- 1University Children’s Hospital and Interdisciplinary Center for Infectious Diseases, University of Tübingen, Tübingen, Germany
- 2Department of Pediatrics, Kinderklinik München Schwabing, StKM GmbH und Klinikum rechts der Isar, Technische Universität München, Munich, Germany
Myeloid-derived suppressor cells (MDSCs) are innate immune cells characterized by their ability to suppress T-cell responses. Recently, we demonstrated that the human-pathogenic fungi Candida albicans and Aspergillus fumigatus induced a distinct subset of neutrophilic MDSCs. To dissect Candida-mediated MDSC induction in more depth, we studied the relative efficacy of different pathogenic non-albicans Candida species to induce and functionally modulate neutrophilic MDSCs, including C. glabrata, C. parapsilosis, C. dubliniensis, and C. krusei. Our data demonstrate that the extent of MDSC generation is largely dependent on the Candida species with MDSCs induced by C. krusei and C. glabrata showing a higher suppressive activity compared to MDSCs induced by C. albicans. In summary, these studies show that fungal MDSC induction is differentially regulated at the species level and differentially affects effector T-cell responses.
Introduction
Candida species cause one of the most prevalent fungal infections worldwide (Pfaller and Diekema, 2007; Brown et al., 2012). Among various Candida species, Candida albicans has been the model organism for the most research studies focused on immunity against Candida infections (Papon et al., 2013). However, the genus Candida consists of multiple species that show a considerable variation in terms of their virulence and phenotype and recent studies showed that particularly diseases caused by NAC species are on the rise (Merseguel et al., 2015).
While C. albicans is well characterized in terms of recognition through PRRs mainly CLRs like Dectin-1, Dectin-2, mannose receptor (MR) and Mincle (Brown, 2010; Plato et al., 2015), recognition of NAC species is less precisely defined. In contrast to C. albicans, phagocytosis of C. parapsilosis by neutrophils was not impaired following Dectin-1 blockade in vitro (Linden et al., 2010) and, dectin-1-/- bone marrow macrophages showed no defect in binding to C. glabrata (Kuhn and Vyas, 2012). Interestingly, studies indicated that Dectin-2 also played a more important role in C. glabrata infection than Dectin-1 (Ifrim et al., 2014). There is also some evidence that T-cell responses are differentially involved in immunity to NAC species. For example, C. albicans and C. parapsilosis were shown to induce different T-cell responses (Tóth et al., 2013), but underlying mechanisms by which different Candida species exert a differential immune response remained elusive.
Myeloid-derived suppressor cells are characterized by their ability to suppress T-cell responses and have mainly been studied in cancer (Bronte, 2009; Gabrilovich and Nagaraj, 2009). However, expansion and involvement of MDSCs has also been reported during various infectious disease conditions, such as polymicrobial sepsis, tuberculosis, and Staphylococcus aureus infections (Delano et al., 2007; Du Plessis et al., 2013; Tebartz et al., 2014). Recently, we showed that C. albicans induces a distinct subset of neutrophilic myeloid-derived suppressor cells (G-MDSCs) which is mediated by a Dectin-1/CARD9 signaling pathway, leading to dampening of T-cell and NK-cell responses (Rieber et al., 2015).
To further broaden our understanding of how MDSCs play a role in modulating the host immune response to Candida infections, we studied the relative efficacy of different pathogenic NAC species to induce neutrophilic MDSCs, including C. glabrata, C. krusei, C. parapsilosis, and C. dubliniensis.
Our data demonstrate that the generation of MDSCs is largely dependent on the Candida species and morphotype. Further results also show, that Dectin-1 but not Dectin-2 has an important role during NAC induced MDSC generation.
Materials and Methods
Study Subjects
The study was conducted at the University Children’s Hospital Tübingen (Germany). MDSCs were analyzed in primary cell cultures from peripheral blood obtained from healthy subjects. Informed consent was obtained from all subjects included in the study and the local ethics committee approved all study methods. At the time of blood sampling, all healthy subjects were without any signs of infection, inflammation, or respiratory symptoms.
Candida Species and Culture Conditions
Candida albicans, C. krusei, C. glabrata, C. dubliniensis, and C. parapsilosis strains were stored as frozen stocks in 35% glycerol at -80°C and routinely grown on Sabouraud (Sab) agar (1% mycological peptone, 4% glucose, and 1.5% agar) and YPD agar (1% yeast extract, 2% bacteriological peptone, 2% glucose, and 1.5% agar) plates at 25°C. One colony was inoculated and shaken at 150 rpm at 30°C in YPD broth (1% yeast extract, 2% bacteriological peptone, and 2% glucose) overnight. Cells were harvested by centrifugation and washed twice in sterile Dulbecco’s phosphate-buffered saline (PBS). Cells were counted in a haemocytometer and density was adjusted to the desired concentration in either PBS or RPMI 1640 medium. To generate hyphae, live yeast forms of C. albicans were grown for 6 h at 37°C in RPMI 1640 medium (Gibco-BRL). Heat-inactivated Candida cells were prepared by heat treatment of the cell suspension at 90°C for 30 min.
In vitro MDSC Generation and Flow Cytometry
Human MDSCs were generated in vitro as described previously (Lechner et al., 2010; Rieber et al., 2015). In brief, isolated human PBMCs were cultured in 24 well flat-bottom plates (Corning) or 25 cm2 flasks (Greiner Bio-One) at 5 × 105 cells/ml in RPMI 1640 supplemented with 10% heat-inactivated FCS (PAA Laboratories), 2 mM glutamine (Sigma-Aldrich), 100 IU/ml penicillin, and 100 mg/ml streptomycin (Biochrom; referred to as “complete medium”) for 6 days, and GM-CSF (10 ng/ml, Genzyme), heat-inactivated C. albicans, C. glabrata, C. krusei, C. dubliniensis, and C. parapsilosis were added at a ratio of 1:5 (Fungi:PBMC) as indicated in figures. Dectin-1 antagonist Laminarin obtained from Laminaria digitata (100 μg/ml, Sigma) and Dectin-2 antagonist whole mannan particle preparation isolated from Saccharomyces cerevisiae (100 μg/ml, Sigma) were added in cell culture where indicated. For ROS inhibition assays, PBMCs were incubated with NADPH oxidase inhibitor DPI (DPI, 0.1 μM; Sigma-Aldrich) for 1 h prior to adding the stimulants.
The number of MDSCs in % of all cells in medium only cultures was set to 1-fold for every single experiment. The MDSC induction due to the specific stimuli is presented as x-fold compared to medium control. Medium and supplements were refreshed on day 4 and supernatants were frozen for ELISA. After 6 days, all cells were collected from PBMC cultures using non-protease cell detachment solution Detachin (Genlantis). G-MDSCs were characterized as CD33+CD11b+ CD14- cells as described before (Rieber et al., 2013, 2015).
