- 1Department of Endocrinology and Nutrition, Virgen de la Victoria University Hospital, Institute of Biomedical Research of Malaga, University and Malaga, Malaga, Spain
- 2CIBER Physiopathology of Obesity and Nutrition (CIBERobn), Institute of Health Carlos III (ISCIII), Madrid, Spain
- 3Department of Medical Oncology, Virgen de la Victoria University Hospital, Institute of Biomedical Research of Malaga, University and Malaga, Malaga, Spain
- 4Department of Biochemistry and Molecular Biology B and Immunology, Faculty of Medicine, University of Murcia, Murcia, Spain
- 5Biomedical Research Institute of Murcia (IMIB), Murcia, Spain
The polyamines putrescine, spermidine, and spermine are widely distributed polycationic compounds essential for cellular functions. Intracellular polyamine pools are tightly regulated by a complex regulatory mechanism involving de novo biosynthesis, catabolism, and transport across the plasma membrane. In mammals, both the production of polyamines and their uptake from the extracellular space are controlled by a set of proteins named antizymes and antizyme inhibitors. Dysregulation of polyamine levels has been implicated in a variety of human pathologies, especially cancer. Additionally, decreases in the intracellular and circulating polyamine levels during aging have been reported. The differences in the polyamine content existing among tissues are mainly due to the endogenous polyamine metabolism. In addition, a part of the tissue polyamines has its origin in the diet or their production by the intestinal microbiome. Emerging evidence has suggested that exogenous polyamines (either orally administrated or synthetized by the gut microbiota) are able to induce longevity in mice, and that spermidine supplementation exerts cardioprotective effects in animal models. Furthermore, the administration of either spermidine or spermine has been shown to be effective for improving glucose homeostasis and insulin sensitivity and reducing adiposity and hepatic fat accumulation in diet-induced obesity mouse models. The exogenous addition of agmatine, a cationic molecule produced through arginine decarboxylation by bacteria and plants, also exerts significant effects on glucose metabolism in obese models, as well as cardioprotective effects. In this review, we will discuss some aspects of polyamine metabolism and transport, how diet can affect circulating and local polyamine levels, and how the modulation of either polyamine intake or polyamine production by gut microbiota can be used for potential therapeutic purposes.
Introduction
Polyamines are small aliphatic amines that are present in all living organisms from bacteria to human beings. They can act as polycations since they are positively charged at physiological pH. The major polyamines in mammalian cells are spermidine, spermine, and their precursor the diamine putrescine (1, 2) (Figure 1). In addition of these molecules, microorganisms, and plants also synthesize other types of polyamines such as cadaverine, agmatine, and thermospermine (3–5) (Figure 1), and even minority polyamines (thermine, caldine, thermospermine, etc.) have been detected in extreme microorganisms (6). Agmatine and cadaverine are also present in very low amount in certain mammalian tissues (7, 8). Although polyamines were discovered in the seventeenth century, major advances in their metabolism and functions were achieved in the second part of the last century (2, 9–11). Numerous experiments have shown that due to their polycationic nature, polyamines can readily bind to negatively charged biomolecules including DNA, RNA, proteins, and phospholipids, modulating in many cases the function of these macromolecules (12, 13). When polyamine metabolism was pharmacologically or genetically altered, many relevant biochemical, and cellular processes resulted affected, including translation, transcription, signal transduction, cell proliferation, and differentiation, apoptosis, or cell stress response (14–19). However, the molecular mechanisms by which polyamines exert their biological effects are only partially understood (20).
In humans, only two genetic diseases affecting enzymes of the biosynthetic pathway of polyamines have been described so far (21, 22). However, several lines of evidence have implicated a dysregulation of the polyamine system in hyperproliferative and neurodegenerative diseases (23–27). The alteration of polyamine metabolism in several types of cancer has sustained the interest in using the polyamine pathway as a target for anticancer therapy (28, 29). In this regard, it is clear that the limitation of the cellular polyamine content has a potential interest in cancer chemoprevention or in the treatment of other pathologies such as certain infectious diseases and type 1 diabetes (28, 30, 31). On the other hand, experiments conducted in different cellular and animal models have revealed that the exogenous administration of spermidine or other natural polyamines may exert beneficial effects by affecting processes such as cellular stress, chronic inflammation, or dysregulated lipid or glucose metabolism (32–34). In this review, we will discuss different aspects related with the homeostasis of polyamines in mammalian tissues, including the relevance of the polyamines generated by the gut microbiota, how the endogenous levels of polyamines can be affected by the administration of exogenous polyamines, and the impact that these treatments may exert on the evolution of the biochemical changes associated with aging or obesity-related metabolic diseases.
Polyamine Functions
Polyamines are essential for life. In fact, the inactivation of the genes that control the biosynthesis of putrescine or spermidine are embryo-lethal in mice (35, 36). This is not surprising due to the multiple essential cellular actions that have being ascribed to polyamines. Thus, owing to their general interaction with nucleic acids, they can affect many processes in which DNA, RNA, or proteins participate as substrates (13, 37, 38). Particularly interesting is the relationship between polyamines and reactive oxygen species (ROS). Although polyamine catabolism may generate potentially toxic products such as H2O2 and polyamine derived aldehydes associated to pathophysiological consequences (39, 40), numerous in vitro and in vivo experiments have suggested that spermine and spermidine may act as scavengers of ROS, and then protecting DNA from oxidative damage (41–43). This double-edged role of polyamines appears to be dependent of certain factors (44). One of these factors in in vitro studies could be the use of animal serum in the cell culture medium, which contains amino oxidases that can oxidize exogenously administrated polyamines and generate ROS, resulting in cell toxicity independently of the action of the polyamine itself. Interestingly, a recent work demonstrated that in the presence of human serum, polyamine administration to the culture medium does not increase ROS production and does not affect cell viability as in the case of the same experiment in presence of either bovine or horse serum (45). Importantly, studies showing a polyamine-dependent cell toxicity in human cell lines in presence of significant amounts of bovine/horse serum should be reevaluated with human serum to corroborate that toxicity could be due to the production of oxidized polyamine-derived products by the action of serum polyamine oxidases and not to a toxic effect of the polyamines per se.
Polyamine Content and its Regulation
Cellular Polyamine Levels and Distribution
In general, spermidine and spermine are the most abundant polyamines in mammalian cells. In addition, their absolute values and the spermidine/spermine ratio depend on the type of cell and tissue (46, 47), and their tissue concentrations are affected by several factors including age (46–49). In mammalian cells, polyamines are present at relatively high concentration compared with other biogenic amines. Total polyamine concentration is at the mM range. However, it has been estimated that the free intracellular concentration of each polyamine is much lower (7–15% of total for spermidine, and 2–5% for spermine), due to the fact that a high percentage of total polyamines are bound by ionic interactions to nucleic acids, proteins, and other negatively charged molecules in the cell (12, 50). According to these data, only a small fraction of the cellular polyamines appears to be metabolically active. In addition, another unsettled aspect of polyamines is related to the subcellular distribution of these molecules inside mammalian cells. In rat liver mitochondria, spermidine, and spermine are present at estimated concentrations of 1.21 and 0.62 mM, respectively (51). Polyamines are also stored in vesicles of secretory cells and neurons (52–54). The intracellular levels of polyamines depend on several factors: polyamine biosynthesis, catabolism, uptake, and efflux. It is generally accepted that a high proportion of the cellular polyamines have an endogenous origin, although in some cases the exogenous supply (i.e., dietary and gut microbiota-derived polyamines) may also significantly affect the polyamine content. Regarding circulating polyamines, several studies have shown that they are present at the low μM range in the blood of both humans and mice (55, 56). It is not clear whether blood polyamine levels are age-dependent (48).
Polyamine Biosynthesis and Catabolism
In mammalian cells, the polyamine biosynthetic pathway consists in four steps catalyzed by enzymes mainly located in the cytosol (Figure 2). In this process, L-ornithine, and S-adenosyl methionine (AdoMet) are used as substrates. The diamine putrescine is synthesized by ornithine decarboxylase (ODC), a key enzyme that presents a complex regulation (57). This diamine can be converted into spermidine by the addition of an aminopropyl moiety donated by decarboxylated S-adenosyl methionine (dcAdoMet) through the action of spermidine synthase (5, 58). The tetra-amine spermine is synthesized by another aminopropyl transferase (spermine synthase) through the incorporation of another aminopropyl group to the amino butyl end of spermidine (5, 59). The second key-rate enzyme in the synthesis of spermidine and spermine is S-adenosyl methionine decarboxylase (AdoMetDC, AMD1), which produces dcAdoMet used as aminopropyl donor for the formation of both polyamines (60). Other biochemical route that participates in the regulation of the intracellular levels of polyamines is the so-called back-conversion pathway of polyamines (Figure 2). Spermidine/spermine N1 acetyltransferase (SSAT) is a cytosolic enzyme that plays a key role in reducing intracellular polyamine levels, through the acetylation of spermine and spermidine to produce in first instance acetyl-spermine or acetyl-spermidine (61, 62), which are either excreted from the cell or oxidized to spermidine or putrescine, respectively, by an acetyl polyamine oxidase (PAOX) (63). PAOX is a FAD-dependent enzyme located in peroxisomes that generates, apart from the corresponding polyamine, the potentially toxic byproducts 3-acetamidopropanal and H2O2 (40). Spermine oxidase (SMOX), another FAD-containing enzyme, is capable of directly oxidizing spermine to spermidine, producing a 3-aminopropanal and H2O2 (64, 65). Due to its localization outside of peroxisomes, the overexpression of the enzyme may enhance the oxidative damage by both increasing H2O2 production and decreasing spermine levels (40, 66).
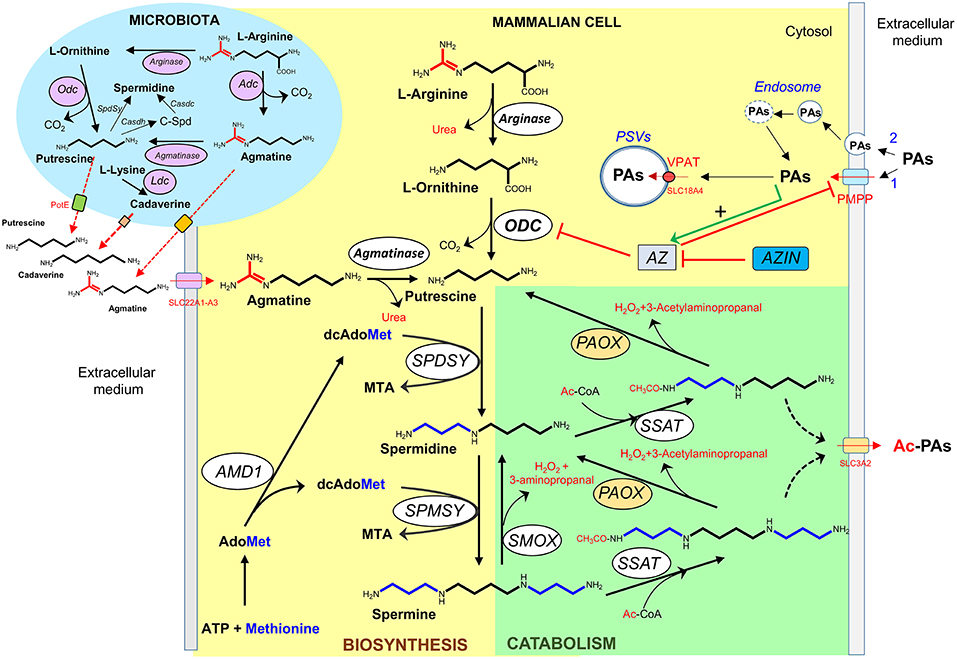
Figure 2. Polyamine metabolism and transport. Biosynthetic (yellow) and degradative (green) pathways of polyamines in mammalian cells, and bacteria (blue). PAs, polyamines; Ac-PAs, acetylated polyamines; C-Spd, carboxyspermidine; PSVs, polyamine sequestering vesicles; PMPP, plasma membrane polyamine permease; VPAT, vesicular polyamine transporter; ODC, Odc, ornithine decarboxylase; Adc, arginine decarboxylase; Ldc, lysine decarboxylase; AMD1, S-adenosylmethionine decarboxylase; SPDSY, Spdsy, spermidine synthase; SPMSY, spermine synthase; SSAT, spermidine/spermine acetyl transferase; PAOX, acetylpolyamine oxidase (microsomal); SMOX, spermine oxidase; AZ, antizyme; AZIN, antizyme inhibitor; Casdh, carboxyspermidine dehydrogenase; Casdc, carboxyspermidine decarboxylase; AdoMet, S-adenosylmethionine; dcAdoMet, decarboxylated S-adenosylmethionine; MTA, methylthioadenosine; Ac-CoA, acetyl-coenzyme A.
