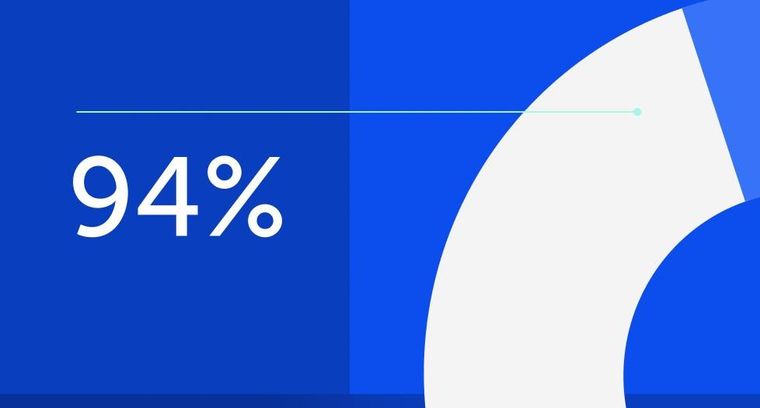
94% of researchers rate our articles as excellent or good
Learn more about the work of our research integrity team to safeguard the quality of each article we publish.
Find out more
ORIGINAL RESEARCH article
Front. Mar. Sci., 26 May 2020
Sec. Marine Ecosystem Ecology
Volume 7 - 2020 | https://doi.org/10.3389/fmars.2020.00347
This article is part of the Research TopicStudying the Biology of Aquatic Animals through Calcified StructuresView all 21 articles
Variations in otolith elemental composition are widely used to reconstruct fish movements. However, reconstructing habitat use and environmental histories of fishes within estuaries is still a major challenge due to the dynamic nature of these coastal environments. In this study, we performed a laboratory experiment to investigate the effects of variations in salinity (three levels; 5, 18, 30) and temperature (two levels; 16, 21°C) on the otolith elemental composition (Mg:Ca, Mn:Ca, Sr:Ca, Ba:Ca) of juvenile Senegalese sole Solea senegalensis. Temperature and salinity treatments mirrored the natural conditions of the estuarine habitats occupied by juvenile Senegalese sole, thereby providing information on the applicability of otolith microchemistry to reconstruct habitat use patterns within estuarine nurseries, where individual fish move across complex salinity and temperature gradients. While Sr:Ca and Ba:Ca in otoliths were both positively related to salinity, no temperature effect was observed. Partition coefficients, proxies for element incorporation rates increased with increasing salinity for Sr (DSr) and Ba (DBa). In contrast, salinity and temperature had little influence on otolith Mn:Ca and Mg:Ca, supporting physiological control on the incorporation of these elements. Our results are a stepping stone for the interpretation of otolith chemical profiles for fish collected in their natural habitats and contribute to better understanding the processes involved in otolith element incorporation.
Estuaries are key nursery areas for many marine fish (Beck et al., 2001; Sheaves et al., 2006; Nagelkerken et al., 2013), including flatfishes (Le Pape et al., 2003; Martinho et al., 2010; Vasconcelos et al., 2011; Freitas et al., 2012). These species often have complex life cycles, which include migrations between coastal areas and inshore nurseries of up to 100s of kilometers. In this sense, development of management and protection plans should take into account connectivity between the distinct ontogenetic habitats, as connectivity is directly linked to population dynamics, productivity and resilience to harvest (Cowen et al., 2000; Thorrold et al., 2001; Pihl et al., 2002).
Otolith chemistry has become a benchmark for reconstructing fish movements and migrations (Elsdon et al., 2008; Izzo et al., 2018; Walther, 2019). The use of otoliths as natural tags is feasible because these paired calcium carbonate structures are metabolically inert, grow continuously forming daily and annual patterns, and incorporate trace elements as they grow without evidence of resorption or reworking of the chemical composition (Campana, 1999; Elsdon et al., 2008). The elemental composition of otoliths is generally influenced by the concentration of the elements in the surrounding water, providing a natural tag in which all individuals are marked similarly, even though individual-based physiological and genetic processes take part (Sturrock et al., 2015; Izzo et al., 2018). For reconstructing migration patterns between the sea and the river, Strontium (Sr) and Barium (Ba) are commonly used as their concentrations tend to inversely related in fresh and saltwater (e.g., Walther and Limburg, 2012; Smith and Kwak, 2014). Yet, a suite of other elements, such as magnesium (Mg) or manganese (Mn), also reflect multiple environmental and physiological responses of individual fish to the surrounding ambient water conditions and can aid in environmental reconstructions (Martin and Thorrold, 2005; Tanner et al., 2013a).
Presently, there is still some debate regarding the use of otolith chemistry to discern movements and habitat use within complex and dynamic environments such as estuaries (Elsdon and Gillanders, 2004; Reis-Santos et al., 2018; Williams et al., 2018; Walther, 2019). Several factors may contribute to variations in otolith elemental composition, particularly environmental factors such as water temperature, salinity and ambient element concentrations, which can play an important role in the control of element uptake, as well as several intrinsic factors such as the genetic background, fish diet and physiological processes (Clarke et al., 2011; Woodcock et al., 2012; Sturrock et al., 2014; Grammer et al., 2017; Izzo et al., 2018). In addition, variations in otolith elemental composition also seem to be species-specific (Reis-Santos et al., 2008; Chang and Geffen, 2012).
Water temperature and salinity are known to influence the concentration of elements in the water, whose variation is often inter-dependent. Moreover, the effects of salinity on the elemental ratios in otoliths generally rises with increasing temperature (Elsdon and Gillanders, 2002), although differential results of temperature response among species and elements have been observed (see Miller, 2009; Izzo et al., 2018). Hence, before using otolith chemistry to reconstruct fish life history or habitat use patterns, we need to evaluate the degree to which movements along salinity gradients within estuaries can be determined with accuracy, and unravel the role of temperature on otolith elemental composition (Miller, 2011; Reis-Santos et al., 2013; Tanner et al., 2013a).
