prone breast coil) is not designed for setup reproducibility. Therefore, it is necessary to develop dedicated RT immobilization equipment that is MR-compatible (i.e., non-conductive, low-density material). This equipment must also fit inside the MR bore and leave room for the MR receiver coils (e.g., flexible receiver coils in a prone breast board), while not degrading image quality (17, 22). Because of the electron stream effect (ESE), further discussed in Treatment Planning for a Hybrid Machine, simulation should include the chin and upper abdominal region.
For optimal quality of MR images, the receiver coil should be placed close to the target volume. Therefore, a strategic setup for the additional coils should be chosen, specific to the selected patient position (e.g., supine or prone). Because RT immobilization devices, such as the supine and prone breast boards and coil bridges, increase the gap between the patient and the receiver coils (i.e., the distance to the posterior coil located in the scanner table and to the anterior coil on top of the patient), it was initially thought that the positioning requirements for breast cancer RT might have a negative impact on MR image quality. However, multiple studies have reported good quality of MR images for breast RT in both supine and prone treatment positions acquired at 1.5- and 3.0-T MR scanners (19, 21, 22).
Another factor that might impair MR image quality is organ motion, including respiratory and cardiac motion, during scanning. Imaging in prone position has the advantage of minimizing breast motion due to respiration and may also minimize motion artefacts (19). Batumalai et al. (22) found no significant effect of the breathing artefacts on image quality in both prone and supine position by instructing their volunteers to maintain shallow breathing and choosing a right–left phase encoding direction in their MRI scans. Additionally, to preventing the motion, artefact reduction (e.g., gating or triggering) or motion correction (e.g., MR navigators) techniques can be used to minimize motion effects on MRI scans. However, it is important to realize how the anatomy relates to the breathing state during RT (18). To prevent step-like displacements in different slices in the scan volume caused by motion during scanning, a three-dimensional (3D) sequence can be used, although motion in a 3D scan will lead to blurring (23).
In studies that evaluated prone breast MRI for RT, a dedicated breast coil is usually used (19, 22, 24). While this coil provides optimal image quality for the breasts, it cannot capture the full body contour and all organs at risk (OARs) with adequate quality (Figure 2). However, for MR-guided RT on a conventional linac, this may be sufficient, provided that enough anatomical landmarks are visible to register the MR scan to the planning CT scan. Scanning with an additional receiver coil on top of the patient could help to overcome this issue, but may not be possible in all patients because of the limited MR bore size.
In case of RT treatment on a hybrid MR-guided RT system, it is not possible to irradiate through the standard dedicated prone breast coils that are used in diagnostic MRI. For that reason, the receiver coils dedicated to hybrid machines have a “window” through which irradiation is possible (15, 25). Because these dedicated coils have different properties to the standard receiver coils (i.e., fewer coil arrays, which restricts acceleration of imaging) and are not breast specific, the image quality can be inferior. Another restriction is that the coil cannot be placed too closely to the patient because of the electron return effect (ERE; see Treatment Planning for a Hybrid Machine), which restricts the signal-to-noise ratio of the imaging. In general, a higher field strength gives a better signal-to-noise ratio, which may place a 1.5-T hybrid system in favour over a 0.35-T system. However, experiences with the 0.35-T hybrid system show that patient setup and online tracking for breast cancer could be performed successfully based on imaging at this lower magnetic field strength (26). To ensure appropriate image quality, the MRI sequences and image quality for breast imaging on the hybrid systems should therefore be tested and optimized for the use of the dedicated coil and each system specifically.
The impact of geometric distortions on MR-based contouring and planning should be taken into account when optimizing image quality and MRI sequences for RT on a hybrid machine (18, 27). The effect of distortions on image quality is described in this section, whereas the effect of distortions on dose distributions is described in Treatment Planning for a Hybrid Machine.
Distortions arise from system-related factors (i.e., main magnetic field inhomogeneity and gradient nonlinearities) and patient-related factors (i.e., chemical shift and susceptibility effects) and depend on the specific scanner and sequence parameters (18, 28–31).
System-related distortions due to gradient non-linearities increase with increasing distance of the target volume from the MRI isocenter and can range up to 12 mm (25, 27, 28, 30, 32). For the Elekta MR-linac (1.5 T), maximum displacements of 2.0 mm were found within 17.5 cm from the isocenter (25). For the ViewRay 60Co-system (0.35 T), this was 1.9 mm, but larger distortions were observed further from the central axis (33). To minimize the effect of image distortion by gradient non-linearities, the target volume should be positioned as close to the scanner isocenter as possible (17), which may be challenging for laterally located target volumes, such as lateral breast tumours. A possible solution may be to shift the patient on the scanner table toward the contralateral side such that the ipsilateral breast moves closer to the machine isocenter, if this is possible within the limited space inside the bore. Furthermore, to minimize system-related distortions, it is also important to always use the scanner's software for gradient non-linearity correction (23, 30). By using a 3D scan, the gradient non-linearity correction can be applied in all directions.
Distortions caused by main magnetic field inhomogeneities and by susceptibility effects induced by the patient's presence in the scanner also need to be corrected for. Distortion caused by patient-induced susceptibility can be particularly large, especially at the tissue–air interface, with mean maximum distortions at 3.0 T having been found to increase from 1.4 to 3.7 mm in a phantom to 3.7 to 11.3 mm in patients (including setup uncertainties) (29). Susceptibility effects scale with the main magnetic field strength (31). A lower field strength or a high receiver bandwidth can help to reduce both main magnetic field inhomogeneity and patient-induced susceptibility, but reduces signal-to-noise ratio (30, 31). Patient-specific correction methods (e.g., using the B0 map) may be helpful to correct for these distortions (18, 30).
Several MRI sequences have been recommended for MR-guided RT. For use of MRI in the adjuvant breast RT setting, use of T1-weighted 3D sequences without fat suppression resulted in the best visualization of surgical clips, whereas T1-weighted images with fat suppression (e.g., mDixon) best enabled differentiation between glandular breast tissue and seroma (9, 20, 34). Two-dimensional or 3D T2-weighted MRI with fat suppression [e.g., Short inversion time inversion recovery (STIR) or water selective excitation] or without fat suppression was preferred for visualization of lumpectomy cavity and associated seroma and for discrimination between glandular breast tissue and tumour bed (17, 19, 21, 22, 24).