Agmatine (decarboxylated arginine) is an aminoguanidine structurally related to polyamines that behaves as a dication at physiological pH. Although agmatine is synthesized in bacteria and plants by arginine decarboxylase (ADC) (Figure 2), its biosynthesis in mammalian cells is controversial. In fact, the product of the initially reported ADC clone (67) was later demonstrated to be devoid of ADC activity by various independent groups. Instead, it encoded an ornithine decarboxylase antizyme inhibitor (AZIN2) (68, 69). Nowadays, multiple pharmacological effects of agmatine have been reported with potential therapeutic interest (70, 71), but to our knowledge clinical applications have not been yet implemented.
Polyamine Transport
Polyamines can enter and exit the cells through different type of carriers. Whereas, in bacteria and fungi, multiple polyamine transporters have been fully characterized (72), in mammalian cells lesser is known about the molecular components related with polyamine transport (73). It is generally accepted that in these cells the polyamine uptake activity is affected by the polyamine requirements of the cells. Thus, enhanced polyamine uptake is characteristic of cells with either high proliferating activity or in which cellular polyamine levels have been depleted by inhibitors of the biosynthetic pathway (74). Although the kinetics and some biochemical properties of polyamine transport have been studied in different types of cell lines and cell mutants, many questions about the “polyamine transport system” are still unsolved. It is possible that the polyamine carriers belong to the solute transport family (SLC) that contains about 400 annotated members. Different experiments of cell transfection by genes of the organic cation transporter (OCT) family or the cationic amino acid transporter (CAT) family have identified genes that could participate in the polyamine uptake system, but not a clear picture has emerged from all these studies (73, 75–78). On the other hand, polyamine export has also been detected in a variety of mammalian cells, having been identified SLC3A2 as a component of a diamine exporter that could participate in the excretion of putrescine and acetylated spermidine (79) (Figure 2). Apart from the polyamine transport system, endocytic pathways for the uptake of circulating polyamines have been described. In one model, polyamines bind electrostatically to the heparan sulfate chains of glypican-1, a proteoglycan of the cell surface, and then are taken into the cells through endocytosis (80). In another model, polyamines interact with non-defined polyamine binding protein(s) and the complex is internalized by caveolar endocytosis by a process that is negatively regulated by caveolin-1 (81).
Regulation of Polyamine Levels by Antizymes and Antizyme Inhibitors
The control of the polyamine homeostasis in mammals is crucial for maintaining cellular functions, and dysregulation of the cellular polyamine levels has been associated to diverse pathological conditions (20). Antizymes (AZs) are regulatory proteins involved in the control of intracellular polyamine levels through the modulation of both polyamine biosynthesis and uptake. AZs affect the polyamine biosynthetic route by interacting with the ODC monomer and preventing the formation of active ODC homodimers, and by stimulating the degradation of ODC by the proteasome without ubiquitination (82). They also affect the import of extracellular polyamines by inhibiting the plasma membrane polyamine transport system by a still unknown mechanism (83).
The negative effects that AZs exert on both the biosynthesis of intracellular polyamines and the polyamine uptake can be abrogated by the action of antizyme inhibitors (AZINs), proteins homologous to ODC but devoid of enzymatic activity. AZINs are able to bind AZs even more efficiently than ODC, releasing ODC from the inhibitory ODC-AZ complex (84, 85). AZINs are also able to enhance the uptake of extracellular polyamines, likely by negating the inhibitory action of AZs on the polyamine transport system (86). In addition, AZs and AZINs can also modulate the uptake of agmatine by mammalian cells (87).
Absorption of Polyamines in the Gastrointestinal Tract
The polyamines present in the lumen of the gastrointestinal tract may have different origin: food, intestinal microbiota, pancreatic-biliary secretions, and intestinal death cells. Although a precise quantitation of the contribution of each process to the whole polyamine pool is non-existent, it is believed that dietary polyamines is the major source of luminal polyamines in humans and animals (88, 89). Polyamine levels have been analyzed in hundreds of food items by different groups (49, 88, 90–93). In general, fruits and cheese are rich in putrescine, whereas vegetables and meat products contain high levels of spermidine and spermine, respectively (94). Despite the high variability of the polyamine content in foods, it has been estimated that a standard human diet provides hundreds of micromoles of polyamines per day (88), with small differences between the major polyamines when it is calculated from diets from different countries (95).
The polyamine levels in the intestinal lumen change over time after a meal, from millimolar levels immediately after the ingestion, to much lower values in the fasting period (96). Luminal polyamines can be taken up mainly by the small intestine. Experiments using radiolabeled polyamines administered intragastrically to rats showed that polyamines can be absorbed and distributed to different tissues (88). This distribution was not uniform, since polyamines were accumulated preferentially in those tissues stimulated to proliferate (88). The first barrier for the uptake of polyamines from the intestinal lumen is formed by the epithelial cells of the intestinal mucosa. In isolated enterocytes, as in other mammalian cells in culture, polyamines can be taken up by different specific polyamine transporters, as already commented in the polyamine transport section. The in vivo polyamine uptake by the intestinal cells is more complex due to the existence of different polyamine transporters in the apical and basolateral membranes, as shown by studies using brush-border and basolateral membrane vesicles of the enterocyte (97). According with experimental data, luminal polyamines could be taken by enterocytes by transport across the apical membrane and extruded across the basolateral membrane by low affinity transporters to the systemic circulation (96). It was also hypothesized that the majority of luminal polyamines could be passively absorbed via the paracellular route (96). Whereas, most of spermidine and spermine taken up by the intestinal cells are not metabolized in these cells, a variable proportion of putrescine is transformed into other compounds including spermidine, γ-aminobutyric acid (GABA) and succinate (88, 98). In the small intestine of rats, putrescine can be transformed into succinate acting as a source of instant energy (99). The absorption of polyamines appears to be rapid, since experiments using an ex vivo rat model revealed that values about 70% of the 14C-polyamines administered to the jejunal lumen were found in the portal vein, after 10 min of polyamine administration (100). Most of the studies on luminal polyamine uptake and their distribution through the body have been based on the acute administration of a low dose of labeled polyamines to rats. Recently, as described in other section, many studies have reported beneficial effects of a prolonged oral administration of either spermidine or spermine to rodents (101–104). However, in most studies tissue polyamine levels were not reported. In mouse models, prolonged administration of polyamine-rich diets have been seen to increase blood levels of spermidine and/or spermine (56, 105, 106). In aged mice spermidine levels significantly increased in blood (107) and liver (101) after supplementation of the drinking water with 3 mM spermidine for 6 months. In line with this, a 28-day oral supplementation of adult mice with 50 mg/kg of spermidine resulted in a significant increase of spermidine in whole blood and heart (but not in brain) of females, but not in males (106). In humans it has been shown that a prolonged intake (2 months) of polyamine-rich products such as natto (fermented soy) produces a significant rise in the levels of spermine (but not spermidine) in blood (56). More recently, the results of a clinical trial using spermidine supplements in older human subjects have been reported, showing no differences in blood polyamine levels between controls and spermidine-supplemented individuals at 3 months of follow-up (106)s.
A part of the absorbed luminal polyamines remains in the intestinal cells. This is not surprising since the intestinal tissue is one of the most rapidly proliferating tissues, and in general the polyamine uptake of intestinal cells is associated with the proliferative stage. Dietary polyamines might be important for the development of the digestive tract, and also for the maintenance of the adult digestive tract (108). Moreover, polyamine reservoirs are not only responsible for maintaining the rapid turnover and high proliferation rates of the intestinal epithelial cells but also for enhancing the integrity of the intestinal barrier. Thus, polyamines are able to stimulate the production of intercellular junction proteins, such as occludin, zonula occludens 1, and E-cadherin, which are essentials to regulate the paracellular permeability and reinforcing epithelial barrier function (109).
Furthermore, intestinal polyamine pools are necessary for the postnatal development of the gastrointestinal tract. These data were confirmed in a study using pup rats, where the polyamine administration was able to induce the production of mucus and secretory IgA in the small intestine, while rats fed with a polyamine-deficient diet developed intestinal mucosal hypoplasia (110). In addition, the oral administration of polyamines to suckling rats accelerated the maturation process affecting intestine, liver and pancreas. Interestingly, the precocious maturation of the intestine only was achieved when spermine was given orally and not when other routes of administration were used, suggesting that this process requires the interaction of the polyamine with the luminal side of the mucosa (111). On the other hand, although it has been postulated that the polyamines present in the human milk, could contribute to prevent food allergic processes in neonates, more studies appear to be necessary (112).
As commented above, antizymes and antizyme inhibitors play a relevant role in cellular polyamine uptake. However, the influence of these proteins on intestinal polyamine absorption is uncertain. Both ODC and AZ1 mRNA transcripts are abundantly expressed in small intestine (113). Treatment of the intestinal epithelial cell line IEC-6 with 10 μM of spermidine or spermine induced the synthesis of the antizyme protein negatively affecting polyamine uptake (114). In this cell line AZ1 is induced in the absence of amino acids, through a mechanism involving mTORC1 (115). Other studies using a human colon adenocarcinoma cell line (Caco-2) revealed that amino acid supplementation also modulates antizyme induction (116), whereas amino acid restriction increased spermidine uptake by Caco-2 cells, by an antizyme-independent mechanism (117). The levels of AZ mRNA have been analyzed in cells isolated from jejunal crypt-villus axis. Whereas, AZ mRNA levels were high in cells from the small intestinal crypts, the message fell to near undetectable levels in cells of the villus tip (118). However, since the expression of AZ protein was not analyzed, and it is well-known that the translation of the AZ mRNA is stimulated by polyamines, no firm conclusion about polyamine uptake though the crypt-villus axis could be established. Another uncertain question is how antizyme inhibitors may affect gut polyamine absorption. In comparison to ODC and AZ1, mRNA expression levels of AZ2, AZIN1, and AZIN2 in mouse intestine are low (113). Whereas, in normal cells AZIN1 stimulates both polyamine biosynthesis and uptake by binding to AZs, an edited form of AZIN1 with higher affinity to AZs has aroused more attention because of its contribution to carcinogenesis (119). In this regard, AZIN1 RNA editing levels were found significantly elevated in colorectal cancer tissues when compared with corresponding normal mucosa (120). It is conceivable that tissues with enhanced AZIN1 activity could accumulate higher levels of polyamines than normal ones, due to the loss of the feedback effect of polyamines by the blockade of AZ function.
Polyamines and Gut Microbiota
The quantity and diversity of microbial species in the gut increase longitudinally from the stomach to the colon, being the colon where the most dense and metabolically active community exist (121). The gut microbiome produces a diverse metabolite repertoire that may harm or benefit the host. These gut microbial metabolites include short-chain fatty acids, polyphenols, vitamins, tryptophan catabolites, and polyamines (122, 123). A recent study has provided data on polyamine biosynthesis and transport in the dominant human gut bacteria (124). As described above the high levels of polyamines present in the intestinal tract may be originated from the diet or produced de novo by host cells and intestinal bacteria (125) (Figure 3). Nevertheless, it is thought that the greatest amounts of the polyamines present in the lower parts of the intestinal tract could be mostly synthesized by intestinal microbiota. A metabolomics study in mice revealed that the intestinal luminal levels of putrescine and spermidine, but not of spermine, are mainly dependent on colonic microbiota (122). In addition, experiments in rats using different dietary interventions demonstrated that the oral administration of fermentable fibers and fructans stimulates certain intestinal microbial species (i.e., bacteroides and fusobacteria) to synthetize large amounts of polyamines in the large intestine (126–128). It is believed that polyamines synthesized by the colonic microflora are transported to the proximal gut via the portal circulation and biliary tree (129), although the contribution of colonic microflora to circulating levels of polyamines in the host is presently unknown. It is likely that part of the polyamines produced by the colonic microbiota is excreted in the feces. In this regard, it was reported that human fecal polyamine concentration may be linked to fecal microbiota (130).