Considering that juveniles of many commercially important fishes use estuaries as nursery areas over prolonged periods of their lives (months to years; Gillanders et al., 2003), a better understanding of the ability of otolith chemistry to discern intra-estuarine habitat use patterns can provide key information for species management and habitat conservation. Thus, the objective of this work was to evaluate the effects of salinity and water temperature on otolith chemical composition (Mg:Ca, Mn:Ca, Sr:Ca, Ba:Ca).
In a controlled laboratory experiment, juveniles of the flatfish Senegalese sole Solea senegalensis Kaup, 1858 were exposed to combinations of three salinity and two temperature treatments, representative of estuarine environments, to evaluate if otolith chemistry can accurately reflect movement and habitat use within estuarine nursery areas.
The Senegalese sole is a flatfish of high commercial value and widely distributed along the eastern Atlantic from the Bay of Biscay to Senegal, and in the western Mediterranean. Spawning and larval development takes place in shelf waters, with metamorphosis and shift to a benthic life form occurring before recruitment to estuarine nursery grounds, where juveniles remain for up to 2 years (Cabral and Costa, 1999; Primo et al., 2013). Despite key estuarine areas for this species having been identified and population connectivity between estuaries and coastal areas quantified (Vasconcelos et al., 2010; Tanner et al., 2013b), there still is a clear lack of detailed knowledge on juvenile habitat use patterns within estuaries.
Solea senegalensis juveniles with an average total length (TL) of 9.8 cm (± 1.1 cm standard deviation), approximately 7 months old, were obtained from a hatchery (where they were born) and acclimatized to laboratory conditions over 30 days in 200 L tanks equipped with aeration and filtration, at constant temperature (19°C) and salinity (20; reconstructed with artificial sea salt TMC REEF – Premium REEF Salt) levels. Afterward, all fish (n = 93) were randomly distributed to 18 tanks (20 L) at a density of 6 to 7 individuals per tank to initiate the acclimatization to the test conditions. These tanks were also equipped with aeration and filtration systems and had the same temperature and salinity (19 and 20°C, respectively) to minimize the stress-induced from fish handling and rearing conditions. Over 21 days, water temperature and salinity in the tanks were gradually altered to acclimatize fish to the experimental temperature (16, 21°C) and salinity (5, 18, and 30) combined treatments. Photoperiod was set at 12 h day (dim) and 12 h night (dark) daily cycles. The final experimental design was composed of six test conditions (2 temperatures × 3 salinities) with three replicates each. Salinity and temperature ranges of the experiment represent typical environmental conditions that S. senegalensis juveniles experience over spring and summer during their estuarine residency and among intra-estuarine nursery areas (Vinagre et al., 2009; Vasconcelos et al., 2010).
During the experiment, salinity was controlled by adding fresh dechlorinated water with artificial marine salt (TMC REEF- Premium REEF Salt) to match each test condition (5, 18, and 30). Experiments were run in a room with controlled temperature, which was maintained constant at 21°C, with the low-temperature treatments achieved via an open water bath system connected to a chiller kept stable at 16°C. Approximately 40% of the water in each tank was changed every 3 days. Salinity, water temperature (°C), oxygen (mg/L) and pH were measured daily, whilst ammonia, nitrite and nitrate levels were analyzed weekly throughout the experiment to ensure the best possible water quality.
Fish were maintained under experimental conditions for 98 days and fed daily with specialized food pellets (provided by the hatchery) until apparent satiation, corresponding to 1–2% of their body weight. Fish feed was the same given at the hatchery. Excess food and debris were siphoned within 1 h. All fish were measured for total length (TL, cm) in the beginning and at the end of the experiment, to determine the respective mean growth rate (cm day–1) per treatment.
This study was carried out following the principles of the Basel Declaration and recommendations of Directive 2010/63/EU and Art° 44 of the Decree-Law no. 113/2013 of 7th August. The protocol was approved by the Portuguese National Authority for Animal Health (DGAV; Ref. 0421/000/000/2017).
On day seven and approximately every 2 weeks from then on, duplicate water samples from each treatment were collected with polypropylene syringes to characterize water elemental concentrations. Over the course of the experiment there were a total of eight water collection times per treatment. Water samples were filtered (GF/F, 0.2 μm) into 60 mL flasks and acidified with concentrated ultrapure nitric acid (HNO3) in a 1:50 ratio and stored at 4°C until analysis. Prior to elemental quantification by inductively coupled plasma mass spectrometry (ICP-MS), 3 mL of each water sample were further acidified (1:10) by adding 3 mL of a previously distilled 10% nitric acid solution. Water samples were analyzed for 24Mg, 43Ca, 55Mn, 88Sr, and 138Ba using a Thermo Fisher Scientific Model iCAP Q spectrophotometer (Bremen, Germany). The calibration of the ICP-MS measurements was done using a five-point calibration curve per element. Standard solutions were prepared with dilutions of a multi-element standard (92091, Periodic table mix 1 for ICP, Sigma-Aldrich), and a standard curve was generated per element at 0, 1, 10, 100, 1000, and 2000 ppm concentrations. Blank controls consisted of ultrapure water acidified with HNO3 at 10%. Standards and blanks were analyzed at the beginning and throughout the session. The detection limits were 1.1213, 2.0350, 0.0122, 0.0188 and 0.0380 ppb for 24Mg, 43Ca, 55Mn, 88Sr, and 138Ba, respectively.