In the neoadjuvant setting, the use of T1-weighted fat-suppressed contrast-enhanced MRI is recommended for optimal tumour and tumour spiculae visualization, because differences in contrast uptake provide a clear distinction between tumour and glandular breast tissue (Figure 3) (35–38). Additionally, T2-weighted images might aid in the differentiation between tumour and postbiopsy changes (35). mDixon fat suppression methods proved to be reliable and are recommended because they are relatively insensitive to main magnetic field inhomogeneities (39, 40). Use of diffusion-weighted imaging (DWI) was described in only one study, where it was used in the context of response evaluation after RT and not for target delineation (35). Use of DWI for RT could help in differentiation between benign and malignant lesions, but magnetic susceptibility-induced geometric distortions make it more suitable for diagnostic imaging than for MR-guided RT (41, 42). All studies presented above used fusion of MRI with a planning CT scan on which the OARs were delineated. Therefore, no recommendations focusing on OAR visualization on different MRI sequences have been published. Based on expert opinion, OARs are clearly visualized on any of the sequences mentioned above, except for DWI. All sequences described were acquired on stand-alone MRI scanners. Hybrid treatment machines may come with only a fixed set of available MRI sequences in clinical mode (15, 43). Therefore, not all sequences described may be available on these machines during treatment. A summary of online available MRI sequences on hybrid machines is presented in Table 2.
Figure 3. Imaging of a primary breast tumour on CT (A,D), (contrast-enhanced) MRI (B,E), and CBCT (C,F) scans indicating the difference in tumour visibility (inside the red circle) between these modalities in two different patients (A–C and D–F). (D–F) The marker inserted in the tumour medial in the left breast is observed as a void on MRI (indicated by the red circles).
Table 2. Overview of recommended MR sequences and commercial online availability for clinical breast cancer treatment on hybrid machines.
With regard to target volume delineation in the adjuvant PBI setting, delineation of the tumour bed on CT should, according to guidelines, include visible seroma and representative surgical clips and the tumour location on preoperative imaging and take into account the microscopic tumour free margins (44–47). The added value of MRI to a standard planning CT scan for delineation in the adjuvant setting is disputed for several reasons (48, 49). First, surgical clips lead to voids on MRI, potentially leading to less accurate target volume definition (34). Second, studies have shown both a significant increase as well as a decrease in the target volume when either a preoperative or postoperative MRI scan was available for delineation in addition to a postoperative planning CT (20, 21, 34, 50). Third, in three separate studies, MRI did not lead to a reduction in interobserver variation (20, 24, 50). However, in a more recent larger study, a significant reduction in interobserver variation was reported for delineation on MRI in patients without surgical clips (51). Therefore, the added value of using MRI for contouring in the adjuvant setting seems likely to be limited to those patients in whom tumour bed clips have not been placed.
In the context of neoadjuvant PBI, given that this is not yet a standard of care in breast cancer management, delineation of in situ breast tumours is a relatively new concept to most radiation oncologists, and new guidelines are needed. Guidelines for the delineation of primary breast tumours on MRI for use in neoadjuvant PBI setting have recently been developed by the Breast Tumor Site Group of the International MR-Linac Atlantic Consortium (36). These recommend the use of contrast-enhanced MRI, which, because of increased contrast uptake in tumours compared to the surrounding glandular breast tissue, allows for better visualization of breast tumours than using CT (Figure 3) (9, 38). Contrast-enhanced MRI has been used for the delineation of target volumes in several recent studies of neoadjuvant PBI (37, 52). In these studies, insertion of an additional fiducial marker by a radiologist was necessary both to help localize the tumour for subsequent surgical resection in case of tumour downstaging and for tumour position verification because the tumour cannot be visualized on CBCT in most patients. These markers cause artefacts on MRI, which can be observed as voids (Figure 3). The size of these artefacts depends on the material and geometry of the marker. As the artefact can obscure tumour tissue, the void of a marker should be included in the target volume. If omission of surgery after an ablative dose RT becomes clinically feasible, insertion of a fiducial marker in the tumour might not be necessary anymore. This would be beneficial for both target volume definition and follow-up imaging, as well as patient satisfaction (53).
For MR-guided RT on a conventional linac, treatment planning is performed according to the standard practice. This includes registering the MRI scan to the planning CT scan used for delineation and producing a dose distribution using a standard treatment planning system. However, when treatment is to be delivered on a MR-guided hybrid machine, several additional factors need to be considered, all of which will be incorporated into the dedicated treatment planning systems. These factors are inherently related to the design of the hybrid machines. First, given that the magnetic field influences the path of secondary electrons, the ERE and the ESE in air have to be taken into account. Second, the influence of geometric accuracy of the MR images on treatment planning must be considered. Third, there are some restrictions for planning to bear in mind.
The Lorentz force acting on moving charged particles in a magnetic field causes several effects during irradiation in a magnetic field (54–59). One of these is the ERE, which refers to the fact that the path of electrons is bent in the presence of a magnetic field, resulting in exit electrons re-entering the body after a helical path in air (55). Studies have shown that skin dose is increased for patients undergoing WBI in a magnetic field due to the ERE (60, 61). According to van Heijst et al. (60), the mean skin dose increased from 29.5 Gy at 0 T to 32.3 Gy at 0.35 T and to 33.2 Gy at 1.5 T for 2-beam WBI. For 7-beam WBI, the mean skin dose increased from 27.9 Gy at 0 T to 30.2 Gy at 0.35 T and to 29.8 Gy at 1.5 T. Given these findings, WBI is not thought to be a good indication for treatment on a hybrid machine, irrespective of the field strength. Although van Heijst et al. found that the mean skin dose for PBI also increased, from 5.2 Gy at 0 T to 5.6 Gy at 0.35 T and 5.8 Gy at 1.5 T, the absolute mean skin dose was small compared to WBI. Therefore, the increase in skin dose for PBI in a magnetic field would be highly unlikely to translate into a higher risk of radiation dermatitis. Furthermore, it has been reported that increasing the number of beam angles helps in decreasing the skin dose (60, 62). Therefore, although PBI is a good indication for breast RT on a hybrid machine, one should remain aware of the risk of increased skin dose and use more rather than fewer beams. Because the ERE effect is also present at the lung–tissue interface, it is also important to check the maximum lung and chest wall dose (57, 62). Previous planning studies concluded that the effects of the magnetic field on OARs, other than the skin, are generally negligible, and doses were within clinical constraints (60, 62, 63).
The second effect that should be kept in mind for breast cancer treatment on a hybrid machine is the ESE in air, which can lead to dose being deposited in tissues well outside the irradiated field (Figure 4). This was first observed and evaluated by Park et al. (64), who, in the context of accelerated PBI delivered on the 0.35 T 60Co ViewRay system, observed an electron stream in air extending toward the head and ipsilateral arm. This ESE is caused by electrons generated inside the body that, instead of scattering in random directions when leaving the body, start spiraling along the magnetic field (65). If unobstructed, this electron stream would reach the chin and arm, causing unwanted irradiation of the skin in these areas. In an extreme case, the maximum dose measured was as high as 16.1% of the prescribed dose (64). Dose to the skin outside the treatment field was highest in patients with tumours located in the cranial part of the breast. Depending on the location of the high-dose region in the breast, this electron stream can also be directed toward the feet (Figure 4). Studies on phantoms and early clinical experiences suggest that the treatment planning system is able to fully describe the ESE and that the use of bolus material to shield the body parts located in the electron stream showed effective reduction of the dose in these regions (64–66).