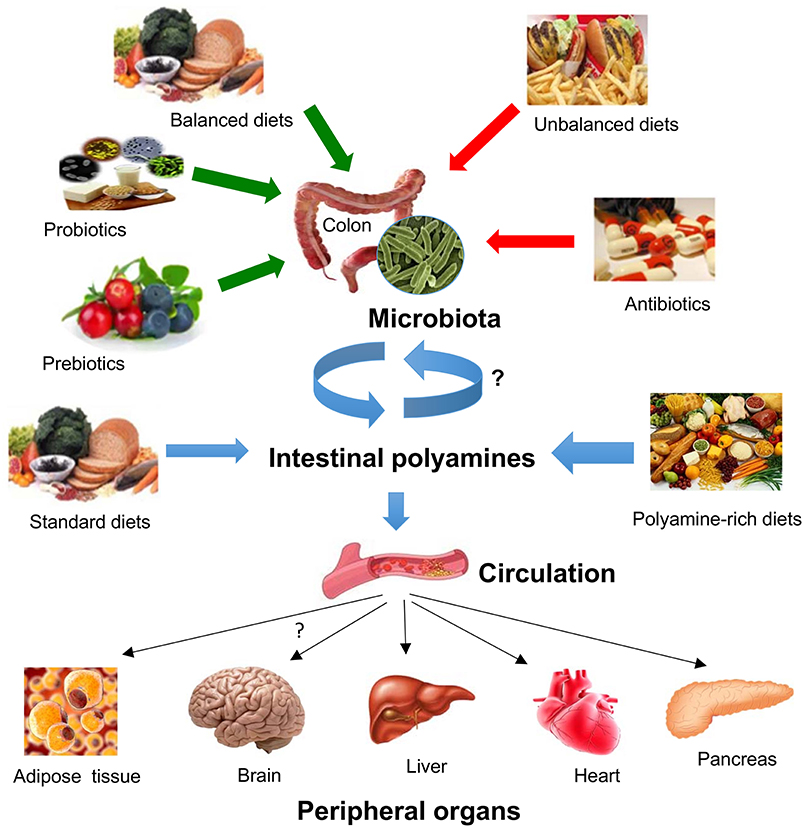
Figure 3. Impact of diet and gut microbiota on polyamine-mediated effects in peripheral organs. Gut microbiota composition and microbial polyamine production are affected by several conditions such as the intake of balanced/unbalanced diets, the consumption of prebiotics/probiotics, and antibiotics. Intestinal polyamines levels depends on their uptake from the gut microbiota or from different dietary conditions, which in turn it can be transported into the circulation where they can target various peripheral organs including adipose tissue, brain, liver, heart, or endocrine pancreas.
Unlike the host polyamine metabolism, gut microorganisms can produce polyamines using constitutive or inducible forms of amino acid decarboxylase enzymes. Ornithine, lysine, and arginine decarboxylase activities have been detected in bacteria and archea (5). In addition, spermidine can be produced from putrescine and dcAdoMet by spermidine synthase, although some microorganisms can synthesize this polyamine from putrescine using L-aspartate β-semialdehyde as aminopropyl donor (5). This alternative biosynthetic route of spermidine is dominant in human gut microbiota (131). Arginine decarboxylation is also the dominant pathway for diamine biosynthesis in the most abundant species of the human intestinal microbiome (132). Interestingly, oral administration of arginine to mice after treatment with antibiotics did not result in increased putrescine levels in the intestinal tract, indicating that putrescine could be predominantly produced by intestinal bacteria (133). In addition, it has been demonstrated by using stable isotope labeled arginine that colonic luminal putrescine was produced by intestinal bacteria from arginine metabolism in a dose-dependent manner (133). On the other hand, the arginine deiminase pathway (an anaerobic route for arginine degradation) is common in gut commensal bacteria such as Enterococcus, Streptococcus, Clostridium, Lactococcus, and Lactobacillus species. However, whereas some of these species cannot produce putrescine because of the lack of essential polyamine biosynthetic enzymes, they acquire it from other bacterial species through a collective biosynthetic pathway catalyzed by enzymes derived from multiple bacterial species and from several extracellular intermediates (134).
The metabolism of polyamines has a central role in the regulation of systemic and mucosal adaptive immunity. Arginine is an important modulator of the immunometabolism of macrophages and T cells able to affect their effector functions (125). Thus, the metabolism of dietary arginine by the gut microbiome might have effects on the immune system (135). In this regard, mice colonized with specific microbial communities significantly contain lower amounts of arginine in their intestines compared to germ-free mice, which has been attributed to the microbiome capacity of metabolizing important amounts of arginine from the diet in the gut and, therefore, regulating the availability of arginine for the immune system (136). Furthermore, polyamines inhibit the production of inflammatory cytokines and have antioxidant effects. In fact, exogenous polyamines can decrease the release cytokines that promotes epithelial repair and barrier function. Similarly, both spermine and histamine inhibit the activation of the NLRP6 inflammasome, which is a protein complex expressed by epithelial cells that can regulate IL-18 secretion (137, 138). On the other hand, a previous study using healthy mice, described that the presence of the probiotic strain Bifidobacterium animalis subsp. lactis LKM512 can induce resistance to oxidative stress and promote longevity, which was dependent on enhanced microbial polyamine synthesis (139).
Dysregulated polyamine metabolism has been suggested to be involved in the tumorigenesis of colorectal cancer (CRC) and other tumors (29). A metabolomics screen comparing paired colon cancer and normal tissue samples from patients with CRC revealed that bacteria biofilm formation, even in the normal colon tissue, was associated with increased colonic epithelial cell proliferation and host-enhanced polyamine metabolism (140). In addition, bacteria-generated polyamines in biofilms may contribute to the inflammation and proliferation of colon cancer (141). Following antibiotic treatment, resected colorectal cancer tissues harbored disrupted bacterial biofilms and lowered N1,N12-diacetylspermine tissue concentrations compare to biofilm-negative colon cancer tissues, suggesting that gut microbes can induce an increase of host generated N1,N12-diacetylspermine (141). In tumor-bearing mice, the administration of antibiotics that reduces the microbial flora of the gastrointestinal tract enhanced the cytostatic effect of difluoromethyl ornithine (DFMO), a potent inhibitor of endogenous polyamine synthesis (142, 143), suggesting that reduction of bacterial polyamine synthesis together with the inhibition of the polyamine biosynthesis route should be considered as an effective antitumoral strategy.
Polyamines in Obesity and Related Metabolic Disorders
In several transgenic mouse models, the dysregulation of the polyamine metabolism has been shown to have an impact in the regulation of the glucose, lipid, and energy homeostasis (144–149). In fact, emerging evidence suggests that increased levels of polyamines in white adipose tissue, liver or skeletal muscle could stimulates energy expenditure and confer resistance to diet-induced obesity and non-alcoholic fatty liver disease (147, 148). In addition, a number of studies using different obesity animal models have reported altered levels of polyamines in adipose tissue (150), liver (151, 152), pancreatic islets (153), and urine (154). In addition, blood polyamines have been shown to be significantly higher in obese children compared to non-obese controls (155). Remarkably, polyamine metabolism has been involved in adipogenesis (156–158), suggesting that increased polyamine levels may be implicated in adipose tissue expandability during obesity. Particularly, in 3T3-L1 preadipocytes both spermidine and spermine are essential factors at early stages of adipocyte differentiation as they modulate the expression levels of transcriptional factors implicated in the regulation of adipogenesis (18, 157, 159).
In diet-induced obesity mouse models, a high-dose daily administration of either spermidine or spermine has been shown to be an effective strategy for weight loss and improvement of the glycemic status (103, 160, 161). For instance, spermidine supplementation resulted in a significant decrease in body weight, increased glucose tolerance and insulin sensitivity and ameliorated hepatic steatosis in high-fat diet-induced obese mice (161). Furthermore, treatment with exogenous spermine has been shown to be effective to decrease body weight and fasting glucose and to improve glucose tolerance in diet-induced obese mice (160). In cultured rat adipocytes, spermine has been shown to enhance glucose transport and the conversion of glucose into triacylglycerols (162), and to increase the ability of insulin to bind to its own receptor (163). Finally, spermidine administration was able to prevent lipid accumulation and necrotic core formation in vascular smooth muscle cells through the induction of cholesterol efflux in an experimental model of atherosclerosis (164).
Despite the fact that several studies have indicated that polyamine metabolism could be dysregulated in obesity, the role of polyamines in type 2 diabetes (T2D), which is frequently associated with obesity, has been less explored. In pancreatic islets, polyamines are mainly located in the secretory granules of the β cells (52), where they have been implicated in proinsulin biosynthesis and insulin secretion (165). Islet polyamine levels diminished with age and obesity (153), suggesting that alterations in the intracellular levels of polyamines might affect β cell function. Remarkably, transgenic mice overexpressing the polyamine catabolic enzyme spermidine/spermine acetyltransferase (SSAT), which contain higher levels of putrescine and reduced levels of spermidine and spermine in the pancreatic islets, display impaired glucose-stimulated insulin secretion (146), suggesting that disturbed levels of polyamines may be involved in the physiopathology of T2D. In this regard, it has been recently reported an association between the serum levels of polyamines and T2D in a cohort of patients with metabolic syndrome (166). In particular, it was found that serum putrescine levels were significantly elevated in diabetic subjects and that correlated with glycosylated hemoglobin, and that serum spermine was closely associated with insulin levels (166). In animal models of diabetes, the polyamine biosynthetic enzyme ODC has been described to be upregulated in rat diabetic kidneys, resulting in increased levels of putrescine but not other polyamines (167).
On the other hand, exogenous administration of spermidine in rats with pharmacologically induced diabetes results in an improvement of glycaemia and a concomitant reduction of glycosylated HbA1c levels (168). Furthermore, spermine administration has been shown to be effective to protect β cells against the diabetogenic effect of alloxan (169). In diabetic rats, spermine administration did not affect hyperglycemia but improved the lipid profile and lead to a reduction in the formation of advanced glycation end-products (170). Finally, spermidine could improve endothelial dysfunction in diabetes by restoring nitric oxide production in an autophagy-dependent fashion (171).
Like polyamines, agmatine has significant metabolic effects when exogenously administered in animal models. For instance, the chronic administration of agmatine resulted in a reduction body weight gain and fat depots in rats fed a high-fat diet (172). Remarkably, this study further demonstrated that the effects of long-term agmatine consumption on body weight and adiposity could be due to the regulation of fat oxidation and gluconeogenesis (172). Additionally, recent studies have reported that exogenous agmatine administration significantly reduces atherosclerotic lesions in ApoE knockout mice (173), although the mechanism by which agmatine exerts these anti-atherosclerotic effect remains to be elucidated.
Numerous works have demonstrated that both oxidative stress and chronic inflammation are factors attributed to obesity that can contribute to the development of metabolic syndrome. Polyamines can act of modulators of oxidative stress and inflammation (174). However, the possible impact polyamines in these processes in the context of obesity have not been explored yet. Gut microbiota is another well-known factor involved in the pathogenesis of obesity and T2D. In fact, recent insight suggests that an altered composition and diversity of gut microbiota could play an important role in the development of metabolic disorders. Gut microbiota dysbiosis is characterized by metabolic endotoxemia, abnormal incretin secretion and production of bacterial metabolites that can influence energy and glucose metabolism (175). As described above polyamines can be produced by several bacterial strains in the gut, although the potential therapeutic use of polyamines derived from the gut microbiota in the treatment of metabolic disorders remains to be investigated.