After the 98-day experimental procedure, all fish were measured and weighed, and their sagittal otoliths removed, cleaned with distilled water and the left otolith mounted on microscope slides with cyanoacrylate glue. Subsequently, otoliths were polished with 3 μm lapping film under a binocular lens to expose an even surface between the core and the edge of the otolith. Otoliths were then sonicated in ultrapure water until they detached from the microscope slide, and were subsequently dried in a laminar flow cabinet and attached to a new microscope slide using double-sided tape (see Tanner et al., 2013a).
Otolith chemical analysis followed the procedure in Reis-Santos et al. (2018). Briefly, a 213 nm high-performance UV (Nd: YAG) laser microprobe coupled to an Agilent 7900 inductively coupled plasma mass spectrometer (ICP-MS) was used to quantify 24Mg, 55Mn,44Ca, 88Sr, and 138Ba concentrations in otoliths. Ca was used as an internal standard to normalize variations in ablation yield (Yoshinaga et al., 2000). Three spots with a diameter of 25 μm were ablated (power ∼10 J cm–2, and fluence 5 Hz) on the marginal edge of all otoliths. These spot analyses targeted otolith material deposited during the experimental period and represent recent elemental incorporation. All spots were pre-ablated to remove any potential surface contamination. Laser ablations occurred in a sealed chamber with resulting analyte transported to the ICP-MS via a smoothing manifold in an argon (Ar) and helium (He) stream. A glass certified reference material (NIST 612 – National Institute of Standards and Technology) was analyzed at the start and end of each session and after every 10 otoliths to correct for mass bias and machine drift. External precision (% relative standard deviation) was determined based on a calcium carbonate certified reference material, MACS-3 (United States Geological Survey) (RSD ≤ 5%). All data reduction, including background corrections, limits of detections and mass count to ppm conversions were done using Iolite software (v 3.1) (Paton et al., 2011).
Variation in growth rates (cm day–1) per treatment was analyzed with permutational analysis of variance (PERMANOVA), using PRIMER v6 & PERMANOVA + v1 software (PRIMER-E, Ltd.), based on Euclidean distance matrices with unrestricted permutations. Temperature and salinity were treated as fixed categorical factors, and replicate tanks were included as a random factor nested within both fixed factors. Pairwise post hoc tests were performed whenever significant differences were found.
Otolith elemental concentration (Me, ppm) values were converted to molar concentrations and standardized to calcium (Me:CaOtolith), and all further data analyses were carried out on the Me:CaOtolith data. The same procedure was adopted for the water samples (Me:CaWater). The Me:CaOtolith values were calculated per individual, and the three tank averages were used as replicates for each experimental condition. Partition coefficients of an element (DMe) depict otolith chemical concentrations relative to water chemical concentrations, and reflect the fractionation and physiological regulation of element incorporation into biogenic carbonates (Morse and Bender, 1990; Reis-Santos et al., 2013). DMe were calculated for each element dividing the Me:CaOtolith by the Me:CaWater. The partition coefficient (DMe) provides an easy and useful metric to compare incorporation of elements in various treatments, since it describes the chemical concentrations of otoliths in relation to the chemical concentration in the water, and is a valuable tool to evaluate abiotic and biotic effects on otolith elemental incorporation and to perform comparisons between species and studies.
Differences among treatments in Me:CaWater, Me:CaOtolith and DMe were analyzed separately with permutational analysis of variance (PERMANOVA), using PRIMER v6 & PERMANOVA + v1 software (PRIMER-E, Ltd.), based on Euclidean distance matrices with unrestricted permutations of log(x + 1) transformed data. Salinity and temperature were treated as fixed factors, and replicate tanks as random factors nested within both fixed factors (only for Me:CaOtolith and DMe). Pearson correlation analyses were used to determine the relationship between the elemental ratio in the rearing water and the elemental ratio in the juvenile S. senegalensis otoliths. A significance level of 0.05 was considered for all test procedures.
Temperature and salinity reflected the required experimental levels and were kept stable throughout the experiment (Table 1). Elemental ratios in the water were within the range found in a temperate estuary in Portugal (Tejo; see Tanner et al., 2013a) and were influenced by the different salinity treatments, but not by temperature. Specifically, Mg:CaWater decreased with increasing salinity, being significantly higher at salinity five treatments than at 18 and 30, but with no distinction between the two latter (P(perm) = 0.001 and P(perm) = 0.001, respectively). Mn:CaWater increased with salinity, being significantly lower at salinity five than at the 18 and 30 treatments, also which did not vary from one another (P(perm) = 0.001 and P(perm) = 0.001, respectively). Sr:CaWater was constant among all salinity and temperature treatments, while Ba:CaWater decreased with increasing salinity, with significant differences among all treatments (P(perm) = 0.001, P(perm) = 0.001, and P(perm) = 0.007, respectively) (Tables 1, 2).
Table 1. Summary of the experimental conditions of salinity and temperature along with water Me:Ca ratios in each treatment, showing mean and standard deviation of water salinity, temperature (°C), and Mg:CaWater (mol mol–1), Mn:CaWater (μmol mol–1), Sr:CaWater (mmol mol–1) and Ba:CaWater (μmol mol–1) ratios.
Table 2. Results of the permutational analysis of variance (PERMANOVA) investigating the effects of salinity and temperature on the Mg:CaWater, Mn:CaWater, Sr:CaWater, and Ba:CaWater of the rearing conditions.
Juvenile S. senegalensis increased in total length in all treatments during the 98-day experimental procedure: initial average TL 9.8 ± 1.1 cm; final average TL 11.2 ± 1.1 cm (Figure 1). There were no differences in growth rates among treatments, with only a significant tank effect (Table 3), which was most probably due to a single tank with lower growth rates (one of the three replicates of the 21°C, 30 salinity treatment).