Figure 4. Simulation of a single fraction neoadjuvant PBI treatment plan (ABLATIVE trial approach, 1 × 20 Gy to GTV) for the 1.5-T MR-linac. The calculated dose distribution shows the electron stream effect in air resulting in dose outside of the treatment field in both cranial and caudal directions. Scale is set to 100% reference dose = 20 Gy.
Because the breast is located peripherally in the body and geometric distortions increase with distance from the isocenter and susceptibility effects arise near tissue–air interfaces (as described in Simulation), the effects of these distortions on dosimetry for breast RT may be significant (27, 30). The system-specific distortions together with patient-related distortions may result in unacceptable dosimetric variations, as has already been shown for WBI (29). This issue still requires investigation in the context of PBI, such as investigation of the impact of distortion at the edges of the breasts, which would lead to inaccurate assignment of air vs. tissue electron density and therefore inaccurate dose calculations when these are based on the MRI. Geometric distortions inside the target region should be carefully considered in choosing adequate planning target volume (PTV) margins in the context of breast RT on an hybrid machine (33).
Technical specifications such as the magnetic field strength, beam energy, source-to-axis distance, and maximum field size are system-specific and are accounted for in the treatment planning systems (13–15). However, there are some specific issues to highlight that will be different from treatment planning for breast irradiation on a conventional linac. First, for the ViewRay MR-linac system angles between 30 and 33° are not available, whereas for the Elekta system 8 to 18° degrees need to be avoided because of the cryostat pipe (15, 67). Furthermore, some beam angles commonly used for breast RT on conventional systems should preferably not be used on the Elekta system, that is, angles around 130–150° and 210–230°, with exact angles depending on the tumour location (66, 67). This is because of high-density material in the treatment couch edges that may cause unwanted dose effects during daily plan adaptation. Because of the design of the hybrid machines, rotations of the table with respect to the gantry angle and therefore irradiation with non-coplanar beams are not possible. No problems are expected because of this because good plan quality for PBI can be achieved with coplanar IMRT (26, 63, 68).
With respect to the methods currently used for dose calculation, co-registration of the planning CT to the pre-treatment and/or online MR images or bulk density assignment are currently used for electron density information for both the ViewRay and Elekta hybrid machines (15, 67, 69). Strategies for creating a synthetic CT directly from an MRI scan, such as atlas-based, voxel intensity–based, or deep learning approaches, are in development (70, 71). However, data on the use of synthetic CT for the breast or thoracic region are limited. Recent data have shown encouraging results for synthetic CT generation for the thoracic region based on (a combination of) voxel intensity– and atlas-based approaches, with a mean absolute error <50 HU in the body and dosimetric differences ≤ 1.7% inside lung tumour PTVs (72, 73). Inclusion of bone density information, specifically the spine in this study on lung tumour treatment plans, proved to be important to reduce local hot spots in the differences between the simulated dose distributions on CT and synthetic CT (73). Ahunbay et al. (74) proposed to continue using a planning CT scan for each patient. Their approach with inclusion of bone density and the use of deformably registered lung density, both of which may be necessary for breast RT treatment planning as well, may enable accurate full online replanning on the daily anatomy. In an online workflow, options may be limited by the specific system, but aforementioned issues should be taken into account, as well as speed of synthetic CT generation.
For MR-guided RT on a conventional linac, the treatment and position verification can be performed according to the current standard RT workflow. Using a hybrid machine with daily online MRI both before and during treatment, new opportunities become available for daily setup and positioning accuracy, online adaptive RT based on daily anatomy, and intrafraction motion management.
Experiences from hospitals that have treated breast cancer patients in the adjuvant setting with the 0.35 T 60Co system have shown that initial patient setup verification based on location of lumpectomy cavity and online motion monitoring could be beneficial for PBI patients in terms of reducing the CTV to PTV margin and therefore irradiated volume and thereby the risk of late toxicity (26, 43, 69, 75). A >52% reduction in treatment volume was achieved by applying no PTV margin for the lumpectomy cavity with the help of online MRI for setup (26, 75). Although a 0-mm PTV margin neglects correction of other uncertainties that would normally be incorporated in the CTV to PTV margin (e.g., mechanical equipment and dosimetric uncertainties) (76), this illustrates that online MRI for setup may help to reduce the PTV margin compared to treatment on a conventional linac. With the aid of an online motion monitoring approach, a mean difference of <1% between planned and delivered dose to 95% of the target volume was achieved (26). For treatment in the neoadjuvant setting, patient setup and positioning accuracy on a hybrid machine are still to be evaluated.
On hybrid machines, a new treatment plan can be made during each fraction based on online MRI. Depending on the specific system, different strategies are available. These range from dose recalculation on the new patient anatomy to full online recontouring and replanning (15, 77, 78). Requirements for online replanning are somewhat different than for pretreatment planning. In particular, the time available for target and OAR redelineation and plan optimization is much reduced because the patient is on the treatment table. The choice of plan adaptation strategy will therefore depend on a trade-off between plan quality and speed of plan adaptation. In general, it is expected that a full reoptimization plan adaptation method will lead to improved dosimetry in most patients, especially in the case of deformations in the tumour or OARs, but will take more time (78, 79). In the group reporting on adjuvant PBI on a 60Co system, where online MRI proved beneficial for setup and PTV margin reduction, no online plan adaptation was performed, and yet retrospective comparison of planned vs. delivered dose showed adequate coverage, suggesting that, in the context of PBI, use of a simpler plan adaptation strategy may be reasonable (26, 43). Currently, injection of contrast agent is not performed during treatment on a hybrid machine, although it could help to recontour the tumour volume in case of neoadjuvant PBI. However, gadolinium chelates, the most commonly used contrast agent for breast cancer, could have a radiosensitizing effect (80). Because of the uncertainty of the effect and safety of irradiation when a contrast agent has been injected and concern about stability and toxicity of irradiated gadolinium, it is not recommended to use contrast-enhanced sequences for imaging during treatment.
Generally three types of intrafraction motion can be distinguished: (1) regular breathing motion, (2) irregular transient motion, and (3) non-transient bulk motion. Breast intrafraction motion evaluated on 2D and 3D MR images (2- to 20-minutes duration) has been reported to be generally regular and limited to <3 mm (26, 81). Larger displacements have been observed, but these were mostly transient. Acharya et al. (26) calculated that a mean PTV margin of 0.7 mm would be sufficient to cover 90% of the lumpectomy cavity for 90% of the treatment time for a mean fraction duration of 12.7 minutes. However, intrafraction displacement seemed to differ substantially between patients, reaching a mean displacement range of 6 mm in anterior-posterior direction for one patient. One possibility to handle intrafraction displacement might be to individualize the PTV margin based on cine MR data from simulation. Larger whole-body shifts of up to 14 mm over a 21-minutes duration have been observed infrequently, although for the majority of patients motion evaluated up to 20 minutes was generally regular and small (81). The impact of intrafraction motion on current standard hypofractionated treatment is therefore likely to be limited. However, for extremely hypofractionated treatment schedules (one to two fractions) delivered on hybrid machines, treatment times will increase significantly because of the online delineation and planning procedure and because of increased beam on time because of a lower dose rate of the hybrid machines and use of IMRT compared to volumetric modulated arc therapy (68, 82, 83). This will increase the risk of systematic non-transient patient displacement both before and during treatment and may also negatively affect patient comfort. Although not yet available, real-time plan adaptation during RT delivery will be the ultimate goal to account for intrafraction motion management (84). Henke et al. (43) noted that online motion tracking and gating on the lumpectomy cavity were beneficial for accelerated PBI treatment with regard to reduction of the PTV margin (26, 43). A disadvantage of gating is that, although it is a solution for intrafraction motion management, it will even further increase the treatment time. Solutions for online monitoring and management of intrafraction motion such as cine MRI-based gated irradiation are not yet implemented for the 1.5-T Elekta MR-linac.