Dietary Polyamines in Aging and Cancer
Several studies have shown that the exogenous addition of polyamines, especially spermidine, increases the lifespan in yeast, nematodes, and fruit flies (101, 176). In rodents, a progressive decrease in the tissue polyamine content has been observed with age (49, 177), and the chronic administration of spermidine extends lifespan (107, 178, 179). Likewise, in animal models, supplementation with polyamines could have a beneficial effect on several age-related disorders including memory decline, neuroinflammation, and cardiovascular disease (107, 180–182). Several in vitro studies have shown that the administration of spermidine in cell lines of neuronal origin inhibits the process of cellular senescence (183, 184). It has been proposed that the mechanism by which polyamines produces these beneficial anti-aging effects could be through the activation of autophagy (34, 101), either through the inhibition of the activity of the acetyltransferase EP300, which is involved in the modulation of autophagic flux (185–187), or the stabilization of pro-autophagic factors such as MAP1S (179).
In addition to the exogenous supply of synthetic polyamines, several studies have shown that an increase in the concentration of gut polyamines through the administration of probiotics inhibits cellular senescence and stimulates longevity in mice (133, 139). In humans, recent epidemiological studies have associated a higher consumption of dietary spermidine with a decrease in cardiovascular events and lower mortality (107, 188). Remarkably, many food products included in the Mediterranean diet, which is known to be associated with increased longevity (189), are rich in polyamines (94, 190). However, whether a greater adherence to a Mediterranean dietary pattern increases circulating polyamine levels remains unknown.
On the other hand, in parallel with the progressive reduction of tissue polyamine levels, many works have reported alterations in the DNA methylation status associated with age (191, 192). Noteworthy, the polyamine pathway is connected to the methionine cycle through the action of AdoMetDC, which is able to decarboxylate AdoMet. In turn, AdoMet is the major donor of methyl groups for nucleic acids or other macromolecules. Thus, it has been proposed that lower spermidine and spermine levels can lead to reduced DNA methylation levels by inducing the accumulation of dcAdoMet, which it could be able to inhibit DNA methyltransferase (DNMT) activity (193). In accordance, in vitro experiments consisting in the pharmacological or genetic depletion of polyamine pools in human cell lines resulted in changes in DNA methylation (105, 194, 195). Moreover, abnormalities in the global DNA methylation status associated with aging can be reversed by exogenous polyamine supply (105), supporting the idea that the reduction of tissue polyamines during aging is closely related to alterations in DNA methylation. Remarkably, an abnormal DNA methylation profile has been related not only with aging, but also with other pathologies including cancer and metabolic disorders (196, 197). Interestingly, adherence to a Mediterranean dietary pattern has been described to be associated with changes in the DNA methylation status (198, 199). However, whether these effects are mediated by polyamines or other metabolites related to one carbon metabolism remain to be elucidated.
Despite the fact that emerging evidence is proposing polyamines as therapeutic option for aging-related human events including cardiovascular disease or memory decline, the pro-carcinogenic potential of polyamines should be carefully considered when polyamine-rich diets are therapeutically recommended. It is widely accepted that polyamine production is abnormally stimulated in different types of human tumors by induction of the polyamine biosynthetic pathway, which could be beneficial for tumor progression (200). Many efforts have been directed to limit the polyamine pools in the tumor by administrating chemical inhibitors of the polyamine biosynthetic enzyme ODC (i.e., DFMO), although this therapeutic option frequently has failed due to the capacity of tumor cells of replacing endogenously synthetized polyamines by extracellular polyamines from the circulation. Hence, restriction of dietary polyamines has been proposed to enhance the effects of the inhibitors of the polyamine synthesis. In CRC patients with advanced adenoma it has been proposed that the limitation of dietary polyamines could be an adjunctive strategy to avoid recurrence after 3 years of treatment using polyamine-inhibitory drugs (201). By contrast, it has been suggested that dietary polyamines might decrease the risk of CRC in postmenopausal women (202). This is supported by experiments in mice that demonstrated that polyamine intake could be an effective strategy to prevent colon tumors after 1,2-demethylhydrazine administration (105). In rats, whereas the treatment with a low spermidine diet can promote the induction of mammary tumors, a high spermidine diet may suppress tumorigenesis (203). Additionally, it has been recently reported that polyamine accumulation could have toxic effects for renal cell carcinoma and that the tumor cells avoid polyamine toxicity by inducing arginase 2 (204). Therefore, although polyamines have been classically considered as oncometabolites by its procarcinogenic actions once the tumor is formed, these new studies could indicate that dietary polyamines could be considered for cancer prevention or even for cancer treatment depending on the cancer type in a dose-dependent fashion. However, because of the insufficient number of studies performed in vivo with exogenously administrated polyamines in mouse cancer models, caution is necessary before considering the ingestion of polyamines as an anticancer strategy in humans.
Concluding Remarks
Although it is well-known that the polyamine content in mammalian tissues is tightly controlled through a complex regulatory network involving the modulation of the biosynthesis, catabolism, and transport pathways, the tissue and circulating levels of polyamines are altered in a number of pathological conditions. Whereas, numerous experiments have demonstrated that inhibitors of the polyamine biosynthetic pathway drastically decrease the tissue levels of putrescine and spermidine, less is known about the effect of either the restriction or the administration of exogenous polyamines on tissue and circulating polyamine levels. Interestingly, recent studies have revealed that oral spermidine supplementation extended the lifespan and has cardioprotective effects in different animal models, but the detailed molecular mechanisms by which spermidine exert these effects are only partially understood. In humans, nutritional studies have described a relationship between spermidine-rich foods and lower cardiovascular events and reduced mortality, but in both cases, no evidence about the tissue or circulating levels of spermidine has been reported. More recently, chronic interventions of elderly subjects with controlled amounts of spermidine have revealed increased levels of this polyamine in blood after several months of treatment. Other studies have demonstrated that prolonged consumption of natto, a traditional Japanese fermented food rich in polyamines, significantly increases spermine, but not spermidine in blood of healthy young volunteers. Because of the beneficial effects of polyamine intake recently reported, it would be interesting to know how the dietary polyamine supplementation might affect polyamine levels in different organs and tissues and why this variation induces all the reported effects.
On the other hand, emerging body of evidence has revealed that in diet-induced obesity models the daily administration of high doses of polyamines (either spermidine or spermine) can reduce body weight and adiposity and can positively regulate glucose homeostasis, suggesting that exogenous polyamines can impact peripheral organs with relevant implications in lipid and glucose metabolism such as adipose tissue and liver (Figure 3). In pharmacological models of T2D, polyamines could also exert protective effects in pancreatic β cells. These effects, however, have not been proven after the oral intake of low concentrations of polyamines with demonstrated effects on longevity or heart protection. Therefore, the impact of a chronic low-dose oral polyamine intake should be performed in models of obesity or T2D before considering polyamines as therapeutic option for these metabolic disorders.
Author Contributions
All authors: conceptualization, writing review, and editing; RP, BR-M, and MQ-O: writing original draft; RP and BR-M funding.
Funding
This study was supported by research grants from the Andalusian Health Public System (grant number PI-0096-2017) and by the Spanish Ministry of Economy and Competitiveness, SAF2011-29051 (with European Community FEDER support) and by Seneca Foundation (Autonomous Community of Murcia), 19875/GERM/15. MQ-O was supported by the Miguel Servet Type II program (CPII18/00003) from the Institute of Health Carlos III (ISCIII), and co-financed by the European Regional Development Fund (ERDF), and by the Andalusian Health Public System (C-0030-2018). BR-M was recipient of a Sara Borrell postdoctoral fellowship from the ISCIII (CD16/0003), co-financed by the European Regional Development Fund (ERDF).
Conflict of Interest Statement
The authors declare that the research was conducted in the absence of any commercial or financial relationships that could be construed as a potential conflict of interest.
The reviewer MA declared a shared affiliation, with no collaboration, with several of the authors, BR-M, MQ-O, FT, to the handling editor at time of review.
References
1. Tabor CW, Tabor H. Polyamines. Annu Rev Biochem. (1984) 53:749–90. doi: 10.1146/annurev.bi.53.070184.003533
4. Takahashi T, Kakehi J. Polyamines: ubiquitous polycations with unique roles in growth and stress responses. Ann Bot. (2010) 105:1–6. doi: 10.1093/aob/mcp259
5. Michael AJ. Biosynthesis of polyamines and polyamine-containing molecules. Biochem J. (2016) 473:2315–29. doi: 10.1042/BCJ20160185
6. Oshima T. Enigmas of biosyntheses of unusual polyamines in an extreme thermophile, Thermus thermophilus. Plant Physiol Biochem. (2010) 48:521–6. doi: 10.1016/j.plaphy.2010.03.011
7. Pegg AE, McGill S. Decarboxylation of ornithine and lysine in rat tissues. Biochim Biophys Acta. (1979) 568:416–27. doi: 10.1016/0005-2744(79)90310-3
8. Raasch W, Regunathan S, Li G, Reis DJ. Agmatine, the bacterial amine, is widely distributed in mammalian tissues. Life Sci. (1995) 56:2319–30. doi: 10.1016/0024-3205(95)00226-V
9. Pegg AE. Recent advances in the biochemistry of polyamines in eukaryotes. Biochem J. (1986) 234:249–62. doi: 10.1042/bj2340249
10. Pegg AE. Mammalian polyamine metabolism and function. IUBMB Life. (2009) 61:880–94. doi: 10.1002/iub.230
11. Bae DH, Lane DJR, Jansson PJ, Richardson DR. The old and new biochemistry of polyamines. Biochim Biophys Acta Gen Subj. (2018) 1862:2053–68. doi: 10.1016/j.bbagen.2018.06.004
12. Igarashi K, Kashiwagi K. Polyamines: mysterious modulators of cellular functions. Biochem Biophys Res Commun. (2000) 271:559–64. doi: 10.1006/bbrc.2000.2601
13. Schuster I, Bernhardt R. Interactions of natural polyamines with mammalian proteins. Biomol Concepts. (2011) 2:79–94. doi: 10.1515/bmc.2011.007
14. Bachrach U, Wang YC, Tabib A. Polyamines: new cues in cellular signal transduction. News Physiol Sci. (2001) 16:106–9. doi: 10.1152/physiologyonline.2001.16.3.106
15. Janne J, Alhonen L, Pietila M, Keinanen TA. Genetic approaches to the cellular functions of polyamines in mammals. Eur J Biochem. (2004) 271:877–94. doi: 10.1111/j.1432-1033.2004.04009.x
16. Landau G, Bercovich Z, Park MH, Kahana C. The role of polyamines in supporting growth of mammalian cells is mediated through their requirement for translation initiation and elongation. J Biol Chem. (2010) 285:12474–81. doi: 10.1074/jbc.M110.106419
17. Landau G, Ran A, Bercovich Z, Feldmesser E, Horn-Saban S, Korkotian E, et al. Expression profiling and biochemical analysis suggest stress response as a potential mechanism inhibiting proliferation of polyamine-depleted cells. J Biol Chem. (2012) 287:35825–37. doi: 10.1074/jbc.M112.381335
18. Brenner S, Bercovich Z, Feiler Y, Keshet R, Kahana C. Dual regulatory role of polyamines in adipogenesis. J Biol Chem. (2015) 290:27384–92. doi: 10.1074/jbc.M115.686980
19. Zwighaft Z, Aviram R, Shalev M, Rousso-Noori L, Kraut-Cohen J, Golik M, et al. Circadian clock control by polyamine levels through a mechanism that declines with age. Cell Metab. (2015) 22:874–85. doi: 10.1016/j.cmet.2015.09.011
20. Miller-Fleming L, Olin-Sandoval V, Campbell K, Ralser M. Remaining mysteries of molecular biology: the role of polyamines in the cell. J Mol Biol. (2015) 427:3389–406. doi: 10.1016/j.jmb.2015.06.020
21. Cason AL, Ikeguchi Y, Skinner C, Wood TC, Holden KR, Lubs HA, et al. X-linked spermine synthase gene (SMS) defect: the first polyamine deficiency syndrome. Eur J Hum Genet. (2003) 11:937–44. doi: 10.1038/sj.ejhg.5201072
22. Bupp CP, Schultz CR, Uhl KL, Rajasekaran S, Bachmann AS. Novel de novo pathogenic variant in the ODC1 gene in a girl with developmental delay, alopecia, and dysmorphic features. Am J Med Genet. (2018) 176, 2548–53. doi: 10.1002/ajmg.a.40523
23. Russell D, Snyder SH. Amine synthesis in rapidly growing tissues: ornithine decarboxylase activity in regenerating rat liver, chick embryo, and various tumors. Proc Natl Acad Sci USA. (1968) 60:1420–7. doi: 10.1073/pnas.60.4.1420
24. Pegg AE. Polyamine metabolism and its importance in neoplastic growth and a target for chemotherapy. Cancer Res. (1988) 48:759–74.