Cell Isolation and T-Cell Suppression Assays
For functional assays, CD33+ MDSCs were isolated from in vitro cultures using anti-CD33 magnetic microbeads and autoMACS®Pro Separator (Miltenyi Biotec) according to manufacturer’s instructions. Morphology of the MDSCs was analyzed by cytospin staining. For cytospin stainings 5 x 104 CD33+ cells were centrifuged in a Cytospin three centrifuge (Shandon) at 800 rpm for 15 min followed by staining with May-Grunwald-Giemsa method (Supplementary Figure S1). T-cell suppression assays were performed as described previously (Rieber et al., 2015). PBMCs were obtained from healthy volunteers and stained with CFSE according to the manufacturer’s protocol (Invitrogen). PBMCs were stimulated with 100 U/ml IL-2 (R&D Systems) and 1 μg/ml OKT3 (Janssen Cilag). Cell number was adjusted to 5 × 105 cells per ml and a total of 60,000 PBMCs per well were seeded in RPMI1640 (Biochrom) medium, in a 96-well microtitre plate and different numbers of MDSCs in RPMI1640 were added to get an MDSC:T-cell ratio 1:2, 1;4, 1:8, 1:16, and 1:32. The cell culture was supplemented with 10% heat-inactivated human serum, 2 mM glutamine, 100 IU/ml penicillin, and 100 mg/ml streptomycin. After 96 h of incubation in a humidified atmosphere at 37°C and 5% CO2, cells were harvested and supernatants were frozen in -20°C. CFSE-fluorescence intensity was analyzed by flow cytometry to determine T-cell proliferation.
Flow Cytometry
Antibodies against human CD4, CD8, and CD14 were purchased from BD Pharmingen. Antibodies against CD11b and CD33 were purchased from Miltenyi Biotec. Flow cytometry was performed using a FACSCalibur (BD). Results were expressed as percent of positive cells and mean fluorescence intensity (MFI). Calculations were performed with BD CellQuest Pro analysis software and FlowJo.
Cytokine Analysis in Culture Supernatants
IL-1β (R&D systems) and GM-CSF (Biolegend) ELISA Kits were used to quantify cytokine protein levels in cell culture supernatants. Released IFN-γ protein was quantified by using the Human IFN-γ DuoSet (R&D Systems). All assays were performed according to the manufacturer’s recommendations.
Statistical Analysis
Statistical analysis was performed in GraphPad Prism version 6.0 using a one-sample t-test. In all tests, differences were considered significant at P < 0.05 (∗P < 0.05; ∗∗P < 0.01; ∗∗∗P < 0.001; ∗∗∗∗P < 0.0001).
Results
Different Candida Species Induce Functional G-MDSCs
First, we assessed the ability of NAC species to induce human G-MDSCs and to control their function. G-MDSCs were defined by their surface markers (CD11b+CD33+CD14-) and by their characteristic to suppress T-cell responses. By comparing Candida species, we found a differential pattern of MDSC induction among all Candida species. While C. albicans (9.1-fold) was the strongest inducer of G-MDSCs, C. krusei, and C. glabrata (5.5- and 6.1-fold, respectively) also induced high amounts of MDSCs, followed by C. parapsilosis (3.5-fold) and C. dubliniensis (2.1-fold), which was least potent in comparison to others (Figure 1A). G-MDSC induction by C. albicans was observed for different fungal morphotypes and even occurred using filter sterilized C. albicans yeast supernatants (Figure 1B). M-MDSCs (CD11b+CD33+CD14+) were not induced during these culture conditions. (Supplementary Figure S2).
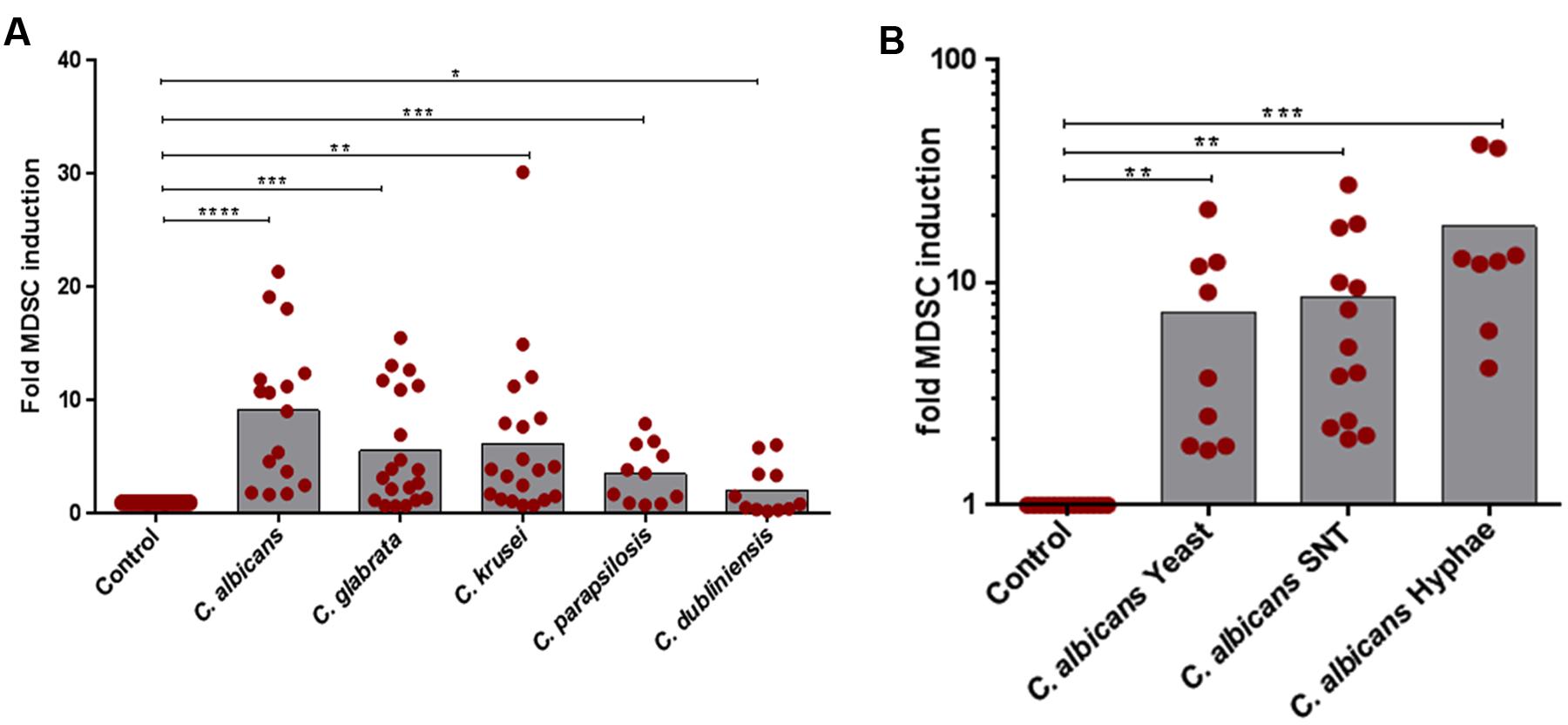
FIGURE 1. In vitro MDSC generation by different Candida non-albicans species and C. albicans morphotypes. MDSCs were generated by incubating freshly isolated PBMCs (5 × 105/ml) from healthy donors with medium only (negative control) or indicated stimulants. (A) PBMCs were cultured with heat killed yeast cells of C. albicans, C. glabrata, C. krusei, C. parapsilosis, and C. dubliniensis (1 × 105/ml) for 6 days (n = 11–20) or (B) with heat killed C. albicans yeast cells (1 × 105/ml), filter sterilized C. albicans yeast supernatant (5% SNT), or C. albicans hyphae (1 × 105/ml) for 6 days (n = 8–13). Granulocytic MDSCs (CD11b+CD33+CD14-) were quantified by using Flow Cytometry. The number of MDSCs in % of all cells in medium only cultures was set to 1-fold for every single experiment. The MDSC induction due to specific stimuli is presented as x-fold compared to medium control (mean ± SEM) and differences compared to controls were analyzed by a one-sample t-test. Significant differences between control and G-MDSCs induction by stimulants are indicated by an asterisk (∗P < 0.05; ∗∗P < 0.01; ∗∗∗P < 0.001; ∗∗∗∗P < 0.0001).