Figure 1. Average total length of juvenile Solea senegalensis at the beginning (light gray) and at the end (dark gray) of the experimental procedure for all treatments.
Table 3. Results of the permutational analysis of variance (PERMANOVA) investigating the effects of salinity and temperature on the growth rates of juvenile Solea senegalensis.
Otolith elemental concentrations varied according to the different salinity treatments, but not with temperature (Figure 2 and Table 4). No effects of temperature, salinity or their interaction were observed for Mn:CaOtolith and Mg:CaOtolith. In contrast, a significant effect of salinity was observed for Sr:CaOtolith, where pairwise comparisons found that Sr:CaOtolith in the highest (30) salinities were higher than in the medium (18) and lowest salinities (5) (P(perm) = 0.004 and P(perm) = 0.016, respectively). No significant differences were observed between the low (5) and medium (18) salinity treatments. A similar result was obtained for Ba:CaOtolith, which increased with salinity (Figure 2 and Table 4). Post hoc pairwise tests found significant differences between salinities 5 and 30, as well as between 18 and 30 (P(perm) = 0.006 and P(perm) = 0.005, respectively) (Figure 2 and Table 4).
Figure 2. Mean and standard error of Mg:CaOtolith, Mn:CaOtolith, Sr:CaOtolith, and Ba:CaOtolith across the salinity (5, 18, 30) and temperature (16 and 21°C) experimental treatments, which are indicated by the different colors: blue colors – 16°C, red colors – 21°C; light tone – 5 salinity, medium tone – 18 salinity, dark tone – 30 salinity.
Table 4. Results of the permutational analysis of variance (PERMANOVA) investigating the effects of salinity and temperature on log transformed Mg:CaOtolith, Mn:CaOtolith, Sr:CaOtolith and Ba:CaOtolith of juvenile Solea senegalensis otoliths.
The partition coefficients for magnesium (DMg) were extremely low, ranging between 3.48 × 10–6 ± 6.89 × 10–7 and 4.28 × 10–6 ± 1.56 × 10–6, with no differences among treatments. For manganese, DMn values ranged between 0.02 ± 0.01 and 0.17 ± 0.07. An effect of salinity was observed for DMn, with post hoc pairwise tests identifying a significant increase between salinities 5 and 18, as well as between 5 and 30 (P(perm) = 0.001 and P(perm) = 0.002, respectively). For strontium, DSr ranged between 0.15 ± 0.02 and 0.22 ± 0.03 and with a significant positive effect of salinity between 5 and 30, and between 18 and 30 (P(perm) = 0.001 and P(perm) = 0.002, respectively). For barium, DBa ranged between 0.02 ± 0.01 and 0.13 ± 0.03, and showed a significant increase between salinities 5 and 18, as well as between 5 and 30 (P(perm) = 0.007 and P(perm) = 0.001, respectively) (Figure 3 and Table 5).
Figure 3. Mean and standard error of partition coefficients of magnesium (DMg), manganese (DMn), strontium (DSr) and barium (DBa), across the salinity (5, 18, 30) and temperature (16 and 21°C) experimental treatments, which are indicated by the different colors: blue colors – 16°C, red colors – 21°C; light tone – 5 salinity, medium tone – 18 salinity, dark tone – 30 salinity.
Table 5. Results of the permutational analysis of variance (PERMANOVA) investigating the effects of salinity and temperature on the partition coefficients of magnesium (DMg), manganese (DMn), strontium (DSr) and barium (DBa).
There were no significant relationships between water and otolith elemental concentrations for all elements (p > 0.1) (Figure 4).
Figure 4. Mean and standard error of the relationship between Mg:CaOtolith and Mg:CaWater, Mn:CaOtolith and Mn:CaWater, Sr:CaOtolith and Sr:CaWater, and Ba:CaOtolith and Ba:CaWater across the salinity (5, 18, 30) and temperature (16 and 21°C) experimental treatments, which are indicated by the different colors: blue colors – 16°C, red colors – 21°C; light tone – 5 salinity, medium tone – 18 salinity, dark tone – 30 salinity.
Resolving estuarine habitat use with otolith chemistry can provide key knowledge on species habitat use, movements and connectivity particularly for species that use estuaries as nursery grounds. Such knowledge can represent important information for protection and management programs. The main finding of our work was related to the ability to use otolith Sr:Ca and Ba:Ca of juvenile Senegalese sole S. senegalensis to distinguish between the tested low and high salinity environments, irrespective of the tested temperature regimes. Yet, we were not able to successfully discern the intermediate salinity tested. Therefore, whilst our results support the use of otolith chemistry to disentangle brackish waters, namely oligohaline (salinity ± 5) from polyhaline waters (salinity ± 30), there were limitations differentiating mesohaline waters (salinity ± 18).
We observed no clear temperature effect on Mg, Mn, Sr, and Ba elemental concentration in juvenile S. senegalensis otoliths, which follows findings from other fish species (e.g., Gallahar and Kingsford, 1996; Elsdon and Gillanders, 2004; Martin et al., 2004; Martin and Thorrold, 2005; Mazloumi et al., 2017). Despite the 5°C difference in treatments, the experimental temperatures chosen (16 and 21°C) are within the range this species typically experience in temperate estuaries and coastal zones, which may explain the little effect on the elemental assimilation rates in the otolith matrix. Nonetheless, it is important to bear in mind that the relationship between otolith chemistry and temperature varies among species, families and life history traits (Collingsworth et al., 2010; Mazloumi et al., 2017). Overall, the high degree of inter-specific variability in the isolated and interactive effects of temperature and salinity on element uptake into otoliths hinders the development of universal models based on otolith chemistry to understand life history patterns (Stanley et al., 2015).