25. Morrison LD, Kish SJ. Brain polyamine levels are altered in Alzheimer's disease. Neurosci Lett. (1995) 197:5–8. doi: 10.1016/0304-3940(95)11881-V
26. Lewandowski NM, Ju S, Verbitsky M, Ross B, Geddie ML, Rockenstein E, et al. Polyamine pathway contributes to the pathogenesis of Parkinson disease. Proc Natl Acad Sci USA. (2010) 107:16970–5. doi: 10.1073/pnas.1011751107
27. Limon A, Mamdani F, Hjelm BE, Vawter MP, Sequeira A. Targets of polyamine dysregulation in major depression and suicide: activity-dependent feedback, excitability, and neurotransmission. Neurosci Biobehav Rev. (2016) 66:80–91. doi: 10.1016/j.neubiorev.2016.04.010
28. Gerner EW, Meyskens FL Jr. Polyamines and cancer: old molecules, new understanding. Nat Rev Cancer. (2004) 4:781–92. doi: 10.1038/nrc1454
29. Casero RA Jr, Murray Stewart T, Pegg AE. Polyamine metabolism and cancer: treatments, challenges and opportunities. Nat Rev Cancer. (2018) 18:681–95. doi: 10.1038/s41568-018-0050-3
30. Tersey SA, Colvin SC, Maier B, Mirmira RG. Protective effects of polyamine depletion in mouse models of type 1 diabetes: implications for therapy. Amino Acids. (2014) 46:633–42. doi: 10.1007/s00726-013-1560-7
31. LoGiudice N, Le L, Abuan I, Leizorek Y, Roberts SC. Alpha-difluoromethylornithine, an irreversible inhibitor of polyamine biosynthesis, as a therapeutic strategy against hyperproliferative and infectious diseases. Med Sci. (2018) 6:12. doi: 10.3390/medsci6010012
32. Minois N, Carmona-Gutierrez D, Madeo F. Polyamines in aging and disease. Aging. (2011) 3:716–32. doi: 10.18632/aging.100361
33. Minois N. Molecular basis of the 'anti-aging' effect of spermidine and other natural polyamines - a mini-review. Gerontology. (2014) 60:319–26. doi: 10.1159/000356748
34. Madeo F, Eisenberg T, Pietrocola F, Kroemer G. Spermidine in health and disease. Science. (2018) 359:eaan2788. doi: 10.1126/science.aan2788
35. Pendeville H, Carpino N, Marine JC, Takahashi Y, Muller M, Martial JA, et al. The ornithine decarboxylase gene is essential for cell survival during early murine development. Mol Cell Biol. (2001) 21:6549–58. doi: 10.1128/MCB.21.19.6549-6558.2001
36. Nishimura K, Nakatsu F, Kashiwagi K, Ohno H, Saito T, Igarashi K. Essential role of S-adenosylmethionine decarboxylase in mouse embryonic development. Genes Cells. (2002) 7:41–7. doi: 10.1046/j.1356-9597.2001.00494.x
37. Igarashi K, Kashiwagi K. Modulation of cellular function by polyamines. Int J Biochem Cell Biol. (2010) 42:39–51. doi: 10.1016/j.biocel.2009.07.009
38. Pasini A, Caldarera CM, Giordano E. Chromatin remodeling by polyamines and polyamine analogs. Amino Acids. (2014) 46:595–603. doi: 10.1007/s00726-013-1550-9
39. Wang Y, Casero RA Jr. Mammalian polyamine catabolism: a therapeutic target, a pathological problem, or both? J Biochem. (2006) 139:17–25. doi: 10.1093/jb/mvj021
40. Pegg AE. Toxicity of polyamines and their metabolic products. Chem Res Toxicol. (2013) 26:1782–800. doi: 10.1021/tx400316s
41. Khan AU, Mei YH, Wilson T. A proposed function for spermine and spermidine: protection of replicating DNA against damage by singlet oxygen. Proc Natl Acad Sci USA. (1992) 89:11426–7. doi: 10.1073/pnas.89.23.11426
42. Ha HC, Sirisoma NS, Kuppusamy P, Zweier JL, Woster PM, Casero RA Jr. The natural polyamine spermine functions directly as a free radical scavenger. Proc Natl Acad Sci USA. (1998) 95:11140–5. doi: 10.1073/pnas.95.19.11140
43. Rider JE, Hacker A, Mackintosh CA, Pegg AE, Woster PM, Casero RA Jr. Spermine and spermidine mediate protection against oxidative damage caused by hydrogen peroxide. Amino Acids. (2007) 33:231–40. doi: 10.1007/s00726-007-0513-4
44. Pedreno E, Lopez-Contreras AJ, Cremades A, Penafiel R. Protecting or promoting effects of spermine on DNA strand breakage induced by iron or copper ions as a function of metal concentration. J Inorg Biochem. (2005) 99:2074–80. doi: 10.1016/j.jinorgbio.2005.07.005
45. Wang L, Liu Y, Qi C, Shen L, Wang J, Liu X, et al. Oxidative degradation of polyamines by serum supplement causes cytotoxicity on cultured cells. Sci Rep. (2018) 8:10384. doi: 10.1038/s41598-018-28648-8
46. Jaenne J, Raina A, Siimes M. Spermidine and spermine in rat tissues at different ages. Acta Physiol Scand. (1964) 62:352–8. doi: 10.1111/j.1748-1716.1964.tb10433.x
47. Wang X, Ikeguchi Y, McCloskey DE, Nelson P, Pegg AE. Spermine synthesis is required for normal viability, growth, and fertility in the mouse. J Biol Chem. (2004) 279:51370–5. doi: 10.1074/jbc.M410471200
48. Scalabrino G, Ferioli ME. Polyamines in mammalian ageing: an oncological problem, too? A review. Mech Ageing Dev. (1984) 26:149–64. doi: 10.1016/0047-6374(84)90090-3
49. Nishimura K, Shiina R, Kashiwagi K, Igarashi K. Decrease in polyamines with aging and their ingestion from food and drink. J Biochem. (2006) 139:81–90. doi: 10.1093/jb/mvj003
50. Watanabe S, Kusama-Eguchi K, Kobayashi H, Igarashi K. Estimation of polyamine binding to macromolecules and ATP in bovine lymphocytes and rat liver. J Biol Chem. (1991) 266:20803–9.
51. Hoshino K, Momiyama E, Yoshida K, Nishimura K, Sakai S, Toida T, et al. Polyamine transport by mammalian cells and mitochondria: role of antizyme and glycosaminoglycans. J Biol Chem. (2005) 280:42801–8. doi: 10.1074/jbc.M505445200
52. Hougaard DM, Nielsen JH, Larsson LI. Localization and biosynthesis of polyamines in insulin-producing cells. Biochem J. (1986) 238:43–7. doi: 10.1042/bj2380043
53. Garcia-Faroldi G, Rodriguez CE, Urdiales JL, Perez-Pomares JM, Davila JC, Pejler G, et al. Polyamines are present in mast cell secretory granules and are important for granule homeostasis. PLoS ONE. (2010) 5:e15071. doi: 10.1371/journal.pone.0015071
54. Takeuchi T, Harada Y, Moriyama S, Furuta K, Tanaka S, Miyaji T, et al. Vesicular polyamine transporter mediates vesicular storage and release of polyamine from mast cells. J Biol Chem. (2017) 292:3909–18. doi: 10.1074/jbc.M116.756197
55. Casti A, Orlandini G, Reali N, Bacciottini F, Vanelli M, Bernasconi S. Pattern of blood polyamines in healthy subjects from infancy to the adult age. J Endocrinol Invest. (1982) 5:263–6. doi: 10.1007/BF03348334
56. Soda K, Kano Y, Sakuragi M, Takao K, Lefor A, Konishi F. Long-term oral polyamine intake increases blood polyamine concentrations. J Nutr Sci Vitaminol. (2009) 55:361–6. doi: 10.3177/jnsv.55.361
57. Pegg AE. Regulation of ornithine decarboxylase. J Biol Chem. (2006) 281:14529–32. doi: 10.1074/jbc.R500031200
58. Wu H, Min J, Ikeguchi Y, Zeng H, Dong A, Loppnau P, et al. Structure and mechanism of spermidine synthases. Biochemistry. (2007) 46:8331–9. doi: 10.1021/bi602498k
59. Pegg AE, Michael AJ. Spermine synthase. Cell Mol Life Sci. (2010) 67:113–21. doi: 10.1007/s00018-009-0165-5
60. Pegg AE. S-Adenosylmethionine decarboxylase. Essays Biochem. (2009) 46:25–45. doi: 10.1042/bse0460003
61. Casero RA Jr, Pegg AE. Spermidine/spermine N1-acetyltransferase–the turning point in polyamine metabolism. FASEB J. (1993) 7:653–61. doi: 10.1096/fasebj.7.8.8500690
62. Pegg AE. Spermidine/spermine-N(1)-acetyltransferase: a key metabolic regulator. Am J Physiol Endocrinol Metab. (2008) 294:E995–1010. doi: 10.1152/ajpendo.90217.2008
63. Vujcic S, Liang P, Diegelman P, Kramer DL, Porter CW. Genomic identification and biochemical characterization of the mammalian polyamine oxidase involved in polyamine back-conversion. Biochem J. (2003) 370:19–28. doi: 10.1042/bj20021779
64. Vujcic S, Diegelman P, Bacchi CJ, Kramer DL, Porter CW. Identification and characterization of a novel flavin-containing spermine oxidase of mammalian cell origin. Biochem J. (2002) 367:665–75. doi: 10.1042/bj20020720
65. Wang Y, Murray-Stewart T, Devereux W, Hacker A, Frydman B, Woster PM, et al. Properties of purified recombinant human polyamine oxidase, PAOh1/SMO. Biochem Biophys Res Commun. (2003) 304:605–11. doi: 10.1016/S0006-291X(03)00636-3
66. Murray-Stewart T, Wang Y, Goodwin A, Hacker A, Meeker A, Casero RA Jr. Nuclear localization of human spermine oxidase isoforms - possible implications in drug response and disease etiology. FEBS J. (2008) 275:2795–806. doi: 10.1111/j.1742-4658.2008.06419.x
67. Zhu MY, Iyo A, Piletz JE, Regunathan S. Expression of human arginine decarboxylase, the biosynthetic enzyme for agmatine. Biochim Biophys Acta. (2004) 1670:156–64. doi: 10.1016/j.bbagen.2003.11.006
68. Lopez-Contreras AJ, Lopez-Garcia C, Jimenez-Cervantes C, Cremades A, Penafiel R. Mouse ornithine decarboxylase-like gene encodes an antizyme inhibitor devoid of ornithine and arginine decarboxylating activity. J Biol Chem. (2006) 281:30896–906. doi: 10.1074/jbc.M602840200
69. Kanerva K, Makitie LT, Pelander A, Heiskala M, Andersson LC. Human ornithine decarboxylase paralogue (ODCp) is an antizyme inhibitor but not an arginine decarboxylase. Biochem J. (2008) 409:187–92. doi: 10.1042/BJ20071004