Several publications have reported on neoadjuvant MR-guided PBI on a conventional linac including favourable toxicity profiles (35, 37, 85). However, no patients have yet been treated with neoadjuvant PBI on a hybrid machine. A planning study has shown that neoadjuvant PBI in a single fraction in prone or supine position on the 1.5-T Elekta MR-linac would be dosimetrically feasible with adequate target coverage and within predefined constraints for OAR (63).
Experiences with adjuvant PBI on a hybrid system have been published. For patients treated on the 0.35-T 60Co Viewray system with single-fraction adjuvant PBI, up to 12 months' follow-up is available, and no local recurrences have been reported. The first clinical results showed good tolerability, low toxicity with a maximum of grade 2 toxicity, and good to excellent cosmetic outcome assessed by both patients and physician (86, 87). Usage of this system resulted in benefits for initial patient setup on lumpectomy cavity and online motion monitoring by which the PTV margin was diminished to 0 mm, which led to a large reduction in treatment volume of 52% (26, 43, 69, 75). The first patient has also been successfully treated with adjuvant PBI in 15 fractions on a 1.5-T Elekta MR-linac, which led to only grade 1 toxicity of the breast with adequate protection of the chin to prevent unwanted irradiation due to the ESE (66).
Patients are currently being recruited for several studies on MR-guided PBI. On ClinicalTrials.gov, two trials are registered aiming to treat patients in the adjuvant setting on a hybrid machine, looking primarily at either reproducibility of treatment or cosmetic outcome (88, 89). Three other trials are being conducted to further explore the effect of neoadjuvant MR-guided PBI on a conventional linac (90–92). The primary outcomes of these trials are postoperative complication rate, reproducibility of treatment, and pathologic response, respectively.
The addition of MR guidance to the breast RT planning pathway facilitates target volume delineation in the neoadjuvant PBI setting, whereas treatment on a hybrid MR and linac or 60Co machine could lead to reduced CTV to PTV margins in the neoadjuvant and adjuvant PBI settings through clearer visualization of the target volume during treatment. Although challenges for treatment of breast cancer patients on these systems remain (Table 1), the first breast cancer patients have been treated successfully with adjuvant PBI on a hybrid system, and studies of MR-guided neoadjuvant PBI will open shortly, through which technical approaches and workflow are likely to be further refined.
Written informed consent was obtained from the patients and volunteers for the publication of any potentially identifiable images or data included in this article. Medical images presented in this work were from subjects participating in the ABLATIVE trial (ClinicalTrials.gov identifier: NCT02316561) and were used with permission from the ABLATIVE trial team. The ABLATIVE protocol was approved by the Institutional Review Board of the University Medical Center Utrecht, Utrecht, the Netherlands.
AK, HB, AH, MG, and JV contributed to conception and design of this work. MG and JV wrote the first draft of the manuscript. All authors contributed to data interpretation, critical manuscript revision, read, and approved the submitted version.
All authors are part of the Elekta MR-Linac Research Consortium. The aim of this consortium is to coordinate international research into the 1.5 T Elekta MR-linac. All authors have received financial support from Elekta for visiting consortium meetings. Elekta had no role in the preparation, review, or approval of the manuscript, and decision to submit the manuscript for publication.
1. van Maaren MC, de Munck L, de Bock GH, Jobsen JJ, van Dalen T, Linn SC, et al. 10 year survival after breast-conserving surgery plus radiotherapy compared with mastectomy in early breast cancer in the Netherlands: a population-based study. Lancet Oncol. (2016). 17:1158–70. doi: 10.1016/S1470-2045(16)30067-5
2. Aalders KC, van Bommel ACM, van Dalen T, Sonke GS, van Diest PJ, Boersma LJ, et al. Contemporary risks of local and regional recurrence and contralateral breast cancer in patients treated for primary breast cancer. Eur J Cancer. (2016) 63:118–26. doi: 10.1016/j.ejca.2016.05.010
3. American Cancer Society. Cancer Facts & Figures 2020. Atlanta: American Cancer Society (2020). Available online at: https://www.cancer.org/research/cancer-facts-statistics.html
4. Curigliano G, Burstein HJ, Winer EP, Gnant M, Dubsky P, Loibl S, et al. De-escalating and escalating treatments for early-stage breast cancer: the St. gallen international expert consensus conference on the primary therapy of early breast cancer 2017. Ann Oncol. (2017) 28:1700–12. doi: 10.1093/annonc/mdx308
5. Coles CE, Griffin CL, Kirby AM, Titley J, Agrawal RK, Alhasso A, et al. Partial-breast radiotherapy after breast conservation surgery for patients with early breast cancer (UK IMPORT LOW trial):5-year results from a multicentre, randomised, controlled, phase 3, non-inferiority trial. Lancet. (2017) 90:1048–60. doi: 10.1016/S0140-6736(17)31145-5
6. Bhattacharya I, Haviland JS, Kirby AM, Kirwan CC, Hopwood P, Yarnold JR, et al. Patient-Reported outcomes over 5 years after whole- or partial-breast radiotherapy: longitudinal analysis of the IMPORT LOW (CRUK/06/003) phase III randomized controlled trial. J Clin Oncol. (2019) 37:305–17. doi: 10.1200/JCO.18.00982