MDSCs Induced by Non-albicans Candida Species Are More Suppressive than MDSCs Induced by C. albicans
The key function attributed to MDSCs is to suppress T-cell responses. (Bronte et al., 2016). Therefore, we performed functional assays to screen for T-cell suppression capability of Candida-induced MDSCs. CFSE assays showed that NAS-induced myeloid cells strongly suppressed both CD4+ and CD8+ T cell proliferation in a dose-dependent manner. Interestingly, MDSCs induced by C. krusei and C. glabrata exhibited an even higher suppressive activity than MDSCs induced by C. albicans, an effect which was significant at MDSC:T cell ratios of 1:8 and 1:16. (Figures 2A,B). Apart from T-cell proliferation assays, we also investigated the impact of fungi-derived MDSCs on IL-2 and OKT3-induced T cell cytokine production. These studies demonstrated that MDSCs efficiently suppressed IFN-γ secretion (Figure 2C).
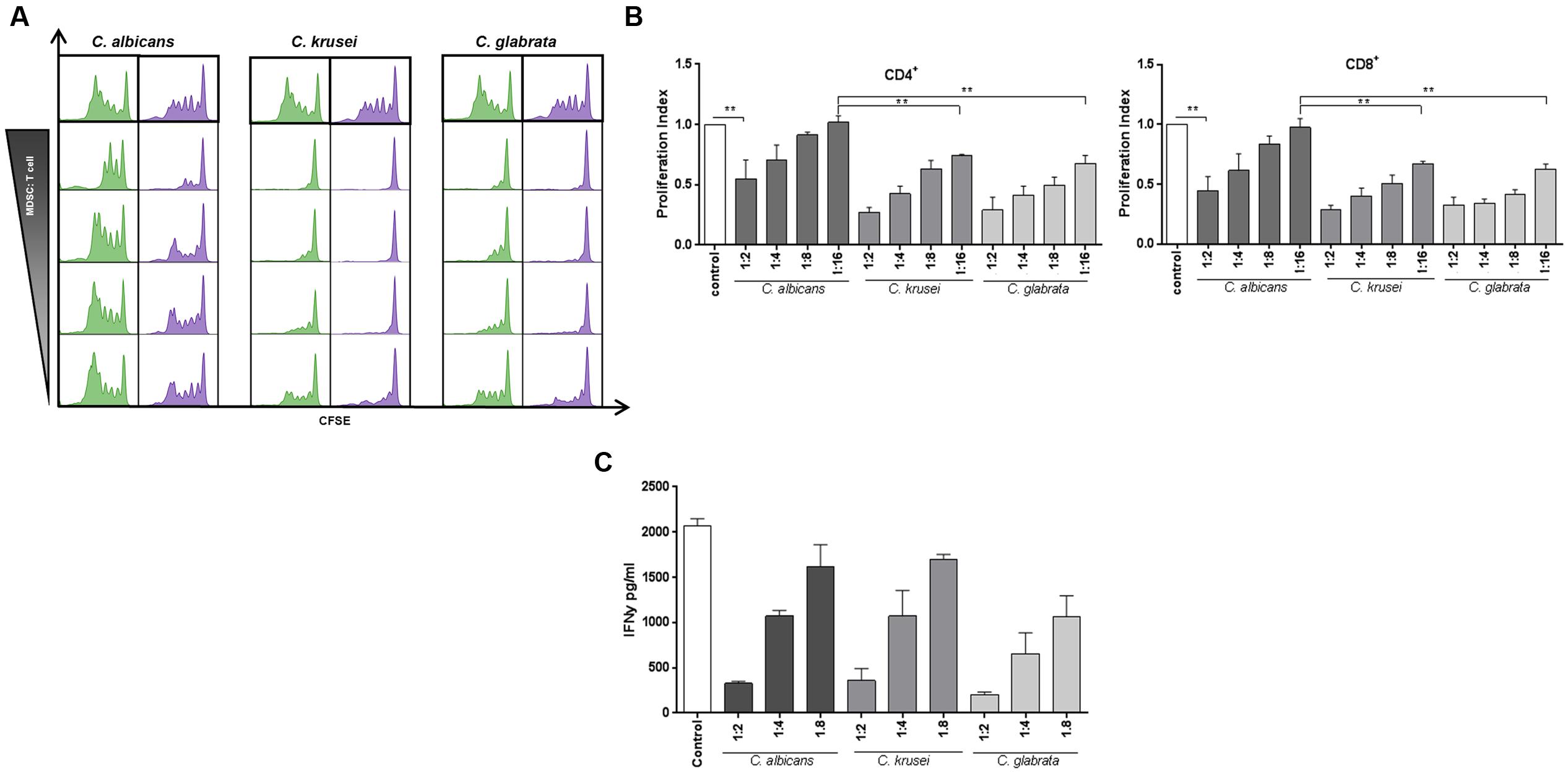
FIGURE 2. Candida-induced MDSCs suppress T cell responses. MDSCs generated by Candida species are able to suppress T-cell proliferation and function in a dose dependent manner. The suppressive effects of CD33+-MACS-isolated MDSCs on CD4+ (green) and CD8+ (lilac) were assessed by T-cell proliferation (CFSE polyclonal proliferation) assay. MDSCs were generated by incubating PBMCs (5 × 105/ml) from healthy donors with heat killed yeast cells of various Candida species (1 × 105/ml) or C. albicans yeasts for 6 days. (A) Representative CFSE stainings, showing the effect of in vitro C. albicans, C. krusei, and C. glabrata induced MDSCs on CD4+ and CD8+ T-cell proliferation. Different MDSC to T cell ratios were assessed by using a wide range of MDSC:Target ratio (1:2, 1:4, 1:6, 1:8, and 1:16). (B) The bar graphs represent the proliferation index compared to control conditions. Even at a higher MDSC:target ratio of 1:16, MDSCs induced by C. krusei, and C. glabrata show higher suppressive activity in comparison to C. albicans. Data is shown as mean ± SEM (n = 4) ∗∗P < 0.01. (C) IFNγ secretion of T cells is decreased by MDSCs. IFNγ secretion in the supernatant was measured on day 4 of MDSC/T cell co-culture experiments by ELISA. The concentration is given in pg/ml (n = 3).