We also found no significant differences between salinity and temperature treatments for both Mg:CaOtotlith and Mn:CaOtotlith in juvenile Senegalese sole, in agreement with other laboratory experiments (Elsdon and Gillanders, 2002; Martin and Wuenschel, 2006; DiMaria et al., 2010). For magnesium, the lack of correlation between the chemical composition of otoliths and water likely indicates that this element is under significant physiological control (Woodcock et al., 2012; Grammer et al., 2017), and supported by the fact Mg is very abundant in the marine environment and required in many metabolic pathways, regulating cell function, bone development and growth. According to Elsdon and Gillanders (2003b), manganese is key to multiple metabolic processes, yet it can become toxic at high levels, and thus it is highly regulated within the organism. In addition, the presence of Mn is also essential for the biomineralization process in otoliths (Thomas et al., 2018). The use of Mn:CaOtotlith as a marker to understand life history patterns has not yet been thoroughly validated, given the likely confounding effects of diet composition, temperature variations, physiology and growth (Pentreath, 1976; Miller, 2009; Sturrock et al., 2015). Our results were not able to disentangle the effects of salinity and temperature on Mn:CaOtotlith, which is indicative of physiological regulation. Overall, although still poorly understood, the incorporation of Mn into otoliths is presumed to be essentially under metabolic control, rather than determined by environmental factors (Tanner et al., 2012; Limburg et al., 2015; Sturrock et al., 2015). While there were no differences in the elemental composition of Mg:CaOtolith and Mn:CaOtolith, there was a significant effect of salinity on the incorporation of manganese (DMn), which mainly reflected the different environmental levels in the rearing water and further highlights the role of physiology in regulating otolith Mn. Earlier works have suggested that Mn:CaOtotlith has potential as an environmental marker of exposure to hypoxia (Mohan et al., 2014; Limburg et al., 2015). Further explored, this facet of Mn and otolith chemistry may provide useful information on fish populations in estuaries, since hypoxic events are increasing in time and space throughout the world, particularly during the warm season (Breitburg et al., 2018; Schmidt et al., 2019).
The use of Sr as a recorder of past salinity history is based on the premise that it replaces Ca in otolith aragonite as fish grow, and especially that there is generally more Sr in seawater than in freshwater (Campana, 1999; Elsdon et al., 2008; Doubleday et al., 2014). In this work, we found a positive influence of salinity on Sr:CaOtolith, as it was higher in the treatment close to marine water (30) when compared with the brackish treatment (18) and the one close to freshwater (5). Our results generally agree with experimental studies on other fish species based on salinity and temperature manipulations (Martin and Wuenschel, 2006; Stanley et al., 2015), but also contrast with others, in which a negative relationship with salinity was reported (Elsdon and Gillanders, 2002; Mazloumi et al., 2017). Considering that most elemental uptake into otoliths is usually acknowledged as species-specific (Swearer et al., 2003; Reis-Santos et al., 2008; Chang and Geffen, 2012), our results also support the use of Sr as a marker for reconstructing past habitat use, preferably between high and low salinities. However, Sr:CaOtolith could not resolve the lower and brackish salinities. This limits its application to discern intra-estuarine habitat use in marine juvenile fishes, particularly if they spend a significant amount of time at mid and upper areas of estuaries, which is the case for the Senegalese sole (Vinagre et al., 2009; Primo et al., 2013). One possible reason is that Sr can be highly variable in estuaries due to tidal mixing with freshwater discharge, suggesting that the increase in Sr:Ca with salinity in these waters might not follow a linear trend (Phillis et al., 2011; Walther and Nims, 2014). Indeed, key to discerning habitat use by marine fish in transitional ecosystems is the elemental mixing kinetics (Walther, 2019), which depend greatly on the hydrodynamics and endmember contributions of each system. The temporal lag between exposure to a water mass (either freshwater, brackish or marine) and the incorporation in otoliths is also an important feature for the correct use of otolith chemistry for tracking fish movements (Elsdon and Gillanders, 2005c; Izzo et al., 2018).
Similarly to Sr:CaOtolith, Ba:CaOtolith also increased with salinity, with higher values in the marine-like treatment (30) than in the near freshwater (5) and brackish (18) experimental conditions. Barium has been used typically in combination with Sr to unravel environmental and migratory histories (Bath et al., 2000; Elsdon and Gillanders, 2002; de Vries et al., 2005; Reis-Santos et al., 2013), given the natural inverse concentration of Sr and Ba in riverine and marine waters (Turner et al., 1981; Coffey et al., 1997; Elsdon and Gillanders, 2005a). Nevertheless, akin to Sr levels, our results for Ba revealed a potential shortcoming due to the inability to resolve near freshwater and brackish environments. Accordingly, Sr:CaOtolith and Ba:CaOtolith in juvenile S. senegalensis can only be used to accurately resolve between low and high salinity environments, without the possibility to resolve habitat use patterns at finer spatial scales.
The incorporation of Sr and Ba, given by their partition coefficients (DMe), was positively influenced by salinity, and was a good metric for comparing the relation between elemental concentrations in the otoliths and in the water (as in Miller, 2009), even if there were no significant differences in Sr:CaWater between treatments. Although the element concentrations in the otoliths were one (Sr) and two (Ba) orders of magnitude lower than the experimental medium, they still reflected the existing variations in salinity, and were within the range reported for other fish species (Elsdon and Gillanders, 2003a; Reis-Santos et al., 2012, 2013). An increase in Sr:CaOtolith and DSr with salinity suggests that the incorporation of Sr may be facilitated by higher salinity, as there were no differences in Sr:CaWater of the rearing treatments. On the other hand, a decrease in Ba:CaWater with increasing salinity resulted in higher elemental incorporation in otoliths and an increase in the DBa, which has been suggested to occur at higher Sr:Ca levels in brackish waters, suggesting facilitation of Ba uptake (de Vries et al., 2005). In elements with high physiological regulation such as Mg and Mn, the use of partition coefficients may be misleading as a series of complex but still poorly understood mechanisms influence the chemical incorporation through multiple osmoregulatory barriers between the environment and the endolymph. The use of partition coefficients (DMe) whilst of great applicability in experimental studies, may have some limitations for in situ evaluations, particular in such dynamic and complex environments as estuaries, since sudden changes in salinity and/or temperature may not be reflected in the incorporation of elements, thus being more useful for prolonged exposures.