70. Piletz JE, Aricioglu F, Cheng JT, Fairbanks CA, Gilad VH, Haenisch B, et al. Agmatine: clinical applications after 100 years in translation. Drug Discov Today. (2013) 18:880–93. doi: 10.1016/j.drudis.2013.05.017
71. Laube G, Bernstein HG. Agmatine: multifunctional arginine metabolite and magic bullet in clinical neuroscience? Biochem J. (2017) 474:2619–40. doi: 10.1042/BCJ20170007
72. Igarashi K, Kashiwagi K. Characteristics of cellular polyamine transport in prokaryotes and eukaryotes. Plant Physiol Biochem. (2010) 48:506–12. doi: 10.1016/j.plaphy.2010.01.017
73. Abdulhussein AA, Wallace HM. Polyamines and membrane transporters. Amino Acids. (2014) 46:655–60. doi: 10.1007/s00726-013-1553-6
74. Seiler N, Delcros JG, Moulinoux JP. Polyamine transport in mammalian cells. An update. Int J Biochem Cell Biol. (1996) 28:843–61. doi: 10.1016/1357-2725(96)00021-0
75. Grundemann D, Hahne C, Berkels R, Schomig E. Agmatine is efficiently transported by non-neuronal monoamine transporters extraneuronal monoamine transporter (EMT) and organic cation transporter 2 (OCT2). J Pharmacol Exp Ther. (2003) 304:810–7. doi: 10.1124/jpet.102.044404
76. Heinen A, Bruss M, Bonisch H, Gothert M, Molderings GJ. Pharmacological characteristics of the specific transporter for the endogenous cell growth inhibitor agmatine in six tumor cell lines. Int J Colorectal Dis. (2003) 18:314–9. doi: 10.1007/s00384-002-0466-8
77. Winter TN, Elmquist WF, Fairbanks CA. OCT2 and MATE1 provide bidirectional agmatine transport. Mol Pharm. (2011) 8:133–42. doi: 10.1021/mp100180a
78. Sala-Rabanal M, Li DC, Dake GR, Kurata HT, Inyushin M, Skatchkov SN, et al. Polyamine transport by the polyspecific organic cation transporters OCT1, OCT2, and OCT3. Mol Pharm. (2013) 10:1450–8. doi: 10.1021/mp400024d
79. Uemura T, Yerushalmi HF, Tsaprailis G, Stringer DE, Pastorian KE, Hawel L III, et al. Identification and characterization of a diamine exporter in colon epithelial cells. J Biol Chem. (2008) 283:26428–35. doi: 10.1074/jbc.M804714200
80. Belting M, Mani K, Jonsson M, Cheng F, Sandgren S, Jonsson S, et al. Glypican-1 is a vehicle for polyamine uptake in mammalian cells: a pivital role for nitrosothiol-derived nitric oxide. J Biol Chem. (2003) 278:47181–9. doi: 10.1074/jbc.M308325200
81. Uemura T, Stringer DE, Blohm-Mangone KA, Gerner EW. Polyamine transport is mediated by both endocytic and solute carrier transport mechanisms in the gastrointestinal tract. Am J Physiol Gastrointest Liver Physiol. (2010) 299:G517–522. doi: 10.1152/ajpgi.00169.2010
82. Murakami Y, Matsufuji S, Kameji T, Hayashi S, Igarashi K, Tamura T, et al. Ornithine decarboxylase is degraded by the 26S proteasome without ubiquitination. Nature. (1992) 360:597–9. doi: 10.1038/360597a0
83. Mitchell JL, Judd GG, Bareyal-Leyser A, Ling SY. Feedback repression of polyamine transport is mediated by antizyme in mammalian tissue-culture cells. Biochem J. (1994) 299:19–22. doi: 10.1042/bj2990019
84. Kahana C. Antizyme and antizyme inhibitor, a regulatory tango. Cell Mol Life Sci. (2009) 66:2479–88. doi: 10.1007/s00018-009-0033-3
85. Ramos-Molina B, Lambertos A, Penafiel R. Antizyme inhibitors in polyamine metabolism and beyond: physiopathological implications. Med Sci. (2018) 6:E89. doi: 10.3390/medsci6040089
86. Lopez-Contreras AJ, Ramos-Molina B, Cremades A, Penafiel R. Antizyme inhibitor 2 (AZIN2/ODCp) stimulates polyamine uptake in mammalian cells. J Biol Chem. (2008) 283:20761–9. doi: 10.1074/jbc.M801024200
87. Ramos-Molina B, Lopez-Contreras AJ, Lambertos A, Dardonville C, Cremades A, Penafiel R. Influence of ornithine decarboxylase antizymes and antizyme inhibitors on agmatine uptake by mammalian cells. Amino Acids. (2015) 47:1025–34. doi: 10.1007/s00726-015-1931-3
88. Bardócz S, Grant G, Brown DS, Ralph A, Pusztai A. Polyamines in food—implications for growth and health. J Nutr Biochem. (1993) 4:66–71. doi: 10.1016/0955-2863(93)90001-D
89. White A, Bardocz S. Estimation of the polyamine body pool: contribution by de novo biosynthesis, diet and luminal bacteria. In: Bardocz S, White A, editors. Polyamines in Health and Nutrition. Aberdeen: Kluwer Academic Publishers; The Rowett Reseach Institute; Greenburn Road Bucksburn (1999). p. 117–27.
90. Kalac P, Krausova P. A review of dietary polyamines: formation, implications for growth and health and occurrence in foods. Food Chem. (2005) 90:219–30. doi: 10.1016/j.foodchem.2004.03.044
91. Kalac P. Biologically active polyamines in beef, pork and meat products: a review. Meat Sci. (2006) 73:1–11. doi: 10.1016/j.meatsci.2005.11.001
92. Cipolla BG, Havouis R, Moulinoux JP. Polyamine contents in current foods: a basis for polyamine reduced diet and a study of its long term observance and tolerance in prostate carcinoma patients. Amino Acids. (2007) 33:203–12. doi: 10.1007/s00726-007-0524-1
93. Nishibori N, Fujihara S, Akatuki T. Amounts of polyamines in foods in Japan and intake by Japanese. Food Chem. (2007) 100:491–7. doi: 10.1016/j.foodchem.2005.09.070
94. Atiya Ali M, Poortvliet E, Stromberg R, Yngve A. Polyamines in foods: development of a food database. Food Nutr Res. (2011) 55:5572. doi: 10.3402/fnr.v55i0.5572
95. Kalac P. Health effects and occurrence of dietary polyamines: a review for the period 2005–mid 2013. Food Chem. (2014) 161:27–39. doi: 10.1016/j.foodchem.2014.03.102
96. Milovic V. Polyamines in the gut lumen: bioavailability and biodistribution. Eur J Gastroenterol Hepatol. (2001) 13:1021–5. doi: 10.1097/00042737-200109000-00004
97. Milovic V, Stein J, Piiper A, Gerhard R, Zeuzem S, Caspary WF. Characterization of putrescine transport across the intestinal epithelium: study using isolated brush border and basolateral membrane vesicles of the enterocyte. Eur J Clin Invest. (1995) 25:97–105. doi: 10.1111/j.1365-2362.1995.tb01533.x
98. Bardocz S. Polyamines in food and their consequences for food quality and human health. Trends Food Sci Technol. (1995) 6:341–6. doi: 10.1016/S0924-2244(00)89169-4
99. Bardocz S, Grant G, Brown DS, Pusztai A. Putrescine as a source of instant energy in the small intestine of the rat. Gut. (1998) 42:24–8. doi: 10.1136/gut.42.1.24
100. Uda K, Tsujikawa T, Fujiyama Y, Bamba T. Rapid absorption of luminal polyamines in a rat small intestine ex vivo model. J Gastroenterol Hepatol. (2003) 18:554–9. doi: 10.1046/j.1440-1746.2003.03020.x
101. Eisenberg T, Knauer H, Schauer A, Buttner S, Ruckenstuhl C, Carmona-Gutierrez D, et al. Induction of autophagy by spermidine promotes longevity. Nat Cell Biol. (2009) 11:1305–14. doi: 10.1038/ncb1975
102. LaRocca TJ, Gioscia-Ryan RA, Hearon CM Jr, Seals DR. The autophagy enhancer spermidine reverses arterial aging. Mech Ageing Dev. (2013) 134:314–20. doi: 10.1016/j.mad.2013.04.004
103. Gao M, Zhao W, Li C, Xie X, Li M, Bi Y, et al. Spermidine ameliorates non-alcoholic fatty liver disease through regulating lipid metabolism via AMPK. Biochem Biophys Res Commun. (2018) 505:93–8. doi: 10.1016/j.bbrc.2018.09.078
104. Yadav M, Parle M, Jindal DK, Sharma N. Potential effect of spermidine on GABA, dopamine, acetylcholinesterase, oxidative stress and proinflammatory cytokines to diminish ketamine-induced psychotic symptoms in rats. Biomed Pharmacother. (2018) 98:207–13. doi: 10.1016/j.biopha.2017.12.016
105. Soda K, Kano Y, Chiba F, Koizumi K, Miyaki Y. Increased polyamine intake inhibits age-associated alteration in global DNA methylation and 1,2-dimethylhydrazine-induced tumorigenesis. PLoS ONE. (2013) 8:e64357. doi: 10.1371/journal.pone.0064357
106. Schwarz C, Stekovic S, Wirth M, Benson G, Royer P, Sigrist SJ, et al. Safety and tolerability of spermidine supplementation in mice and older adults with subjective cognitive decline. Aging. (2018) 10:19–33. doi: 10.18632/aging.101354
107. Eisenberg T, Abdellatif M, Schroeder S, Primessnig U, Stekovic S, Pendl T, et al. Cardioprotection and lifespan extension by the natural polyamine spermidine. Nat Med. (2016) 22:1428–38. doi: 10.1038/nm.4222
108. Deloyer P, Peulen O, Dandrifosse G. Dietary polyamines and non-neoplastic growth and disease. Eur J Gastroenterol Hepatol. (2001) 13:1027–32. doi: 10.1097/00042737-200109000-00005
109. Liu L, Guo X, Rao JN, Zou T, Xiao L, Yu T, et al. Polyamines regulate E-cadherin transcription through c-Myc modulating intestinal epithelial barrier function. Am J Physiol Cell Physiol. (2009) 296:C801–810. doi: 10.1152/ajpcell.00620.2008
110. Loser C, Eisel A, Harms D, Folsch UR. Dietary polyamines are essential luminal growth factors for small intestinal and colonic mucosal growth and development. Gut. (1999) 44:12–6. doi: 10.1136/gut.44.1.12
111. Kaouass M, Deloyer P, Dandrifosse G. Intestinal development in suckling rats: direct or indirect spermine action? Digestion. (1994) 55:160–7.