Dectin-1, but not Dectin-2, Is Involved in MDSC Induction by Non-albicans Candida Species
In our previous work we showed that Dectin-1 plays a key role in C. albicans-induced MDSC generation. Several studies also reveal the role of Dectin-1 and also Dectin-2 (Saijo and Iwakura, 2011) in immune mechanisms against NAC species. We therefore focussed on Dectin-1 and Dectin-2 as β-glucan and mannan receptors, essentially involved in recognition of fungi. As shown for C. albicans, blocking of Dectin-1 prior to co-culture with fungal cells diminished the MDSC-inducing effect significantly in C. glabrata. For C. krusei-induced MDSCs we observed a similar, however, not significant effect. On the other hand, blocking of Dectin-2 had no effect (Figure 3A) suggesting that Dectin-2 is dispensable for Candida-mediated MDSC generation. Since fundamental differences have been reported between host recognition of C. albicans morphotypes (Lowman et al., 2014), we next examined the impact of Dectin-1 blockage on MDSC generation. In case of C. albicans yeast cells and hyphae, Dectin-1 blockage significantly inhibited the MDSCs. Dectin-1 blockage also led to a similar trend for filter sterilized C. albicans yeast cell supernatant, however, it was not significant (Figure 3B).
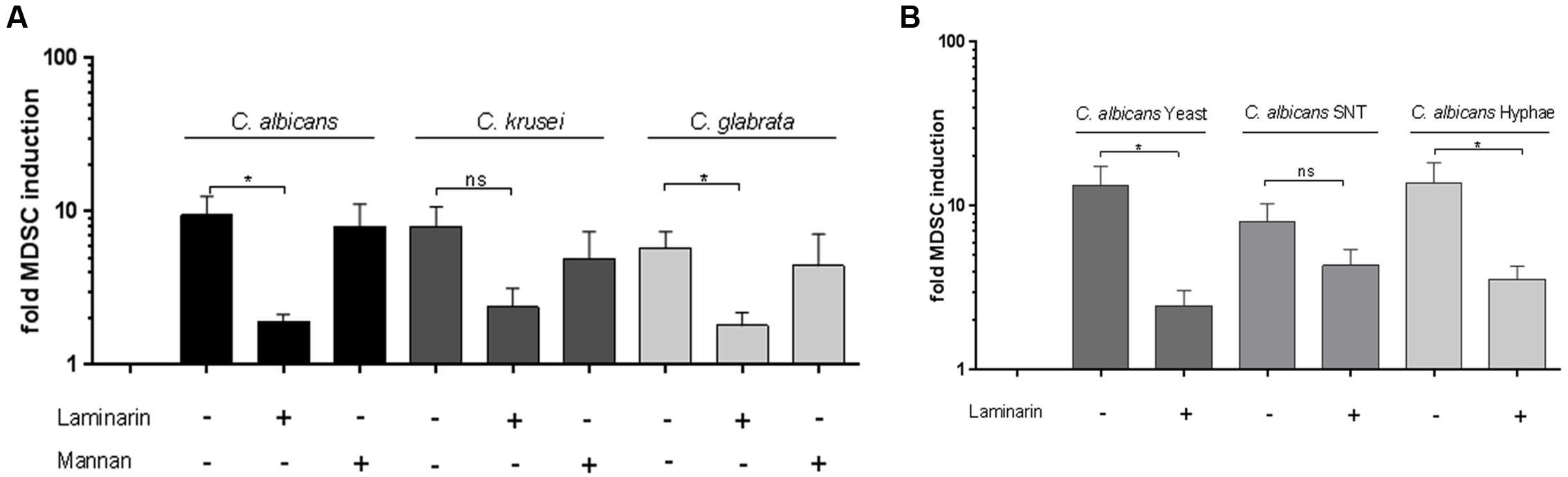
FIGURE 3. Dectin-1 is involved in Candida-mediated MDSC induction in vitro. MDSCs were generated in vitro by incubating isolated PBMCs (5 × 105 cells/ml) with stimulants and inhibitors. (A) with heat killed yeast cells of C. albicans, C. krusei, and C. glabrata (all 1 × 105/ml), (n = 8–11) or (B) with heat killed C. albicans yeast cells (1 × 105/ml), filter sterilized C. albicans yeast cell supernatant (5% SNT) or C. albicans hyphae for 6 days (n = 8–13). Where indicated, prior to stimulation, PBMCs were pretreated for 60 min with Dectin-1 inhibitor Laminarin (100 μg/ml) or Mannan (100 μg/ml) from Saccharomyces cerevisea to mimic Dectin-2 binding without receptor activating capacity. (∗P < 0.05, Bars represent SEM).
Candida-Mediated MDSC Generation Is Associated with GM-CSF, IL-1β, and ROS Production
The cytokine GM-CSF has been involved in MDSC generation (Gabrilovich and Nagaraj, 2009; Dolcetti et al., 2010) and previous studies showed that GM-CSF is secreted upon stimulation with fungal pathogens. (Li and Dongari-Bagtzoglou, 2009; Svobodová et al., 2012). Therefore we hypothesized that GM-CSF might play a role in Candida-mediated MDSC generation and analyzed the amount of GM-CSF in conditioned medium obtained from PBMC-Candida co-culture. Our results demonstrate that C. albicans stimulation leads to a high amount of GM-CSF release in comparison to C. glabrata and C. krusei (Figure 4A). In addition to GM-CSF, the inflammasome product IL-1β has been previously involved in MDSC induction (Elkabets et al., 2010; Lechner et al., 2011; Ballbach et al., 2016). Hence, we quantified IL-1β protein in our assays and found that C. albicans, C. glabrata, and C. krusei, all three major pathogenic Candida species lead to high amounts of IL-1β secretion upon PBMC stimulation (Figure 4B). These results indicate that the two MDSC-related cytokines GM-CSF and IL-1β seem to be associated with fungal MDSC induction. ROS have been consistently involved in MDSC generation and function (Gabrilovich and Nagaraj, 2009). To check the role of ROS, MDSCs were generated in vitro by incubating isolated PBMCs (5 × 105 cells/ml) with different Candida stimulants (1 × 105 cells/ml) and pretreatment for 1 h with the NADPH oxidase inhibitor DPI (0.1 μM) where indicated. These experiments showed that ROS contributed substantially to fungi-mediated MDSC induction in vitro (Figure 4C).