7. Strnad V, Ott OJ, Hildebrandt G, Kauer-Dorner D, Knauerhase H, Major T, et al. 5-year results of accelerated partial breast irradiation using sole interstitial multicatheter brachytherapy versus whole-breast irradiation with boost after breast-conserving surgery for low-risk invasive and in-situ carcinoma of the female breast: a ran. Lancet. (2016). 387:229–38. doi: 10.1016/S0140-6736(15)00471-7
8. Whelan TJ, Julian JA, Berrang TS, Kim D-H, Germain I, Nichol AM, et al. External beam accelerated partial breast irradiation versus whole breast irradiation after breast conserving surgery in women with ductal carcinoma in situ and node-negative breast cancer (RAPID): a randomised controlled trial. Lancet. (2019) 394:2165–72. doi: 10.1016/S0140-6736(19)32515-2
9. Den Hartogh MD, Philippens MEP, van Dam IE, Kleynen CE, Tersteeg RJHA, Pijnappel RM, et al. MRI and CT imaging for preoperative target volume delineation in breast-conserving therapy. Radiat Oncol. (2014) 9:1–9. doi: 10.1186/1748-717X-9-63
10. van der Leij F, Elkhuizen PHM, Janssen TM, Poortmans P, van der Sangen M, Scholten AN, et al. Target volume delineation in external beam partial breast irradiation: less inter-observer variation with preoperative- compared to postoperative delineation. Radiother Oncol. (2014) 110:467–70. doi: 10.1016/j.radonc.2013.10.033
11. Lightowlers SV, Boersma LJ, Fourquet A, Kirova YM, Offersen BV, Poortmans P, et al. Preoperative breast radiation therapy: indications and perspectives. Eur J Cancer. (2017) 82:184–92. doi: 10.1016/j.ejca.2017.06.014
12. Lagendijk JJW, Raaymakers BW, Raaijmakers AJE, Overweg J, Brown KJ, Kerkhof EM, et al. MRI/linac integration. Radiother Oncol. (2008) 86:25–9. doi: 10.1016/j.radonc.2007.10.034
13. Raaymakers BW, Jürgenliemk-Schulz IM, Bol GH, Glitzner M, Kotte ANTJ, Van Asselen B, et al. First patients treated with a 1.5 T MRI-Linac: clinical proof of concept of a high-precision, high-field MRI guided radiotherapy treatment. Phys Med Biol. (2017) 62:L41–50. doi: 10.1088/1361-6560/aa9517
14. Mutic S, Dempsey JF. The viewray system: magnetic resonance–guided and controlled radiotherapy. Semin Radiat Oncol. (2014) 24:196–9. doi: 10.1016/j.semradonc.2014.02.008
15. Klüter S. Technical design and concept of a 0.35 T MR-Linac. Clin Transl Radiat Oncol. (2019) 18:98–101. doi: 10.1016/j.ctro.2019.04.007
16. Dundas K, Pogson EM, Batumalai V, Delaney GP, Boxer MM, Yap ML, et al. The impact of imaging modality (CT vs MRI) and patient position (supine vs prone) on tangential whole breast radiation therapy planning. Pract Radiat Oncol. (2018) 8:e87–e97. doi: 10.1016/j.prro.2017.07.007
17. Paulson ES, Erickson B, Schultz C, Allen Li X. Comprehensive MRI simulation methodology using a dedicated MRI scanner in radiation oncology for external beam radiation treatment planning. Med Phys. (2015) 42:28–39. doi: 10.1118/1.4896096
18. Schmidt MA, Payne GS. Radiotherapy planning using MRI. Phys Med Biol. (2015) 60:R323–61. doi: 10.1088/0031-9155/60/22/R323
19. Ahn KH, Hargreaves BA, Alley MT, Horst KC, Luxton G, Daniel BL, et al. MRI guidance for accelerated partial breast irradiation in prone position: imaging protocol design and evaluation. Int J Radiat Oncol Biol Phys. (2009) 75:285–93. doi: 10.1016/j.ijrobp.2009.03.063
20. Giezen M, Kouwenhoven E, Scholten AN, Coerkamp EG, Heijenbrok M, Jansen WPA, et al. MRI- versus CT-based volume delineation of lumpectomy cavity in supine position in breast-conserving therapy: an exploratory study. Int J Radiat Oncol Biol Phys. (2012) 82:1332–40. doi: 10.1016/j.ijrobp.2011.05.008
21. Jolicoeur M, Racine ML, Trop I, Hathout L, Nguyen D, Derashodian T, et al. Localization of the surgical bed using supine magnetic resonance and computed tomography scan fusion for planification of breast interstitial brachytherapy. Radiother Oncol. (2011) 100:480–4. doi: 10.1016/j.radonc.2011.08.024
22. Batumalai V, Liney G, DeLaney GP, Rai R, Boxer M, Min M, et al. Assessment of MRI image quality for various setup positions used in breast radiotherapy planning. Radiother Oncol. (2016) 119:57–60. doi: 10.1016/j.radonc.2016.02.024
23. Liney GP, Moerland MA. Magnetic resonance imaging acquisition techniques for radiotherapy planning. Semin Radiat Oncol. (2014) 24:160–8. doi: 10.1016/j.semradonc.2014.02.014
24. Pogson EM, Delaney GP, Ahern V, Boxer MM, Chan C, David S, et al. Comparison of magnetic resonance imaging and computed tomography for breast target volume delineation in prone and supine positions. Int J Radiat Oncol Biol Phys. (2016) 96:905–12. doi: 10.1016/j.ijrobp.2016.08.002
25. Tijssen RHN, Philippens MEP, Paulson ES, Glitzner M, Chugh B, Wetscherek A, et al. MRI commissioning of 1.5T MR-linac systems – a multi-institutional study. Radiother Oncol. (2019) 132:114–20. doi: 10.1016/j.radonc.2018.12.011
26. Acharya S, Fischer-Valuck BW, Mazur TR, Curcuru A, Sona K, Kashani R, et al. Magnetic resonance image guided radiation therapy for external beam accelerated partial-breast irradiation: evaluation of delivered dose and intrafractional cavity motion. Int J Radiat Oncol Biol Phys. (2016) 96:785–92. doi: 10.1016/j.ijrobp.2016.08.006
27. Prott F-J, Haverkamp U, Eich H, Resch A, Micke O, Fischedick A-R, et al. Effect of distortions and asymmetry in MR images on radiotherapeutic treatment planning. Int J Cancer. (2000) 90:46–50. doi: 10.1002/(SICI)1097-0215(20000220)90:1<46::AID-IJC6>3.0.CO;2-6