In this work, we did not find a significant correlation between element concentration in the otolith (Me:CaOtolith) and the water (Me:CaWater) for any of the considered elements. The complex nature of the relationship between Me:CaOtolith and Me:CaWater is emphasized by the disparity in results from previous studies mostly on Sr:Ca, Ba:Ca and water chemistry, which described linear (Martin and Wuenschel, 2006; Macdonald and Crook, 2010), exponential (Dorval et al., 2007) and even no relationships (Elsdon and Gillanders, 2005b; Mazloumi et al., 2017) as in the present study, and can be related with the different mode of element uptake by each species. Element uptake is a multi-stage process, characterized by a succession of barriers with varying degrees of inter-dependence from the ambient water to blood plasma through gills, endolymph and finally into the otolith (Campana, 1999). To our knowledge, few experimental procedures on otolith chemistry have been performed with flatfishes (e.g., Sturrock et al., 2014), whose particular ecological characteristics (life history, metamorphosis, physiology) might drive some of the differences observed in terms of Me:CaOtolith and Me:CaWater relationships. In addition, the lack of discrimination between water and otolith composition may also be due to the use of a reduced range of temperatures (Elsdon and Gillanders, 2002) and/or elemental concentrations in water (Elsdon and Gillanders, 2003a).
Despite the limitations described above, this study is a stepping-stone for the interpretation of otolith chemical profiles and for the reconstruction of estuarine habitat use. Very few studies have focused on flatfishes, whose specific ecological features might be responsible for the distinct patterns observed, and several questions arose that foster further research to improve our ability to reconstruct past estuarine habitat use by fishes. Among others, these include experimental work based on a wider range of temperatures covering the range of wintering conditions, as well as establishing a parallel with field conditions, since the complex dynamics and interactions between the chemical elements in the environment might lead to discrepancies with laboratory results (Elsdon and Gillanders, 2005b; Dorval et al., 2007; Tanner et al., 2013a; Williams et al., 2018). Additional research should address element mixing dynamics between heterogenous water masses, as well as the kinetics of element incorporation, ion transport and internal regulation in fish, with the ultimate goal of elucidating the suitability of the selected elements as environmental tracers. Indeed, the suite of factors that govern otolith elemental composition from that found in the environment are complex and, in most cases, poorly understood (Izzo et al., 2018; Reis-Santos et al., 2018; Walther, 2019). Apart from salinity, temperature and ambient elemental concentration, other examples of biological factors play a role in determining the elemental concentrations in the otolith, such as genetic profiles, size, sex or age (Barnes and Gillanders, 2013; Izzo et al., 2018; Reis-Santos et al., 2018; Walther, 2019). These are factors that should be considered early in the experimental design to control for their confounding effects. Accordingly, all fish in our experiment were obtained from the same hatchery, brood stock, age and size range to minimize potential ontogenic and genetic effects, while a commercial feed designed for the selected size range was provided to avoid any potential bias related to dietary variations. The absence of significant differences in growth rates between treatments also contributed to mitigating any other effects related to individual responses to the test conditions. Further studies using prey from the field should be performed to disentangle the possible effects of diet composition on the otolith chemistry of fishes in estuaries.
The measured elemental composition of otoliths of S. senegalensis were linked to the tested environmental factors. In particular, Sr and Ba were suitable elements for the reconstruction of juvenile S. senegalensis movements along natural salinity gradients, but with limited resolving power for finer scale variations, namely between oligo- and mesohaline, and meso- and polyhaline waters. Ultimately, further work should focus on combining laboratory experiments with species-specific information on the elemental incorporation of otoliths and field trials, to better improve our knowledge of the processes involved under natural conditions, as well as the timeframe between element uptake and otolith assimilation.
The datasets generated for this study are available on request to the corresponding author.
The animal study was reviewed and approved by Portuguese National Authority for Animal Health (DGAV; Ref. 0421/000/000/2017).
ST, RV, VF, PR-S, and FM designed the experiment and approach. BP, MN, AV, DC, AP, MP, and FM executed the experiment and laboratory work. PR-S and BG executed the otolith chemical analyses. BP and FM analyzed the data and wrote the manuscript. All authors commented on and approved the final version of the manuscript.
This research was supported by the Portuguese Foundation for Science and Technology (FCT, I.P.) via a post-doctoral grant to PR-S (SFRH/BPD/95784/2013), a Ph.D. grant to AV (SFRH/BD/137862/2018), and researcher contracts to ST (DL57/2016/CP1479/CT0022), VF (DL57/2016/CP1479/CT0024), FM, and AP, in the scope of the framework contract foreseen in the numbers 4, 5, and 6 of the article 23, of the Decree-Law 57/2016, of August 29, changed by Law 57/2017, of July 19. FCT, I.P. also provided support through the research project “Mytag – Integrating natural and artificial tags to reconstruct fish migrations and ontogenetic niche shifts” (PTDC/MAR-EST/2098/2014), under the Project 9471 – Reforçar a Investigação, o Desenvolvimento Tecnológico e a Inovação (Projeto 9471-RIDTI) and subsidized by the European Regional Development Fund (FEDER, POCI-01-0145-FEDER-016787), the Centre for Functional Ecology Strategic Project (UID/BIA/04004/2019) within the PT2020 Partnership Agreement and COMPETE 2020, and the Marine and Environmental Sciences Centre Strategic Project (UID/MAR/04292/2019). Financial support was also provided by FEDER through the project ReNATURE – Valorization of the Natural Endogenous Resources of the Centro Region (Centro 2020, Centro-01-765-0145-FEDER-000007).