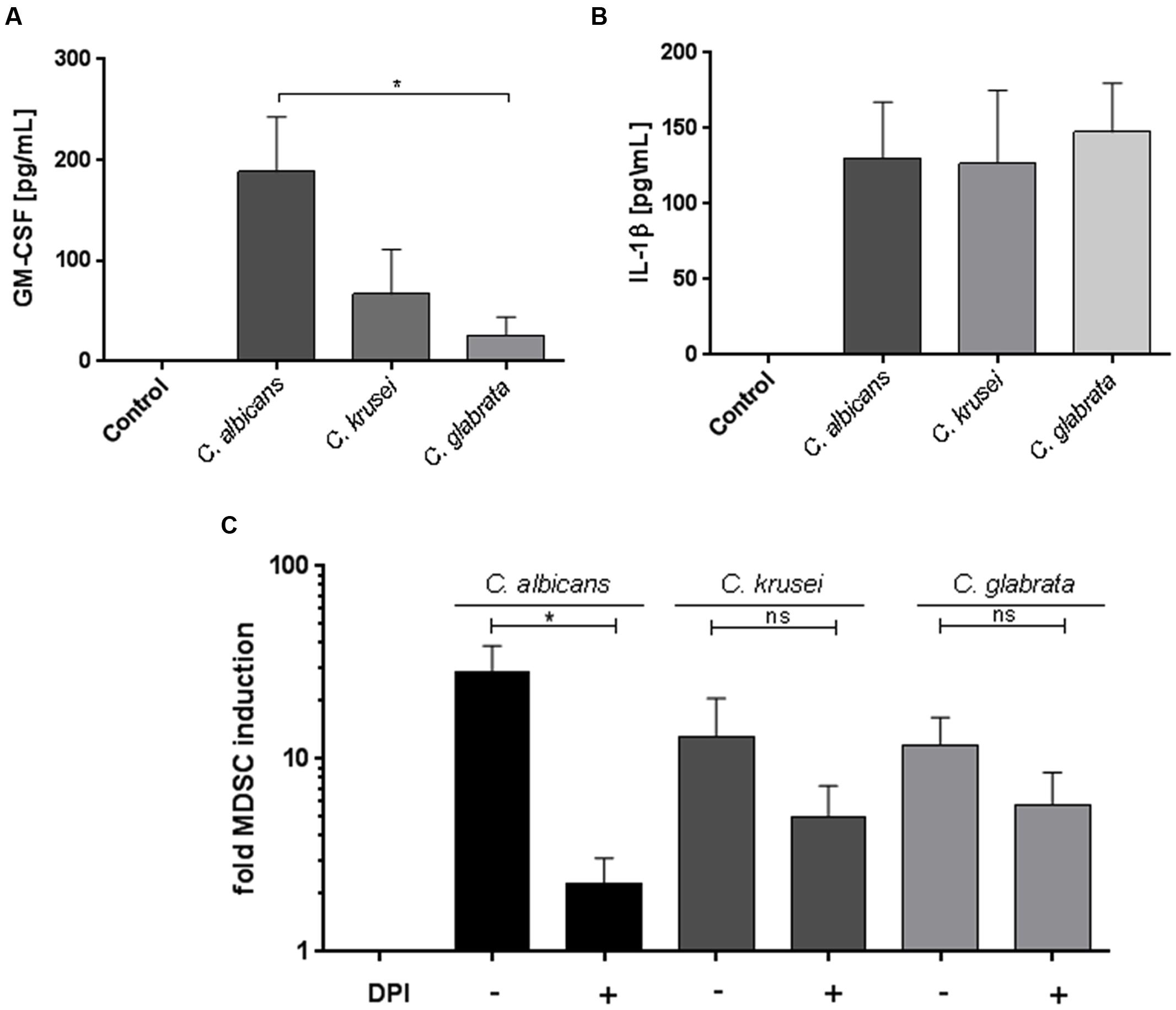
FIGURE 4. Candida-mediated MDSC generation involves GM-CSF, IL-1β, and ROS. GM-CSF, IL-1β, and ROS are involved in Candida-mediated MDSC generation. Freshly isolated PBMCs (5 × 105 cells/ml) were cultured in medium only, or with heat killed yeast cells of C. albicans (1 × 105/ml), C. krusei (1 × 105 cells/ml), and C. glabrata (1 × 105 cells/ml) for 4 days. For quantification of cytokines, co-culture supernatants were collected on day 4. (A) GM-CSF (n = 8) and (B) IL-1β (n = 6) levels were quantified by ELISA. (C) MDSCs were generated in vitro by incubating isolated PBMCs (5 × 105 cells/ml) with with heat killed yeast cells of C. albicans (1 × 105/ml), C. krusei (1 × 105 cells/ml), and C. glabrata (1 × 105 cells/ml) for 6 days. Prior to stimulation, PBMCs were pretreated for 60 min where indicated with the NADPH oxidase inhibitor DPI (0.1 μM; n = 6) (∗P < 0.05, Bars represent SEM).
Discussion
Previous studies from our group demonstrated that pathogenic fungi A. fumigatus and C. albicans induce MDSCs, which suppress T cell responses (Rieber et al., 2015). In this study, we compared the capacity of C. albicans, C. glabrata, C. krusei, and C. parapsilosis to induce G-MDSCs and the relative strength of Candida-induced G-MDSCs to suppress T-cell proliferation and cytokine production.
Candida species are found as commensal organisms at mucosal surfaces in the human body. Since C. albicans is the most prominent fungus isolated from clinical samples, research related to anti-fungal immune response is largely centered on it. However, recent clinical studies have reported a rise in the NAC species isolated from clinical samples of fungal infections. NAC species associated with disease mainly include C. glabrata, C. krusei, C. dubliniensis, and C. parapsilosis (Butler et al., 2009). Here we extend our previous findings by showing that the strength of Candida-mediated MDSC induction substantially depends on the Candida species. While C. albicans was the strongest inducer of MDSCs, C. dubliniensis showed the lowest capacity. Importantly, our studies further show that not only the extent, but also the functionality of MDSCs is regulated by distinct Candida species. Collectively, these studies add to our understanding of how different Candida species differentially modulate host immunity.
Candida species consist of a diverse range of virulence factors and morphotypes. Although limited in number, studies using in vitro methods and in vivo infection strategies demonstrate that host innate immune responses to Candida challenge including activation and function of neutrophils (Dementhon et al., 2012; Svobodová et al., 2012; Duggan et al., 2015), dendritic cells (Bourgeois et al., 2011), and macrophages (Seider et al., 2011) differ depending on the Candida species. In addition to different species, we also used C. albicans yeast and hyphal forms and filter sterilized supernatant from yeast cultures to study the impact of different fungal morphotypes and soluble products during fungi-mediated MDSC generation. C. albicans yeast to hyphae morphogenesis has been attributed as a crucial virulence factor during fungal pathogenesis. Various studies demonstrate that immune cell recognition and subsequent immune response toward different morphotypes of C. albicans differs (Lewis et al., 2012; Lowman et al., 2014) due to differential exposure of cell wall components, e.g., β-glucans (Wheeler et al., 2008; Gow et al., 2011). However, in our studies, we did not find a difference in MDSC induction after stimulation with C. albicans yeast and hyphae (Rieber et al., 2015) or supernatants. Further studies involving various morphotypes of different NAC species and secreted fungal virulence factors will help to dissect the mechanism underlying Candida-mediated MDSC generation and function. T cells are pivotal immune cells during C. albicans infection and patients with decreased CD4+ T cells were found to be highly susceptible to mucocutaneous and invasive Candidiasis (Fidel, 2011; Lionakis and Netea, 2013). Interestingly, C. glabrata and C. krusei-generated MDSCs were more suppressive on T cell proliferation than C. albicans-generated MDSCs and this phenomenon was recapitulated in the suppression of IFNγ release. There is some evidence suggesting differential T-cell responses depending on the Candida species. C. albicans and C. parapsilosis were found to induce different T-cell responses and cytokines. Human PBMCs stimulated with heat killed C. parapsilosis yeast cells showed higher production of IL-10 but lower amounts of IL-1β, IFNγ, IL-17, and IL-22, when compared to cells stimulated with C. albicans (Tóth et al., 2013). Another study reported distinct T-cell generation in response to C. albicans and NAC species and T cells generated after stimulation with C. albicans displayed cross-reactivity only with C. tropicalis but not C. glabrata (Tramsen et al., 2007). Our findings now also hint toward a species-dependent innate immune response against different Candida species. The induction of MDSCs might contribute to a fine-tuned balance between pro-inflammatory effector and counter-regulatory immune mechanisms, which has been demonstrated to be crucial for an effective anti-fungal immune response (Zelante et al., 2011, 2012; Rieber et al., 2015).