28. Fransson A, Andreo P, Pötter R. Strahlentherapie und onkologie aspects of MR image distortions in radiotherapy treatment planning. Strahlentherapie und Onkol. (2001) 177:59–73. doi: 10.1007/PL00002385
29. Walker A, Metcalfe P, Liney G, Batumalai V, Dundas K, Glide-Hurst C, et al. MRI geometric distortion: impact on tangential whole-breast IMRT. J Appl Clin Med Phys. (2016) 17:7–19. doi: 10.1120/jacmp.v17i5.6242
30. Walker A, Liney G, Metcalfe P, Holloway L. MRI distortion: considerations for MRI based radiotherapy treatment planning. Australas Phys Eng Sci Med. (2014) 37:103–13. doi: 10.1007/s13246-014-0252-2
31. Das IJ, McGee KP, Tyagi N, Wang H. Role and future of MRi in radiation oncology. Br J Radiol. (2019) 91:1–14. doi: 10.1259/bjr.20180505
32. Tijssen RHN, Crijns SPM, Bluemink JJ, Hackett SS, De Vries JHW, Kruiskamp MJ, et al. OC-0257: comprehensive MRI acceptance testing & commissioning of a 1.5T MR-linac: guidelines and results. Radiother Oncol. (2017) 123:S130–1. doi: 10.1016/S0167-8140(17)30700-4
33. Ginn JS, Agazaryan N, Cao M, Baharom U, Low DA, Yang Y, et al. Characterization of spatial distortion in a 0.35 T MRI-guided radiotherapy system. Phys Med Biol. (2017) 62:4525–40. doi: 10.1088/1361-6560/aa6e1a
34. Kirby AM, Yarnold JR, Evans PM, Morgan VA, Schmidt MA, Scurr ED, et al. Tumor bed delineation for partial breast and breast boost radiotherapy planned in the prone position: what does MRI add to X-ray CT localization of titanium clips placed in the excision cavity wall? Int J Radiat Oncol Biol Phys. (2009) 74:1276–82. doi: 10.1016/j.ijrobp.2009.02.028
35. Horton JK, Blitzblau RC, Yoo S, Geradts J, Chang Z, Baker JA, et al. Preoperative single-fraction partial breast radiation therapy: a novel phase 1, dose-escalation protocol with radiation response biomarkers. Int J Radiat Oncol Biol Phys. (2015) 92:846–55. doi: 10.1016/j.ijrobp.2015.03.007
36. Vasmel JE, Groot Koerkamp ML, Kirby AM, Russell NS, Shaitelman SF, Vesprini D, et al. Consensus on contouring primary breast tumors on MRI in the setting of neoadjuvant partial breast irradiation in trials. Pract Radiat Oncol. (in press). doi: 10.1016/j.prro.2020.03.011
37. Guidolin K, Yaremko B, Lynn K, Gaede S, Kornecki A, Muscedere G, et al. Stereotactic image-guided neoadjuvant ablative single-dose radiation, then lumpectomy, for early breast cancer: the SIGNAL prospective single-arm trial of single-dose radiation therapy. Curr Oncol. (2019) 26:1–7. doi: 10.3747/co.26.4479
38. Mouawad M, Biernaski H, Brackstone M, Lock M, Yaremko B, Sexton T, et al. Reducing the dose of gadolinium-based contrast agents for DCE-MRI guided SBRT: the effects on inter and intra observer variability for preoperative target volume delineation in early stage breast cancer patients. Radiother Oncol. (2019) 131:60–5. doi: 10.1016/j.radonc.2018.11.020
39. Glover GH. Multipoint dixon technique for water and fat proton and susceptibility imaging. J Magn Reson Imaging. (1991) 1:521–30. doi: 10.1002/jmri.1880010504
40. Van Heijst TCF, Van Asselen B, Pijnappel RM, Cloos-van Balen M, Lagendijk JJW, Van den Bongard D, et al. MRI sequences for the detection of individual lymph nodes in regional breast radiotherapy planning. Br J Radiol. (2016) 89:20160072. doi: 10.1259/bjr.20160072
41. Partridge SC, Nissan N, Rahbar H, Kitsch AE, Sigmund EE. Diffusion-weighted breast MRI: Clinical applications and emerging techniques. J Magn Reson Imaging. (2017) 45:337–55. doi: 10.1002/jmri.25479
42. Leibfarth S, Winter RM, Lyng H, Zips D, Thorwarth D. Potentials and challenges of diffusion-weighted magnetic resonance imaging in radiotherapy. Clin Transl Radiat Oncol. (2018) 13:29–37. doi: 10.1016/j.ctro.2018.09.002
43. Henke LE, Contreras JA, Green OL, Cai B, Kim H, Roach MC, et al. Magnetic resonance image-guided radiotherapy (MRIgRT): a 4.5-year clinical experience. Clin Oncol. (2018) 30:720–7. doi: 10.1016/j.clon.2018.08.010
44. Major T, Gutiérrez C, Guix B, van Limbergen E, Strnad V, Polgár C. Recommendations from GEC ESTRO breast cancer working group (II): target definition and target delineation for accelerated or boost partial breast irradiation using multicatheter interstitial brachytherapy after breast conserving open cavity surgery. Radiother Oncol. (2016) 118:199–204. doi: 10.1016/j.radonc.2015.12.006
45. Offersen B V, Boersma LJ, Kirkove C, Hol S, Aznar MC, Biete Sola A, et al. ESTRO consensus guideline on target volume delineation for elective radiation therapy of early stage breast cancer. Radiother Oncol. (2015) 114:3–10. doi: 10.1016/j.radonc.2014.11.030
46. Strnad V, Hannoun-Levi J-M, Guinot J-L, Lössl K, Kauer-Dorner D, Resch A, et al. Recommendations from GEC ESTRO breast cancer working Group (I): target definition and target delineation for accelerated or boost partial breast irradiation using multicatheter interstitial brachytherapy after breast conserving closed cavity surgery. Radiother Oncol. (2015) 115:342–8. doi: 10.1016/j.radonc.2015.06.010
47. Boersma LJ, Janssen T, Elkhuizen PHM, Poortmans P, van der Sangen M, Scholten AN, et al. Reducing interobserver variation of boost-CTV delineation in breast conserving radiation therapy using a pre-operative CT and delineation guidelines. Radiother Oncol. (2012) 103:178–82. doi: 10.1016/j.radonc.2011.12.021
48. Mast M, Coerkamp E, Heijenbrok M, Scholten A, Jansen W, Kouwenhoven E, et al. Target volume delineation in breast conserving radiotherapy: are co-registered CT and MR images of added value? Radiat Oncol. (2014) 9(Lc):65. doi: 10.1186/1748-717X-9-65