The authors declare that the research was conducted in the absence of any commercial or financial relationships that could be construed as a potential conflict of interest.
We are grateful to Ana Varela and João Rito for their help during the experimental setup and laboratory work. We also thank Sarah Gilbert for her assistance and acknowledge Adelaide Microscopy, The University of Adelaide and the Australian Microscopy and Microanalysis Research Facility (AMMRF) for use of equipment.
Barnes, T. C., and Gillanders, B. M. (2013). Combined effects of extrinsic and intrinsic factors on otolith chemistry: implications for environmental reconstructions Ed K. Rose. Can. J. Fish. Aquat. Sci. 70, 1159–1166. doi: 10.1139/cjfas-2012-0442
Bath, G. E., Thorrold, S. R., Jones, C. M., Campana, S. E., McLaren, J. W., and Lam, J. W. H. (2000). Strontium and barium uptake in aragonitic otoliths of marine fish. Geochim. Cosmochim. Acta 64, 1705–1714. doi: 10.1016/S0016-7037(99)00419-6
Beck, M., Heck, K., Able, K., Childers, D., Eggleston, D., Gillanders, B. M., et al. (2001). The identification, conservation, and management of estuarine and marine nurseries for fish and invertebrates. Bioscience 51, 633–641.
Breitburg, D., Levin, L. A., Oschlies, A., Grégoire, M., Chavez, F. P., Conley, D. J., et al. (2018). Declining oxygen in the global ocean and coastal waters. Science 359:eaam7240. doi: 10.1126/science.aam7240
Cabral, H. N., and Costa, M. J. (1999). Differential use of nursery areas within the Tagus estuary by sympatric soles, Solea solea and Solea senegalensis. Environ. Biol. Fish. 56, 389–397.
Campana, S. E. (1999). Chemistry and composition of fish otoliths: pathways, mechanisms and applications. Mar. Ecol. Progr. Ser. 188, 263–297.
Chang, M.-Y., and Geffen, A. J. (2012). Taxonomic and geographic influences on fish otolith microchemistry. Fish Fish. 14, 458–492. doi: 10.1111/j.1467-2979.2012.00482.x
Clarke, L. M., Thorrold, S. R., and Conover, D. O. (2011). Population differences in otolith chemistry have a genetic basis in Menidia menidia. Can. J. Fish. Aquat. Sci. 68, 105–114. doi: 10.1139/F10-147
Coffey, M., Dehairs, F., Collette, O., Luther, G., Church, T., and Jickells, T. (1997). The behaviour of dissolved barium in estuaries. Estuar. Coast. Shelf Sci. 45, 113–121. doi: 10.1006/ecss.1996.0157
Collingsworth, P. D., Van Tassell, J. J., Olesik, J. W., and Marschall, E. A. (2010). Effects of temperature and elemental concentration on the chemical composition of juvenile yellow perch (Perca flavescens) otoliths. Can. J. Fish. Aquat. Sci. 67, 1187–1196. doi: 10.1139/F10-050
Cowen, R. K., Lwiza, K. M. M., Sponaugle, S., Paris, C. B., and Olson, D. B. (2000). Connectivity of marine populations: open or closed? Science 287, 857–859. doi: 10.1126/science.287.5454.857
de Vries, M. C., Gillanders, B. M., and Elsdon, T. S. (2005). Facilitation of barium uptake into fish otoliths: influence of strontium concentration and salinity. Geochim. Cosmochim. Acta 69, 4061–4072. doi: 10.1016/j.gca.2005.03.052
DiMaria, R. A., Miller, J. A., and Hurst, T. P. (2010). Temperature and growth effects on otolith elemental chemistry of larval Pacific cod, Gadus macrocephalus. Environ. Biol. Fish. 89, 453–462. doi: 10.1007/s10641-010-9665-2
Dorval, E., Jones, C. M., Hannigan, R., and Montfrans, J. V. (2007). Relating otolith chemistry to surface water chemistry in a coastal plain estuary. Can. J. Fish. Aquat. Sci. 64, 411–424. doi: 10.1139/f07-015
Doubleday, Z. A., Harris, H. H., Izzo, C., and Gillanders, B. M. (2014). Strontium randomly substituting for calcium in fish otolith aragonite. Analyt. Chem. 86, 865–869. doi: 10.1021/ac4034278
Elsdon, T. S., and Gillanders, B. M. (2002). Interactive effects of temperature and salinity on otolith chemistry: challenges for determining environmental histories of fish. Can. J. Fish. Aquat. Sci. 59, 1796–1808. doi: 10.1139/f02-154
Elsdon, T. S., and Gillanders, B. M. (2003a). Reconstructing migratory patterns of fish based on environmental influences on otolith chemistry. Rev. Fish Biol. Fish. 13, 217–235.