Candida albicans is recognized by different classes of PRRs among which, the CLRs including Dectin-1and Dectin-2 are the most important ones described so far. In our previous work, we showed that dectin-1 mediated signaling was prominent in fungi-induced MDSC generation. While Dectin-1 has been shown to be the key PRR for C. albicans (Taylor et al., 2007; Marakalala et al., 2013), Dectin-2 has emerged as a leading PRR to recognize both C. albicans and C. glabrata (Saijo et al., 2010; Ifrim et al., 2014). Therefore we focussed on these two PRRs to clarify their role in Candida-mediated MDSC generation. In consistence with our previous findings for C. albicans (Rieber et al., 2015), we found that blockage of Dectin-1 but not Dectin-2 led to diminished MDSC generation by C. albicans, C. glabrata, and C. krusei. Our results demonstrate that Candida-mediated MDSC induction is dependent on the type of Candida species, which is in line with the notion that anti-fungal immune responses are species- and strain-specific and vary in terms of recognition by the host immune system (Netea et al., 2010; Marakalala et al., 2013). Future studies will be essential to expand the understanding how differential adaptation of Candida strains plays a role in MDSC generation. Different morphotypes of C. albicans induce an altered immune response. It has been reported that C. albicans yeast cells and hyphae are differentially recognized by Dectin-1 and Dectin-2 during host-pathogen-interaction (Saijo et al., 2010; Saijo and Iwakura, 2011). We observed a similar MDSC induction independent of the C. albicans morphotype. Dectin-1 blockage significantly inhibited the MDSC generation by C. albicans yeast cells and hyphae, and led to a similar trend for C. albicans supernatant. This hints toward the presence of a soluble Dectin-1 ligand in C. albicans supernatant that contributes to MDSC generation. Interestingly, while yeast mannan particles have been described to impact not only Dectin-2, but also other PRRs like MR, DC-SIGN, and Mincle (Netea et al., 2015), we did not observe any effect of mannan treatment on Candida-mediated MDSC generation in our studies.
To elucidate the mechanism of Candida-mediated MDSC induction, we further focused on two key cytokines, GM-CSF and IL-1β, both reported to play an important role in MDSC generation and homeostasis (Elkabets et al., 2010; Lechner et al., 2011; Gabrilovich et al., 2012; Bayne et al., 2016), as well as during fungal pathogenesis (Svobodová et al., 2012; Netea et al., 2015). Stimulation of PBMCs with C. albicans and NAC species led to release of GM-CSF and IL-1β. C. albicans-mediated release of GM-CSF was significantly higher than that of C. glabrata, possibly explaining the stronger induction of MDSCs upon C. albicans stimulation. All three species C. albicans, C. glabrata, and C. krusei released similar amounts of IL-1β upon PBMC stimulation. Since Dectin-1 was found to be the key receptor for Candida-mediated MDSC generation, and previous studies demonstrated that ROS act downstream of Dectin-1 (Branzk et al., 2014), and ROS have been shown to be involved in MDSC homeostasis (Corzo et al., 2010; Gabrilovich et al., 2012), we further examined the role of ROS for Candida-mediated MDSC induction. These studies demonstrated that ROS contributed substantially to NAC-mediated MDSC induction in vitro.
Conclusion
Our results demonstrate that Candida-mediated MDSC induction is differentially regulated at the species level and differentially affects effector T-cell responses. In our previous study using a systemic infection mouse model for C. albicans, we showed that adaptive transfer with MDSCs leads to a protective effect against invasive Candidiasis. While the classical MDSC inducing factor GM-CSF has already been proposed as one of the leading candidates for anti-fungal adjunctive therapy (Vazquez et al., 1998; van de Veerdonk et al., 2012), in vivo generation of MDSCs or ex vivo expansion and adoptive transfer might become an interesting approach for future therapeutic strategies against infections caused by Candida species.
Author Contributions
AS designed the study, performed the experiments, analyzed the data, and wrote the manuscript. FL, SB, and IS performed the experiments. DH and NR co-designed the study, supervised experiments, discussed data, and co-wrote the manuscript.
Funding
This work was supported by the German Research Foundation (DFG, SFB/CRC685) at Tübingen to DH and the fortüne program (no. 2268-0-0) of faculty of medicine, Tübingen, to NR.
Conflict of Interest Statement
The authors declare that the research was conducted in the absence of any commercial or financial relationships that could be construed as a potential conflict of interest.
Acknowledgment
Authors thank all the blood donors who participated in the study.
Supplementary Material
The Supplementary Material for this article can be found online at: http://journal.frontiersin.org/article/10.3389/fmicb.2016.01624
Abbreviations
CFSE, Carboxyfluoresceinsuccinimidyl ester; DPI, Diphenyleneiodonium chloride; ELISA, Enzyme-linked immunosorbent assay; FACS, Fluorescence-activated cell sorting; GM-CSF, Granulocyte-macrophage colony-stimulating factor; G-MDSCs, Granulocytic myeloid-derived suppressor cells; IL-, Interleukin; MACS, Magnetic-activated cell sorting; MDSCs, Myeloid-derived suppressor cells; M-MDSCs, Monocytic myeloid-derived suppressor cells; NAC, Non-albicans Candida; PBMCs, Peripheral blood mononuclear cells; PRR, Pattern recognition receptor; ROS, Reactive oxygen species.