112. Larque E, Sabater-Molina M, Zamora S. Biological significance of dietary polyamines. Nutrition. (2007) 23:87–95. doi: 10.1016/j.nut.2006.09.006
113. Ramos-Molina B, Lopez-Contreras AJ, Cremades A, Penafiel R. Differential expression of ornithine decarboxylase antizyme inhibitors and antizymes in rodent tissues and human cell lines. Amino Acids. (2012) 42:539–47. doi: 10.1007/s00726-011-1031-y
114. Yuan Q, Ray RM, Viar MJ, Johnson LR. Polyamine regulation of ornithine decarboxylase and its antizyme in intestinal epithelial cells. Am J Physiol Gastrointest Liver Physiol. (2001) 280:G130–138. doi: 10.1152/ajpgi.2001.280.1.G130
115. Ray RM, Viar MJ, Johnson LR. Amino acids regulate expression of antizyme-1 to modulate ornithine decarboxylase activity. J Biol Chem. (2012) 287:3674–90. doi: 10.1074/jbc.M111.232561
116. Chabanon H, Persson L, Wallace HM, Ferrara M, Brachet P. Increased translation efficiency and antizyme-dependent stabilization of ornithine decarboxylase in amino acid-supplemented human colon adenocarcinoma cells, Caco-2. Biochem J. (2000) 348 (Pt 2):401–8. doi: 10.1042/bj3480401
117. Aubel C, Chabanon H, Persson L, Thiman L, Ferrara M, Brachet P. Antizyme-dependent and -independent mechanisms are responsible for increased spermidine transport in amino acid-restricted human cancer cells. Biochem Biophys Res Commun. (1999) 256:646–51. doi: 10.1006/bbrc.1999.0397
118. Gill J, Kirby L, Seidel ER. Antizyme mRNA distribution along the crypt/villus axis and modulation of expression in response to polyamines. Gastroenterology. (1998) 114:A880. doi: 10.1016/S0016-5085(98)83583-X
119. Qiu S, Liu J, Xing F. Antizyme inhibitor 1: a potential carcinogenic molecule. Cancer Sci. (2017) 108:163–9. doi: 10.1111/cas.13122
120. Shigeyasu K, Okugawa Y, Toden S, Miyoshi J, Toiyama Y, Nagasaka T, et al. AZIN1 RNA editing confers cancer stemness and enhances oncogenic potential in colorectal cancer. JCI Insight. (2018) 3:99976. doi: 10.1172/jci.insight.99976
121. Rooks MG, Garrett WS. Gut microbiota, metabolites and host immunity. Nat Rev Immunol. (2016) 16:341–52. doi: 10.1038/nri.2016.42
122. Matsumoto M, Kibe R, Ooga T, Aiba Y, Kurihara S, Sawaki E, et al. Impact of intestinal microbiota on intestinal luminal metabolome. Sci Rep. (2012) 2:233. doi: 10.1038/srep00233
123. Bhat MI, Kapila R. Dietary metabolites derived from gut microbiota: critical modulators of epigenetic changes in mammals. Nutr Rev. (2017) 75:374–89. doi: 10.1093/nutrit/nux001
124. Sugiyama Y, Nara M, Sakanaka M, Gotoh A, Kitakata A, Okuda S, et al. Comprehensive analysis of polyamine transport and biosynthesis in the dominant human gut bacteria: potential presence of novel polyamine metabolism and transport genes. Int J Biochem Cell Biol. (2017) 93:52–61. doi: 10.1016/j.biocel.2017.10.015
125. Postler TS, Ghosh S. Understanding the holobiont: how microbial metabolites affect human health and shape the immune system. Cell Metab. (2017) 26:110–30. doi: 10.1016/j.cmet.2017.05.008
126. Noack J, Kleessen B, Proll J, Dongowski G, Blaut M. Dietary guar gum and pectin stimulate intestinal microbial polyamine synthesis in rats. J Nutr. (1998) 128:1385–91. doi: 10.1093/jn/128.8.1385
127. Delzenne NM, Kok N, Deloyer P, Dandrifosse G. Dietary fructans modulate polyamine concentration in the cecum of rats. J Nutr. (2000) 130:2456–60. doi: 10.1093/jn/130.10.2456
128. Noack J, Dongowski G, Hartmann L, Blaut M. The human gut bacteria Bacteroides thetaiotaomicron and Fusobacterium. varium produce putrescine and spermidine in cecum of pectin-fed gnotobiotic rats. J Nutr. (2000) 130:1225–31. doi: 10.1093/jn/130.5.1225
129. Osborne DL, Seidel ER. Gastrointestinal luminal polyamines: cellular accumulation and enterohepatic circulation. Am J Physiol. (1990) 258(4 Pt 1), G576–584. doi: 10.1152/ajpgi.1990.258.4.G576
130. Matsumoto M, Benno Y. The relationship between microbiota and polyamine concentration in the human intestine: a pilot study. Microbiol Immunol. (2007) 51:25–35. doi: 10.1111/j.1348-0421.2007.tb03887.x
131. Hanfrey CC, Pearson BM, Hazeldine S, Lee J, Gaskin DJ, Woster PM, et al. Alternative spermidine biosynthetic route is critical for growth of Campylobacter jejuni and is the dominant polyamine pathway in human gut microbiota. J Biol Chem. (2011) 286:43301–12. doi: 10.1074/jbc.M111.307835
132. Burrell M, Hanfrey CC, Murray EJ, Stanley-Wall NR, Michael AJ. Evolution and multiplicity of arginine decarboxylases in polyamine biosynthesis and essential role in Bacillus subtilis biofilm formation. J Biol Chem. (2010) 285:39224–38. doi: 10.1074/jbc.M110.163154
133. Kibe R, Kurihara S, Sakai Y, Suzuki H, Ooga T, Sawaki E, et al. Upregulation of colonic luminal polyamines produced by intestinal microbiota delays senescence in mice. Sci Rep. (2014) 4:4548. doi: 10.1038/srep04548
134. Nakamura A, Ooga T, Matsumoto M. Intestinal luminal putrescine is produced by collective biosynthetic pathways of the commensal microbiome. Gut Microbes. (2018) 5:1–13. doi: 10.1080/19490976.2018.1494466
135. O'Neill LA, Kishton RJ, Rathmell J. A guide to immunometabolism for immunologists. Nat Rev Immunol. (2016) 16:553–65. doi: 10.1038/nri.2016.70
136. Mardinoglu A, Shoaie S, Bergentall M, Ghaffari P, Zhang C, Larsson E, et al. The gut microbiota modulates host amino acid and glutathione metabolism in mice. Mol Syst Biol. (2015) 11:834. doi: 10.15252/msb.20156487
137. Levy M, Thaiss CA, Zeevi D, Dohnalova L, Zilberman-Schapira G, Mahdi JA, et al. Microbiota-modulated metabolites shape the intestinal microenvironment by regulating NLRP6 inflammasome signaling. Cell. (2015) 163:1428–43. doi: 10.1016/j.cell.2015.10.048
138. Levy M, Thaiss CA, Elinav E. Metabolites: messengers between the microbiota and the immune system. Genes Dev. (2016) 30:1589–97. doi: 10.1101/gad.284091.116
139. Matsumoto M, Kurihara S, Kibe R, Ashida H, Benno Y. Longevity in mice is promoted by probiotic-induced suppression of colonic senescence dependent on upregulation of gut bacterial polyamine production. PLoS ONE. (2011) 6:e23652. doi: 10.1371/journal.pone.0023652
140. Dejea CM, Wick EC, Hechenbleikner EM, White JR, Mark Welch JL, Rossetti BJ, et al. Microbiota organization is a distinct feature of proximal colorectal cancers. Proc Natl Acad Sci USA. (2014) 111:18321–6. doi: 10.1073/pnas.1406199111
141. Johnson CH, Dejea CM, Edler D, Hoang LT, Santidrian AF, Felding BH, et al. Metabolism links bacterial biofilms and colon carcinogenesis. Cell Metab. (2015) 21:891–7. doi: 10.1016/j.cmet.2015.04.011
142. Hessels J, Kingma AW, Ferwerda H, Keij J, van den Berg GA, Muskiet FA. Microbial flora in the gastrointestinal tract abolishes cytostatic effects of alpha-difluoromethylornithine in vivo. Int J Cancer. (1989) 43:1155–64. doi: 10.1002/ijc.2910430632
143. Quemener V, Blanchard Y, Chamaillard L, Havouis R, Cipolla B, Moulinoux JP. Polyamine deprivation: a new tool in cancer treatment. Anticancer Res. (1994) 14:443–8.
144. Niiranen K, Keinanen TA, Pirinen E, Heikkinen S, Tusa M, Fatrai S, et al. Mice with targeted disruption of spermidine/spermine N1-acetyltransferase gene maintain nearly normal tissue polyamine homeostasis but show signs of insulin resistance upon aging. J Cell Mol Med. (2006) 10:933–45. doi: 10.1111/j.1582-4934.2006.tb00536.x
145. Pirinen E, Kuulasmaa T, Pietila M, Heikkinen S, Tusa M, Itkonen P, et al. Enhanced polyamine catabolism alters homeostatic control of white adipose tissue mass, energy expenditure, and glucose metabolism. Mol Cell Biol. (2007) 27:4953–67. doi: 10.1128/MCB.02034-06
146. Cerrada-Gimenez M, Tusa M, Casellas A, Pirinen E, Moya M, Bosch F, et al. Altered glucose-stimulated insulin secretion in a mouse line with activated polyamine catabolism. Transgenic Res. (2012) 21:843–53. doi: 10.1007/s11248-011-9579-6
147. Kraus D, Yang Q, Kong D, Banks AS, Zhang L, Rodgers JT, et al. Nicotinamide N-methyltransferase knockdown protects against diet-induced obesity. Nature. (2014) 508:258–62. doi: 10.1038/nature13198
148. Bonhoure N, Byrnes A, Moir RD, Hodroj W, Preitner F, Praz V, et al. Loss of the RNA polymerase III repressor MAF1 confers obesity resistance. Genes Dev. (2015) 29:934–47. doi: 10.1101/gad.258350.115
149. Yuan F, Zhang L, Cao Y, Gao W, Zhao C, Fang Y, et al. Spermidine/spermine N1-acetyltransferase-mediated polyamine catabolism regulates beige adipocyte biogenesis. Metabolism. (2018) 85:298–304. doi: 10.1016/j.metabol.2018.04.007
150. Jamdar SC, Cao WF, Samaniego E. Relationship between adipose polyamine concentrations and triacylglycerol synthetic enzymes in lean and obese Zucker rats. Enzyme Protein. (1996) 49:222–30. doi: 10.1159/000468632
151. Yun KU, Ryu CS, Lee JY, Noh JR, Lee CH, Lee HS, et al. Hepatic metabolism of sulfur amino acids in db/db mice. Food Chem Toxicol. (2013) 53:180–6. doi: 10.1016/j.fct.2012.11.046
152. Kwak HC, Kim YM, Oh SJ, Kim SK. Sulfur amino acid metabolism in Zucker diabetic fatty rats. Biochem Pharmacol. (2015) 96:256–66. doi: 10.1016/j.bcp.2015.05.014
153. Sjoholm A, Arkhammar P, Berggren PO, Andersson A. Polyamines in pancreatic islets of obese-hyperglycemic (ob/ob) mice of different ages. Am J Physiol Cell Physiol. (2001) 280:C317–323. doi: 10.1152/ajpcell.2001.280.2.C317
154. Pelantova H, Bartova S, Anyz J, Holubova M, Zelezna B, Maletinska L, et al. Metabolomic profiling of urinary changes in mice with monosodium glutamate-induced obesity. Anal Bioanal Chem. (2016) 408:567–78. doi: 10.1007/s00216-015-9133-0
155. Codoner-Franch P, Tavarez-Alonso S, Murria-Estal R, Herrera-Martin G, Alonso-Iglesias E. Polyamines are increased in obese children and are related to markers of oxidative/nitrosative stress and angiogenesis. J Clin Endocrinol Metab. (2011) 96:2821–5. doi: 10.1210/jc.2011-0531
156. Erwin BG, Bethell DR, Pegg AE. Role of polyamines in differentiation of 3T3-L1 fibroblasts into adipocytes. Am J Physiol. (1984) 246(3 Pt 1):C293–300. doi: 10.1152/ajpcell.1984.246.3.C293
157. Vuohelainen S, Pirinen E, Cerrada-Gimenez M, Keinanen TA, Uimari A, Pietila M, et al. Spermidine is indispensable in differentiation of 3T3-L1 fibroblasts to adipocytes. J Cell Mol Med. (2010) 14:1683–92. doi: 10.1111/j.1582-4934.2009.00808.x
158. Ishii I, Ikeguchi Y, Mano H, Wada M, Pegg AE, Shirahata A. Polyamine metabolism is involved in adipogenesis of 3T3-L1 cells. Amino Acids. (2012) 42:619–26. doi: 10.1007/s00726-011-1037-5
159. Hyvonen MT, Koponen T, Weisell J, Pietila M, Khomutov AR, Vepsalainen J, et al. Spermidine promotes adipogenesis of 3T3-L1 cells by preventing interaction of ANP32 with HuR and PP2A. Biochem J. (2013) 453:467–74. doi: 10.1042/BJ20130263
160. Sadasivan SK, Vasamsetti B, Singh J, Marikunte VV, Oommen AM, Jagannath MR, et al. Exogenous administration of spermine improves glucose utilization and decreases bodyweight in mice. Eur J Pharmacol. (2014) 729:94–9. doi: 10.1016/j.ejphar.2014.01.073
161. Fernandez AF, Barcena C, Martinez-Garcia GG, Tamargo-Gomez I, Suarez MF, Pietrocola F, et al. Autophagy couteracts weight gain, lipotoxicity and pancreatic beta-cell death upon hypercaloric pro-diabetic regimens. Cell Death Dis. (2017) 8:e2970. doi: 10.1038/cddis.2017.373
162. Lockwood DH, East LE. Studies of the insulin-like actions of polyamines on lipid and glucose metabolism in adipose tissue cells. J Biol Chem. (1974) 249:7717–22.