49. Christopherson KM, Smith BD. How does MR imaging help care for my breast cancer patient? Perspective of a radiation oncologist. Magn Reson Imaging Clin N Am. (2018) 26:295–302. doi: 10.1016/j.mric.2017.12.007
50. Den Hartogh MD, Philippens MEP, Van Dam IE, Kleynen CE, Tersteeg RJHA, Kotte ANTJ, et al. Post-lumpectomy CT-guided tumor bed delineation for breast boost and partial breast irradiation: can additional pre- and postoperative imaging reduce interobserver variability? Oncol Lett. (2015) 10:2795–801. doi: 10.3892/ol.2015.3697
51. Al-Hammadi N, Caparrotti P, Divakar S, Riyas M, Chandramouli SH, Hammoud R, et al. MRI reduces variation of contouring for boost clinical target volume in breast cancer patients without surgical clips in the tumour bed. Radiol Oncol. (2017) 51:160–8. doi: 10.1515/raon-2017-0014
52. Charaghvandi RK, van Asselen B, Philippens MEP, Verkooijen HM, van Gils CH, van Diest PJ, et al. Redefining radiotherapy for early-stage breast cancer with single dose ablative treatment: a study protocol. BMC Cancer. (2017) 17:181. doi: 10.1186/s12885-017-3144-5
53. Seely JM, Hill F, Peddle S, Lau J. An evaluation of patient experience during percutaneous breast biopsy. Eur Radiol. (2017) 27:4804–11. doi: 10.1007/s00330-017-4872-2
54. Raaymakers BW, Raaijmakers AJE, Kotte ANTJ, Jette D, Lagendijk JJW. Integrating a MRI scanner with a 6 MV radiotherapy accelerator: dose deposition in a transverse magnetic field. Phys Med Biol. (2004) 49:4109–18. doi: 10.1088/0031-9155/49/17/019
55. Raaijmakers AJE, Raaymakers BW, Lagendijk JJW. Integrating a MRI scanner with a 6 MV radiotherapy accelerator: dose increase at tissue-air interfaces in a lateral magnetic field due to returning electrons. Phys Med Biol. (2005) 50:1363–76. doi: 10.1088/0031-9155/50/7/002
56. Raaijmakers AJE, Raaymakers BW, Van Der Meer S, Lagendijk JJW. Integrating a MRI scanner with a 6 MV radiotherapy accelerator: impact of the surface orientation on the entrance and exit dose due to the transverse magnetic field. Phys Med Biol. (2007) 52:929–39. doi: 10.1088/0031-9155/52/4/005
57. Raaijmakers AJE, Raaymakers BW, Lagendijk JJW. Magnetic-field-induced dose effects in MR-guided radiotherapy systems: dependence on the magnetic field strength. Phys Med Biol. (2008) 53:909–23. doi: 10.1088/0031-9155/53/4/006
58. Oborn BM, Metcalfe PE, Butson MJ, Rosenfeld AB. High resolution entry and exit monte carlo dose calculations from a linear accelerator 6 MV beam under the influence of transverse magnetic fields. Med Phys. (2009) 36:3549–59. doi: 10.1118/1.3157203
59. Oborn BM, Metcalfe PE, Butson MJ, Rosenfeld AB. Monte carlo characterization of skin doses in 6 MV transverse field MRI-linac systems: effect of field size, surface orientation, magnetic field strength, and exit bolus. Med Phys. (2010) 37:5208–17. doi: 10.1118/1.3488980
60. van Heijst TCF, den Hartogh MD, Lagendijk JJW, van den Bongard HJGD, van Asselen B. MR-guided breast radiotherapy: feasibility and magnetic-field impact on skin dose. Phys Med Biol. (2013) 58:5917–30. doi: 10.1088/0031-9155/58/17/5917
61. Esmaeeli AD, Mahdavi SR, Pouladian M, Monfared AS, Bagheri S. Improvement of dose distribution in breast radiotherapy using a reversible transverse magnetic field linac-MR unit. Med Phys. (2013) 41:011709. doi: 10.1118/1.4845175
62. Kim A, Lim-Reinders S, Mccann C, Ahmad SB, Sahgal A, Lee J, et al. Magnetic field dose effects on different radiation beam geometries for hypofractionated partial breast irradiation. J Appl Clin Med Phys. (2017) 18:62–70. doi: 10.1002/acm2.12182
63. Charaghvandi KR, Van't Westeinde T, Yoo S, Houweling AC, Rodrigues A, Verkooijen HM, et al. Single dose partial breast irradiation using an MRI linear accelerator in the supine and prone treatment position. Clin Transl Radiat Oncol. (2019) 14:1–7. doi: 10.1016/j.ctro.2018.09.001
64. Park JM, Shin KH, Kim J, in Park SY, Jeon SH, Choi N, et al. Air–electron stream interactions during magnetic resonance IGRT: skin irradiation outside the treatment field during accelerated partial breast irradiation. Strahlentherapie und Onkol. (2018) 194:50–9. doi: 10.1007/s00066-017-1212-z
65. Malkov VN, Hackett SL, Wolthaus JWH, Raaymakers BW, van Asselen B. Monte carlo simulations of out-of-field surface doses due to the electron streaming effect in orthogonal magnetic fields to. Phys Med Biol. (2019) 64:115029. doi: 10.1088/1361-6560/ab0aa0
66. Nachbar M, Mönnich D, Boeke S, Gani C, Weidner N, Heinrich V, et al. Partial breast irradiation with the 1.5 T MR-Linac: First patient treatment and analysis of electron return and stream effects. Radiother Oncol. (2019) 145:30–5. doi: 10.1016/j.radonc.2019.11.025
67. Werensteijn-Honingh AM, Kroon PS, Winkel D, Aalbers EM, van Asselen B, Bol GH, et al. Feasibility of stereotactic radiotherapy using a 1.5 T MR-linac: multi-fraction treatment of pelvic lymph node oligometastases. Radiother Oncol. (2019) 134:50–4. doi: 10.1016/j.radonc.2019.01.024
68. Yoo S, Blitzblau R, Yin FF, Horton JK. Dosimetric comparison of preoperative single-fraction partial breast radiotherapy techniques: 3D CRT, noncoplanar IMRT, coplanar IMRT, and VMAT. J Appl Clin Med Phys. (2015) 16:5126. doi: 10.1120/jacmp.v16i1.5126
69. Fischer-Valuck BW, Henke L, Green O, Kashani R, Acharya S, Bradley JD, et al. Two-and-a-half-year clinical experience with the world's first magnetic resonance image guided radiation therapy system. Adv Radiat Oncol. (2017) 2:485–93.