References
Ballbach, M., Hall, T., Brand, A., Neri, D., Singh, A., Schaefer, I., et al. (2016). Induction of myeloid-derived suppressor cells in cryopyrin-associated periodic syndromes. J. Innate Immun. 8, 493–506. doi: 10.1159/000446615
Bayne, L. J., Beatty, G. L., Jhala, N., Clark, C. E., Rhim, A. D., Stanger, B. Z., et al. (2016). Tumor-derived granulocyte-macrophage colony-stimulating factor regulates myeloid inflammation and T cell immunity in pancreatic cancer. Cancer Cell 21, 822–835. doi: 10.1016/j.ccr.2012.04.025
Bourgeois, C., Majer, O., Frohner, I. E., Lesiak-Markowicz, I., Hildering, K.-S., Glaser, W., et al. (2011). Conventional Dendritic Cells Mount a Type I IFN Response against Candida spp. Requiring Novel Phagosomal TLR7-Mediated IFN-β Signaling. J. Immunol. 186, 3104–3112. doi: 10.4049/jimmunol.1002599
Branzk, N., Lubojemska, A., Hardison, S. E., Wang, Q., Gutierrez, M. G., Brown, G. D., et al. (2014). Neutrophils sense microbe size and selectively release neutrophil extracellular traps in response to large pathogens. Nat. Immunol. 15, 1017–1025. doi: 10.1038/ni.2987
Bronte, V. (2009). Myeloid-derived suppressor cells in inflammation: uncovering cell subsets with enhanced immunosuppressive functions. Eur. J. Immunol. 39, 2670–2672. doi: 10.1002/eji.200939892
Bronte, V., Brandau, S., Chen, S.-H., Colombo, M. P., Frey, A. B., Greten, T. F., et al. (2016). Recommendations for myeloid-derived suppressor cell nomenclature and characterization standards. Nat. Commun. 7:12150. doi: 10.1038/ncomms12150
Brown, G. D. (2010). How fungi have shaped our understanding of mammalian immunology. Cell Host Microbe 7, 9–11. doi: 10.1016/j.chom.2009.12.005
Brown, G. D., Denning, D. W., and Levitz, S. M. (2012). Tackling Human Fungal Infections. Science 336, 647. doi: 10.1126/science.1222236
Butler, G., Rasmussen, M. D., Lin, M. F., Santos, M. A. S., Sakthikumar, S., Munro, C. A., et al. (2009). Evolution of pathogenicity and sexual reproduction in eight Candida genomes. Nature 459, 657–662. doi: 10.1038/nature08064
Corzo, C. A., Condamine, T., Lu, L., Cotter, M. J., Youn, J.-I., Cheng, P., et al. (2010). HIF-1α regulates function and differentiation of myeloid-derived suppressor cells in the tumor microenvironment. J. Exp. Med. 207, 2439–2453. doi: 10.1084/jem.20100587
Delano, M. J., Scumpia, P. O., Weinstein, J. S., Coco, D., Nagaraj, S., Kelly-Scumpia, K. M., et al. (2007). MyD88-dependent expansion of an immature GR-1(+)CD11b(+) population induces T cell suppression and Th2 polarization in sepsis. J. Exp. Med. 204, 1463–1474. doi: 10.1084/jem.20062602
Dementhon, K., El-Kirat-Chatel, S., and Noël, T. (2012). Development of an in vitro model for the multi-parametric quantification of the cellular interactions between Candida Yeasts and Phagocytes. PLoS ONE 7:e32621. doi: 10.1371/journal.pone.0032621
Dolcetti, L., Peranzoni, E., Ugel, S., Marigo, I., Gomez, A. F., Mesa, C., et al. (2010). Hierarchy of immunosuppressive strength among myeloid-derived suppressor cell subsets is determined by GM-CSF. Eur. J. Immunol. 40, 22–35. doi: 10.1002/eji.200939903
Du Plessis, N., Loebenberg, L., Kriel, M., Von Groote-Bidlingmaier, F., Ribechini, E., Loxton, A. G., et al. (2013). Increased frequency of Myeloid-derived suppressor cells during active tuberculosis and after recent Mycobacterium tuberculosis infection suppresses T-cell function. Am. J. Respir. Crit. Care Med. 188, 724–732. doi: 10.1164/rccm.201302-0249OC
Duggan, S., Essig, F., Hünniger, K., Mokhtari, Z., Bauer, L., Lehnert, T., et al. (2015). Neutrophil activation by Candida glabrata but not Candida albicans promotes fungal uptake by monocytes. Cell. Microbiol. 17, 1259–1276. doi: 10.1111/cmi.12443
Elkabets, M., Ribeiro, V., Dinarello, C., Ostrand-Rosenberg, S., Di Santo, J., Apte, R., et al. (2010). IL-1β regulates a novel myeloid-derived suppressor cell subset that impairs NK cell development and function. Eur. J. Immunol. 40, 3347–3357. doi: 10.1002/eji.201041037.IL-1
Fidel, P. L. (2011). Candida-host interactions in HIV disease: implications for oropharyngeal candidiasis. Adv. Dent. Res. 23, 45–49. doi: 10.1177/0022034511399284
Gabrilovich, D. I., and Nagaraj, S. (2009). Myeloid-derived suppressor cells as regulators of the immune system. Nat. Rev. Immunol. 9, 162–174. doi: 10.1038/nri2506
Gabrilovich, D. I., Ostrand-Rosenberg, S., and Bronte, V. (2012). Coordinated regulation of myeloid cells by tumours. Nat. Rev. Immunol. 12, 253–268. doi: 10.1038/nri3175
Gow, N. A. R., van de Veerdonk, F. L., Brown, A. J. P., and Netea, M. G. (2011). Candida albicans morphogenesis and host defence: discriminating invasion from colonization. Nat. Rev. Microbiol. 10, 112–122. doi: 10.1038/nrmicro2711
Ifrim, D. C., Bain, J. M., Reid, D. M., Oosting, M., Verschueren, I., Gow, N. A. R., et al. (2014). Role of Dectin-2 for host defense against systemic infection with Candida glabrata. Infect. Immun. 82, 1064–1073. doi: 10.1128/IAI.01189-13
Kuhn, D. M., and Vyas, V. K. (2012). The Candida glabrata adhesin Epa1p causes adhesion, phagocytosis, and cytokine secretion by innate immune cells. FEMS Yeast Res. 12, 398–414. doi: 10.1111/j.1567-1364.2011.00785.x
Lechner, M. G., Liebertz, D. J., and Epstein, A. L. (2010). Characterization of cytokine-induced myeloid-derived suppressor cells from normal human peripheral blood mononuclear cells. J. Immunol. 185, 2273–2284. doi: 10.4049/jimmunol.1000901
Lechner, M. G., Megiel, C., Russell, S. M., Bingham, B., Arger, N., Woo, T., et al. (2011). Functional characterization of human Cd33+ and Cd11b+ myeloid-derived suppressor cell subsets induced from peripheral blood mononuclear cells co-cultured with a diverse set of human tumor cell lines. J. Transl. Med. 9:90. doi: 10.1186/1479-5876-9-90
Lewis, L. E., Bain, J. M., Lowes, C., Gillespie, C., Rudkin, F. M., Gow, N. A. R., et al. (2012). Stage specific assessment of Candida albicans phagocytosis by macrophages identifies cell wall composition and morphogenesis as key determinants. PLoS Pathog. 8:e1002578. doi: 10.1371/journal.ppat.1002578
Li, L., and Dongari-Bagtzoglou, A. (2009). Epithelial GM-CSF induction by Candida glabrata. J. Dent. Res. 88, 746–751. doi: 10.1177/0022034509341266
Linden, J. R., Maccani, M. A., Laforce-Nesbitt, S. S., and Bliss, J. M. (2010). High efficiency opsonin-independent phagocytosis of Candida parapsilosis by human neutrophils. Med. Mycol. 48, 355–364. doi: 10.1080/13693780903164566
Lionakis, M. S., and Netea, M. G. (2013). Candida and Host determinants of susceptibility to invasive candidiasis. PLoS Pathog. 9:e1003079. doi: 10.1371/journal.ppat.1003079