163. Pedersen SB, Hougaard DM, Richelsen B. Polyamines in rat adipocytes: their localization and their effects on the insulin receptor binding. Mol Cell Endocrinol. (1989) 62:161–6. doi: 10.1016/0303-7207(89)90002-6
164. Michiels CF, Kurdi A, Timmermans JP, De Meyer GRY, Martinet W. Spermidine reduces lipid accumulation and necrotic core formation in atherosclerotic plaques via induction of autophagy. Atherosclerosis. (2016) 251:319–27. doi: 10.1016/j.atherosclerosis.2016.07.899
165. Sjoholm A. Role of polyamines in the regulation of proliferation and hormone production by insulin-secreting cells. Am J Physiol. (1993) 264(3 Pt 1):C501–18. doi: 10.1152/ajpcell.1993.264.3.C501
166. Fernandez-Garcia JC, Delpino-Rius A, Samarra I, Castellano-Castillo D, Munoz-Garach A, Bernal-Lopez MR, et al. Type 2 diabetes is associated with a different pattern of serum polyamines: a case(-)control study from the PREDIMED-plus trial. J Clin Med. (2019) 8:71. doi: 10.3390/jcm8010071
167. Deng A, Munger KA, Valdivielso JM, Satriano J, Lortie M, Blantz RC, et al. Increased expression of ornithine decarboxylase in distal tubules of early diabetic rat kidneys: are polyamines paracrine hypertrophic factors? Diabetes. (2003) 52:1235–9. doi: 10.2337/diabetes.52.5.1235
168. Mendez JD, Balderas FL. Inhibition by L-arginine and spermidine of hemoglobin glycation and lipid peroxidation in rats with induced diabetes. Biomed Pharmacother. (2006) 60:26–31. doi: 10.1016/j.biopha.2005.08.004
169. Mendez JD, Hernandez Rde H. L-arginine and polyamine administration protect beta-cells against alloxan diabetogenic effect in Sprague-Dawley rats. Biomed Pharmacother. (2005) 59:283–9. doi: 10.1016/j.biopha.2005.05.006
170. Jafarnejad A, Bathaie SZ, Nakhjavani M, Hassan MZ. Effect of spermine on lipid profile and HDL functionality in the streptozotocin-induced diabetic rat model. Life Sci. (2008) 82:301–7. doi: 10.1016/j.lfs.2007.11.015
171. Fetterman JL, Holbrook M, Flint N, Feng B, Breton-Romero R, Linder EA, et al. Restoration of autophagy in endothelial cells from patients with diabetes mellitus improves nitric oxide signaling. Atherosclerosis. (2016) 247:207–17. doi: 10.1016/j.atherosclerosis.2016.01.043
172. Nissim I, Horyn O, Daikhin Y, Chen P, Li C, Wehrli SL, et al. The molecular and metabolic influence of long term agmatine consumption. J Biol Chem. (2014) 289:9710–29. doi: 10.1074/jbc.M113.544726
173. Wisniewska A, Olszanecki R, Toton-Zuranska J, Kus K, Stachowicz A, Suski M, et al. Anti-atherosclerotic action of agmatine in ApoE-knockout mice. Int J Mol Sci. (2017) 18:E1706. doi: 10.3390/ijms18081706
174. Babbar N, Murray-Stewart T, Casero RA Jr. Inflammation and polyamine catabolism: the good, the bad and the ugly. Biochem Soc Trans. (2007) 35:300–4. doi: 10.1042/BST0350300
175. Moreno-Indias I, Cardona F, Tinahones FJ, Queipo-Ortuno MI. Impact of the gut microbiota on the development of obesity and type 2 diabetes mellitus. Front Microbiol. (2014) 5:190. doi: 10.3389/fmicb.2014.00190
176. Morselli E, Galluzzi L, Kepp O, Criollo A, Maiuri MC, Tavernarakis N, et al. Autophagy mediates pharmacological lifespan extension by spermidine and resveratrol. Aging. (2009) 1:961–70. doi: 10.18632/aging.100110
177. Das R, Kanungo MS. Activity and modulation of ornithine decarboxylase and concentrations of polyamines in various tissues of rats as a function of age. Exp Gerontol. (1982) 17:95–103. doi: 10.1016/0531-5565(82)90042-0
178. Soda K, Dobashi Y, Kano Y, Tsujinaka S, Konishi F. Polyamine-rich food decreases age-associated pathology and mortality in aged mice. Exp Gerontol. (2009) 44:727–32. doi: 10.1016/j.exger.2009.08.013
179. Yue F, Li W, Zou J, Jiang X, Xu G, Huang H, et al. Spermidine prolongs lifespan and prevents liver fibrosis and hepatocellular carcinoma by activating MAP1S-mediated autophagy. Cancer Res. (2017) 77:2938–51. doi: 10.1158/0008-5472.CAN-16-3462
180. Gupta VK, Scheunemann L, Eisenberg T, Mertel S, Bhukel A, Koemans TS, et al. Restoring polyamines protects from age-induced memory impairment in an autophagy-dependent manner. Nat Neurosci. (2013) 16:1453–60. doi: 10.1038/nn.3512
181. Fruhauf PK, Ineu RP, Tomazi L, Duarte T, Mello CF, Rubin MA. Spermine reverses lipopolysaccharide-induced memory deficit in mice. J Neuroinflamm. (2015) 12:3. doi: 10.1186/s12974-014-0220-5
182. Zhang H, Wang J, Li L, Chai N, Chen Y, Wu F, et al. Spermine and spermidine reversed age-related cardiac deterioration in rats. Oncotarget. (2017) 8:64793–808. doi: 10.18632/oncotarget.18334
183. Jing YH, Yan JL, Wang QJ, Chen HC, Ma XZ, Yin J, et al. Spermidine ameliorates the neuronal aging by improving the mitochondrial function in vitro. Exp Gerontol. (2018) 108:77–86. doi: 10.1016/j.exger.2018.04.005
184. Zhu WW, Xiao F, Tang YY, Zou W, Li X, Zhang P, et al. Spermidine prevents high glucose-induced senescence in HT-22 cells by upregulation of CB1 receptor. Clin Exp Pharmacol Physiol. (2018) 45:832–40. doi: 10.1111/1440-1681.12955
185. Morselli E, Marino G, Bennetzen MV, Eisenberg T, Megalou E, Schroeder S, et al. Spermidine and resveratrol induce autophagy by distinct pathways converging on the acetylproteome. J Cell Biol. (2011) 192:615–29. doi: 10.1083/jcb.201008167
186. Pietrocola F, Lachkar S, Enot DP, Niso-Santano M, Bravo-San Pedro JM, Sica V, et al. Spermidine induces autophagy by inhibiting the acetyltransferase EP300. Cell Death Differ. (2015) 22:509–16. doi: 10.1038/cdd.2014.215
187. Sacitharan PK, Lwin S, Gharios GB, Edwards JR. Spermidine restores dysregulated autophagy and polyamine synthesis in aged and osteoarthritic chondrocytes via EP300. Exp Mol Med. (2018) 50:123. doi: 10.1038/s12276-018-0149-3
188. Kiechl S, Pechlaner R, Willeit P, Notdurfter M, Paulweber B, Willeit K, et al. Higher spermidine intake is linked to lower mortality: a prospective population-based study. Am J Clin Nutr. (2018) 108:371–80. doi: 10.1093/ajcn/nqy102
189. Crous-Bou M, Fung TT, Prescott J, Julin B, Du M, Sun Q, et al. Mediterranean diet and telomere length in Nurses' Health Study: population based cohort study. BMJ. (2014) 349:g6674. doi: 10.1136/bmj.g6674
190. Soda K, Phan Nguyen Thanh B, Masanobu K. Mediterranean diet and polyamine intake: possible contribution of increased polyamine intake to inhibition of age-associated disease. Nutr Diet Suppl. (2010) 2011:1–7. doi: 10.2147/NDS.S15349
191. Jung M, Pfeifer GP. Aging and DNA methylation. BMC Biol. (2015) 13:7. doi: 10.1186/s12915-015-0118-4
192. Ciccarone F, Tagliatesta S, Caiafa P, Zampieri M. DNA methylation dynamics in aging: how far are we from understanding the mechanisms? Mech Ageing Dev. (2018) 174:3–17. doi: 10.1016/j.mad.2017.12.002
193. Soda K. Polyamine metabolism and gene methylation in conjunction with one-carbon metabolism. Int J Mol Sci. (2018) 19:3106. doi: 10.3390/ijms19103106
194. Tsuji T, Usui S, Aida T, Tachikawa T, Hu GF, Sasaki A, et al. Induction of epithelial differentiation and DNA demethylation in hamster malignant oral keratinocyte by ornithine decarboxylase antizyme. Oncogene. (2001) 20:24–33. doi: 10.1038/sj.onc.1204051
195. Yamamoto D, Shima K, Matsuo K, Nishioka T, Chen CY, Hu GF, et al. Ornithine decarboxylase antizyme induces hypomethylation of genome DNA and histone H3 lysine 9 dimethylation (H3K9me2) in human oral cancer cell line. PLoS ONE. (2010) 5:e12554. doi: 10.1371/journal.pone.0012554
196. Barres R, Zierath JR. DNA methylation in metabolic disorders. Am J Clin Nutr. (2011) 93:897S−900. doi: 10.3945/ajcn.110.001933
197. Bergman Y, Cedar H. DNA methylation dynamics in health and disease. Nat Struct Mol Biol. (2013) 20:274–81. doi: 10.1038/nsmb.2518
198. Arpon A, Milagro FI, Razquin C, Corella D, Estruch R, Fito M, et al. Impact of consuming extra-virgin olive oil or nuts within a mediterranean diet on DNA methylation in peripheral white blood cells within the PREDIMED-navarra randomized controlled trial: a role for dietary lipids. Nutrients. (2017) 10:E15. doi: 10.3390/nu10010015
199. Chamberlain JA, Dugue PA, Bassett JK, Hodge AM, Brinkman MT, Joo JE, et al. Dietary intake of one-carbon metabolism nutrients and DNA methylation in peripheral blood. Am J Clin Nutr. (2018) 108:611–21. doi: 10.1093/ajcn/nqy119
200. Soda K. The mechanisms by which polyamines accelerate tumor spread. J Exp Clin Cancer Res. (2011) 30:95. doi: 10.1186/1756-9966-30-95
201. Raj KP, Zell JA, Rock CL, McLaren CE, Zoumas-Morse C, Gerner EW, et al. Role of dietary polyamines in a phase III clinical trial of difluoromethylornithine (DFMO) and sulindac for prevention of sporadic colorectal adenomas. Br J Cancer. (2013) 108:512–8. doi: 10.1038/bjc.2013.15
202. Vargas AJ, Ashbeck EL, Wertheim BC, Wallace RB, Neuhouser ML, Thomson CA, et al. Dietary polyamine intake and colorectal cancer risk in postmenopausal women. Am J Clin Nutr. (2015) 102:411–9. doi: 10.3945/ajcn.114.103895
203. Wada M, Funada-Wada U, Mano H, Higashiguchi M, Haba R, Watanabe S, et al. Effects of dietary polyamines on the promotion of mammary tumor in rats. J Health Sci. (2002) 48:376–80. doi: 10.1248/jhs.48.376
Keywords: polyamines, diet, gut microbiota, metabolism, aging, obesity
Citation: Ramos-Molina B, Queipo-Ortuño MI, Lambertos A, Tinahones FJ and Peñafiel R (2019) Dietary and Gut Microbiota Polyamines in Obesity- and Age-Related Diseases. Front. Nutr. 6:24. doi: 10.3389/fnut.2019.00024
Received: 21 December 2018; Accepted: 20 February 2019;
Published: 14 March 2019.
Edited by:
Antonio F. Tiburcio, University of Barcelona, SpainReviewed by:
Chaim Kahana, Weizmann Institute of Science, IsraelJie Yin, Institute of Subtropical Agriculture (CAS), China
Miguel Ángel Medina, Universidad de Málaga, Spain
Copyright © 2019 Ramos-Molina, Queipo-Ortuño, Lambertos, Tinahones and Peñafiel. This is an open-access article distributed under the terms of the Creative Commons Attribution License (CC BY). The use, distribution or reproduction in other forums is permitted, provided the original author(s) and the copyright owner(s) are credited and that the original publication in this journal is cited, in accordance with accepted academic practice. No use, distribution or reproduction is permitted which does not comply with these terms.
*Correspondence: Bruno Ramos-Molina, bruno.ramos@ibima.eu
Rafael Peñafiel, rapegar@um.es
†These authors have contributed equally to this work