70. Johnstone E, Wyatt JJ, Henry AM, Short SC, Sebag-Montefiore D, Murray L, et al. Systematic review of synthetic computed tomography generation methodologies for use in magnetic resonance imaging–only radiation therapy. Int J Radiat Oncol Biol Phys. (2018) 100:199–217. doi: 10.1016/j.ijrobp.2017.08.043
71. Han X. MR-based synthetic CT generation using a deep convolutional neural network method. Med Phys. (2017). 44:1408–19. doi: 10.1002/mp.12155
72. Su K, Friel HT, Kuo J, Al Helo R, Baydoun A, Stehning C, et al. UTE-mDixon-based thorax synthetic CT ceneration. Med Phys. (2019) 46(8):3520–31. doi: 10.1002/mp.13574
73. Freedman JN, Bainbridge H, Nill S, Collins DJ, Kachelriess M, Leach MO, et al. Synthetic 4D-CT of the thorax for treatment plan adaptation on MR-guided radiotherapy systems. Phys Med Biol. (2019) 64:115005. doi: 10.1088/1361-6560/ab0dbb
74. Ahunbay EE, Thapa R, Chen X, Paulson E, Li XA. A technique to rapidly generate synthetic computed tomography for magnetic resonance imaging–guided online adaptive replanning: an exploratory study. Int J Radiat Oncol Biol Phys. (2019) 103:1261–70. doi: 10.1016/j.ijrobp.2018.12.008
75. Jeon SH, Shin KH, Park S-Y, Kim J, Park JM, Kim JH, et al. Seroma change during magnetic resonance imaging-guided partial breast irradiation and its clinical implications. Radiat Oncol. (2017) 12:103. doi: 10.1186/s13014-017-0843-7
76. International Commission on Radiation Units Measurements. Prescribing, recording, reporting photon-beam intensity-modulated radiation therapy (IMRT). ICRU report 83. J ICRU. (2010) 10:1–106. doi: 10.1093/jicru/ndq002
77. Winkel D, Bol GH, Kiekebosch IH, Van Asselen B, Kroon PS, Jürgenliemk-Schulz IM, et al. Evaluation of online plan adaptation strategies for the 1.5T MR-linac based on “First-In-Man” treatments. Cureus. (2018) 10:1–7. doi: 10.7759/cureus.2431
78. Acharya S, Fischer-Valuck BW, Kashani R, Parikh P, Yang D, Zhao T, et al. Online magnetic resonance image guided adaptive radiation therapy: first clinical applications. Int J Radiat Oncol Biol Phys. (2016) 94:394–403. doi: 10.1016/j.ijrobp.2015.10.015
79. Winkel D, Kroon PS, Werensteijn-Honingh AM, Bol GH, Raaymakers BW, Jürgenliemk-Schulz IM. Simulated dosimetric impact of online replanning for stereotactic body radiation therapy of lymph node oligometastases on the 1.5T MR-linac. Acta Oncol (Madr). (2018) 57:1705–12. doi: 10.1080/0284186X.2018.1512152
80. Taupin F, Flaender M, Delorme R, Brochard T, Mayol JF, Arnaud J, et al. Gadolinium nanoparticles and contrast agent as radiation sensitizers. Phys Med Biol. (2015) 60:4449–64. doi: 10.1088/0031-9155/60/11/4449
81. van Heijst TCF, Philippens MEP, Charaghvandi RK, den Hartogh MD, Lagendijk JJW, van den Bongard HJGD, et al. Quantification of intra-fraction motion in breast radiotherapy using supine magnetic resonance imaging. Phys Med Biol. (2016) 61:1352–70. doi: 10.1088/0031-9155/61/3/1352
82. van Herk M, McWilliam A, Dubec M, Faivre-Finn C, Choudhury A. Magnetic resonance imaging–guided radiation therapy: a short strengths, weaknesses, opportunities, and threats analysis. Int J Radiat Oncol Biol Phys. (2018) 101:1057–60. doi: 10.1016/j.ijrobp.2017.11.009
83. Park JM, Wu HG, Kim HJ, Choi CH, Kim JI. Comparison of treatment plans between IMRT with MR-linac and VMAT for lung SABR. Radiat Oncol. (2019) 14:1–8. doi: 10.1186/s13014-019-1314-0
84. Kontaxis C, Bol GH, Stemkens B, Glitzner M, Prins FM, Kerkmeijer LGW, et al. Towards fast online intrafraction replanning for free-breathing stereotactic body radiation therapy with the MR-linac. Phys Med Biol. (2017) 62:7233–48. doi: 10.1088/1361-6560/aa82ae
85. Vasmel JE, Charaghvandi RK, Houweling AC, Philippens MEP, van Asselen B, Vreuls CPH, et al. Tumor response following neoadjuvant MR-guided single ablative dose partial breast irradiation. Int J Radiat Oncol. (2020) 106:821–9. doi: 10.1016/j.ijrobp.2019.11.406
86. Zoberi I, Thomas MA, Ochoa LL. Evaluation of single fraction high-gradient partial breast irradiation as the sole method of radiation therapy for low-risk stage 0 and i breast cancer—early results of a single institution prospective clinical trial. Int J Radiat Oncol Biol Phys. (2017) 99:E61. doi: 10.1016/j.ijrobp.2017.06.736
87. Kennedy WR, Thomas MA, DeBroeck KR, Ochoa LL, Atkinson AR, Green OL, et al. Postoperative single-fraction partial breast irradiation for low-risk stage 0 and i breast carcinomas: results of a prospective clinical trial. Int J Radiat Oncol Biol Phys. (2018) 102:S227–8. doi: 10.1016/j.ijrobp.2018.07.159
88. Anderson B. Real-time MRI-Guided 3-Fraction Accelerated Partial Breast Irradiation in Early Breast Cancer (Clinical Trials.gov Identifier NCT03936478). (2019)
89. Gani C. Feasibility of Online MR-Guided Radiotherapy on a 1.5T MR-Linac (Clinical trials.gov Identifier NCT04172753). (2019).
90. Currey A. MRI-Based Preoperative Accelerated Partial Breast Irradiation (Clinical trials.gov Identifier NCT02728076). (2016).
Keywords: breast cancer, neoadjuvant radiation therapy, partial breast irradiation, MR-guided radiotherapy, hybrid machine, MR-linac, magnetic resonance imaging (MRI)
Citation: Groot Koerkamp ML, Vasmel JE, Russell NS, Shaitelman SF, Anandadas CN, Currey A, Vesprini D, Keller BM, De-Colle C, Han K, Braunstein LZ, Mahmood F, Lorenzen EL, Philippens MEP, Verkooijen HM, Lagendijk JJW, Houweling AC, van den Bongard HJGD and Kirby AM (2020) Optimizing MR-Guided Radiotherapy for Breast Cancer Patients. Front. Oncol. 10:1107. doi: 10.3389/fonc.2020.01107
Received: 14 February 2020; Accepted: 03 June 2020;
Published: 28 July 2020.
Edited by:
Cristiane Takita, University of Miami Health System, United StatesReviewed by:
Yidong Yang, University of Science and Technology of China, ChinaCopyright © 2020 Groot Koerkamp, Vasmel, Russell, Shaitelman, Anandadas, Currey, Vesprini, Keller, De-Colle, Han, Braunstein, Mahmood, Lorenzen, Philippens, Verkooijen, Lagendijk, Houweling, van den Bongard and Kirby. This is an open-access article distributed under the terms of the Creative Commons Attribution License (CC BY). The use, distribution or reproduction in other forums is permitted, provided the original author(s) and the copyright owner(s) are credited and that the original publication in this journal is cited, in accordance with accepted academic practice. No use, distribution or reproduction is permitted which does not comply with these terms.
*Correspondence: Maureen L. Groot Koerkamp, bS5sLmdyb290a29lcmthbXAtM0B1bWN1dHJlY2h0Lm5s; Jeanine E. Vasmel, ai5lLnZhc21lbEB1bWN1dHJlY2h0Lm5s; Anna M. Kirby, YW5uYS5raXJieUBybWgubmhzLnVr
†These authors share first authorship
Disclaimer: All claims expressed in this article are solely those of the authors and do not necessarily represent those of their affiliated organizations, or those of the publisher, the editors and the reviewers. Any product that may be evaluated in this article or claim that may be made by its manufacturer is not guaranteed or endorsed by the publisher.
Research integrity at Frontiers
Learn more about the work of our research integrity team to safeguard the quality of each article we publish.