Lowman, D. W., Greene, R. R., Bearden, D. W., Kruppa, M. D., Pottier, M., Monteiro, M. A., et al. (2014). Novel structural features in Candida albicans hyphal glucan provide a basis for differential innate immune recognition of hyphae versus yeast. J. Biol. Chem. 289, 3432–3443. doi: 10.1074/jbc.M113.529131
Marakalala, M. J., Vautier, S., Potrykus, J., Walker, L. A., Shepardson, K. M., Hopke, A., et al. (2013). Differential adaptation of Candida albicans in vivo modulates immune recognition by dectin-1. PLoS Pathog. 9:e1003315. doi: 10.1371/journal.ppat.1003315
Merseguel, K. B., Nishikaku, A. S., Rodrigues, A. M., Padovan, A. C., e Ferreira, R. C., Salles de Azevedo Melo, A., et al. (2015). Genetic diversity of medically important and emerging Candida species causing invasive infection. BMC Infect. Dis. 15:57. doi: 10.1186/s12879-015-0793-3
Netea, M. G., Gow, N. A. R., Joosten, L. A. B., Verschueren, I., van der Meer, J. W. M., and Kullberg, B. J. (2010). Variable recognition of Candida albicans strains by TLR4 and lectin recognition receptors. Med. Mycol. 48, 897–903. doi: 10.3109/13693781003621575
Netea, M. G., Joosten, L. A. B., van der Meer, J. W. M., Kullberg, B.-J., and van de Veerdonk, F. L. (2015). Immune defence against Candida fungal infections. Nat. Rev. Immunol. 15, 630–642. doi: 10.1038/nri3897
Papon, N., Courdavault, V., Clastre, M., and Bennett, R. J. (2013). Emerging and emerged pathogenic Candida species: beyond the Candida albicans Paradigm. PLoS Pathog. 9:e1003550. doi: 10.1371/journal.ppat.1003550
Pfaller, M. A., and Diekema, D. J. (2007). Epidemiology of invasive candidiasis: a persistent public health problem. Clin. Microbiol. Rev. 20, 133–163. doi: 10.1128/CMR.00029-06
Plato, A., Hardison, S. E., and Brown, G. D. (2015). Pattern recognition receptors in antifungal immunity. Semin. Immunopathol. 37, 97–106. doi: 10.1007/s00281-014-0462-4
Rieber, N., Brand, A., Hector, A., Graepler-Mainka, U., Ost, M., Schäfer, I., et al. (2013). Flagellin induces myeloid-derived suppressor cells: implications for Pseudomonas aeruginosa infection in cystic fibrosis lung disease. J. Immunol. 190, 1276–1284. doi: 10.4049/jimmunol.1202144
Rieber, N., Singh, A., Öz, H., Carevic, M., Bouzani, M., Amich, J., et al. (2015). Pathogenic fungi regulate immunity by inducing neutrophilic myeloid-derived suppressor cells. Cell Host Microbe 17, 507–514. doi: 10.1016/j.chom.2015.02.007
Saijo, S., Ikeda, S., Yamabe, K., Kakuta, S., Ishigame, H., Akitsu, A., et al. (2010). Dectin-2 recognition of α-mannans and induction of Th17 cell differentiation is essential for host defense against Candida albicans. Immunity 32, 681–691. doi: 10.1016/j.immuni.2010.05.001
Saijo, S., and Iwakura, Y. (2011). Dectin-1 and Dectin-2 in innate immunity against fungi. Int. Immunol. 23, 467–472. doi: 10.1093/intimm/dxr046
Seider, K., Brunke, S., Schild, L., Jablonowski, N., Wilson, D., Majer, O., et al. (2011). The facultative intracellular pathogen Candida glabrata subverts macrophage cytokine production and phagolysosome maturation. J. Immunol. 187, 3072–3086. doi: 10.4049/jimmunol.1003730
Svobodová, E., Staib, P., Losse, J., Hennicke, F., Barz, D., and Józsi, M. (2012). Differential interaction of the two related fungal species Candida albicans and Candida dubliniensis with human neutrophils. J. Immunol. 189, 2502–2511. doi: 10.4049/jimmunol.1200185
Taylor, P. R., Tsoni, S. V., Willment, J. A., Dennehy, K. M., Rosas, M., Findon, H., et al. (2007). Dectin-1 is required for β-glucan recognition and control of fungal infection. Nat. Immunol. 8, 31–38. doi: 10.1038/ni1408
Tebartz, C., Horst, S. A., Sparwasser, T., Huehn, J., Beineke, A., Peters, G., et al. (2014). A major role for myeloid-derived suppressor cells and a minor role for regulatory T cells in immunosuppression during Staphylococcus aureus Infection. J. Immunol. 194, 1100–1111. doi: 10.4049/jimmunol.1400196
Tóth, A., Csonka, K., Jacobs, C., Vágvölgyi, C., Nosanchuk, J. D., Netea, M. G., et al. (2013). Candida albicans and Candida parapsilosis induce different T-cell responses in human peripheral blood mononuclear cells. J. Infect. Dis. 208, 690–698. doi: 10.1093/infdis/jit188
Tramsen, L., Beck, O., Schuster, F. R., Hunfeld, K.-P., Latgé, J.-P., Sarfati, J., et al. (2007). Generation and characterization of anti-Candida T cells as potential immunotherapy in patients with Candida infection after allogeneic hematopoietic stem-cell transplant. J. Infect. Dis. 196, 485–492. doi: 10.1086/519389
van de Veerdonk, F. L., Kullberg, B. J., and Netea, M. G. (2012). Adjunctive immunotherapy with recombinant cytokines for the treatment of disseminated candidiasis. Clin. Microbiol. Infect. 18, 112–119. doi: 10.1111/j.1469-0691.2011.03676.x
Vazquez, J. A., Gupta, S., and Villanueva, A. (1998). Potential utility of recombinant human GM-CSF as adjunctive treatment of refractory oropharyngeal candidiasis in AIDS patients. Eur. J. Clin. Microbiol. Infect. Dis. 17, 781–783. doi: 10.1007/s100960050185
Wheeler, R. T., Kombe, D., Agarwala, S. D., and Fink, G. R. (2008). Dynamic, morphotype-specific Candida albicans β-glucan exposure during infection and drug treatment. PLoS Pathog. 4:e1000227. doi: 10.1371/journal.ppat.1000227
Zelante, T., Iannitti, R., De Luca, A., and Romani, L. (2011). IL-22 in antifungal immunity. Eur. J. Immunol. 41, 270–275. doi: 10.1002/eji.201041246
Keywords: Candida, anti-fungal immunity, myeloid-derived suppressor cells, MDSCs, T-cell suppression, Dectin-1, Dectin-2
Citation: Singh A, Lelis F, Braig S, Schäfer I, Hartl D and Rieber N (2016) Differential Regulation of Myeloid-Derived Suppressor Cells by Candida Species. Front. Microbiol. 7:1624. doi: 10.3389/fmicb.2016.01624
Received: 01 August 2016; Accepted: 29 September 2016;
Published: 13 October 2016.
Edited by:
Oscar Zaragoza, Instituto de Salud Carlos III, SpainReviewed by:
Attila Gacser, University of Szeged, HungaryJeanette Wagener, University of Aberdeen, UK
Copyright © 2016 Singh, Lelis, Braig, Schäfer, Hartl and Rieber. This is an open-access article distributed under the terms of the Creative Commons Attribution License (CC BY). The use, distribution or reproduction in other forums is permitted, provided the original author(s) or licensor are credited and that the original publication in this journal is cited, in accordance with accepted academic practice. No use, distribution or reproduction is permitted which does not comply with these terms.
*Correspondence: Anurag Singh, anurag.singh@med.uni-tuebingen.de
†These authors have contributed equally to this work.