Elsdon, T. S., and Gillanders, B. M. (2003b). Relationship between water and otolith elemental concentrations in juvenile black bream Acanthopagrus butcheri. Mar. Ecol. Prog. Ser. 260, 263–272. doi: 10.3354/meps260263
Elsdon, T. S., and Gillanders, B. M. (2004). Fish otolith chemistry influenced by exposure to multiple environmental variables. J. Exp. Mar. Biol. Ecol. 313, 269–284. doi: 10.1016/j.jembe.2004.08.010
Elsdon, T. S., and Gillanders, B. M. (2005a). Alternative life-history patterns of estuarine fish: barium in otoliths elucidates freshwater residency. Can. J. Fish. Aquat. Sci. 62, 1143–1152. doi: 10.1139/f05-029
Elsdon, T. S., and Gillanders, B. M. (2005b). Consistency of patterns between laboratory experiments and field collected fish in otolith chemistry: an example and applications for salinity reconstructions. Mar. Freshw. Res. 56:609. doi: 10.1071/MF04146
Elsdon, T. S., and Gillanders, B. M. (2005c). Strontium incorporation into calcified structures: separating the effects of ambient water concentration and exposure time. Mar. Ecol. Prog. Ser. 285, 233–243. doi: 10.3354/meps285233
Elsdon, T. S., Wells, B. K., Campana, S. E., Gillanders, B. M., Jones, C. M., Limburg, K. E., et al. (2008). Otolith chemistry to describe movements and life-history parameters of fishes: hypotheses, assumptions, limitations and inferences. Oceanogr. Mar. Biol. Annu. Rev. 46, 297–330.
Freitas, V., Kooijman, S., and van der Veer, H. W. (2012). Latitudinal trends in habitat quality of shallow-water flatfish nurseries. Mar. Ecol. Prog. Ser. 471, 203–214. doi: 10.3354/meps10025
Gallahar, N. K., and Kingsford, M. J. (1996). Factors influencing Sr/Ca ratios in otoliths of Girella elevata: an experimental investigation. J. Fish Biol. 48, 174–186. doi: 10.1111/j.1095-8649.1996.tb01111.x
Gillanders, B. M., Able, K., Brown, J. A., Eggleston, D., and Sheridan, P. (2003). Evidence of connectivity between juvenile and adult habitats for mobile marine fauna: an important component of nurseries. Mar. Ecol. Prog. Ser. 247, 281–295.
Grammer, G. L., Morrongiello, J. R., Izzo, C., Hawthorne, P. J., Middleton, J. F., and Gillanders, B. M. (2017). Coupling biogeochemical tracers with fish growth reveals physiological and environmental controls on otolith chemistry. Ecolo. Monogr. 87, 487–507. doi: 10.1002/ecm.1264
Izzo, C., Reis-Santos, P., and Gillanders, B. M. (2018). Otolith chemistry does not just reflect environmental conditions: a meta-analytic evaluation. Fish Fish. 1:291. doi: 10.1016/j.gca.2012.07.003
Le Pape, O., Holley, J., Guerault, D., and Desaunay, Y. (2003). Quality of coastal and estuarine essential fish habitats: estimations based on the size of juvenile common sole (Solea solea L.). Estuar. Coast. Shelf Sci. 58, 793–803. doi: 10.1016/S0272-7714(03)00185-9
Limburg, K. E., Walther, B. D., Lu, Z., Jackman, G., Mohan, J., Walther, Y., et al. (2015). In search of the dead zone: use of otoliths for tracking fish exposure to hypoxia. J. Mar. Syst. 141, 167–178. doi: 10.1016/j.jmarsys.2014.02.014
Macdonald, J. I., and Crook, D. A. (2010). Variability in Sr:Ca and Ba:Ca ratios in water and fish otoliths across an estuarine salinity gradient. Mar. Ecol. Prog. Ser. 413, 147–161. doi: 10.3354/meps08703
Martin, G. B., and Thorrold, S. R. (2005). Temperature and salinity effects on magnesium, manganese, and barium incorporation in otoliths of larval and early juvenile spot Leiostomus xanthurus. Mar. Ecol. Prog. Ser. 293, 223–232. doi: 10.3354/meps293223
Martin, G. B., Thorrold, S. R., and Jones, C. M. (2004). Temperature and salinity effects on strontium incorporation in otoliths of larval spot (Leiostomus xanthurus). Can. J. Fish. Aquat. Sci. 61, 34–42. doi: 10.1139/f03-143
Martin, G. B., and Wuenschel, M. J. (2006). Effect of temperature and salinity on otolith element incorporation in juvenile gray snapper Lutjanus griseus. Mar. Ecol. Prog. Ser. 324, 229–239. doi: 10.3354/meps324229
Martinho, F., Dolbeth, M., Viegas, I., Baptista, J., Cabral, H. N., and Pardal, M. A. (2010). Does the flatfish community of the Mondego estuary (Portugal) reflect environmental changes? J. Appl. Ichthyol. 26, 843–852. doi: 10.1111/j.1439-0426.2010.01486.x
Mazloumi, N., Doubleday, Z. A., and Gillanders, B. M. (2017). The effects of temperature and salinity on otolith chemistry of King George whiting. Fish. Res. 196, 66–74. doi: 10.1016/j.fishres.2017.08.010
Miller, J. A. (2009). The effects of temperature and water concentration on the otolith incorporation of barium and manganese in black rockfish Sebastes melanops. J. Fish Biol. 75, 39–60. doi: 10.1111/j.1095-8649.2009.02262.x
Miller, J. A. (2011). Effects of water temperature and barium concentration on otolith composition along a salinity gradient: implications for migratory reconstructions. J. Exp. Mar. Biol. Ecol. 405, 42–52. doi: 10.1016/j.jembe.2011.05.017
Mohan, J., Rahman, M., Thomas, P., and Walther, B. (2014). Influence of constant and periodic experimental hypoxic stress on Atlantic croaker otolith chemistry. Aquat. Biol. 20, 1–11. doi: 10.3354/ab00542
Morse, J. W., and Bender, M. L. (1990). Partition coefficients in calcite: examination of factors influencing the validity of experimental results and their application to natural systems. Chem. Geol. 82, 265–277. doi: 10.1016/0009-2541(90)90085-L
Nagelkerken, I., Sheaves, M., Baker, R., and Connolly, R. M. (2013). The seascape nursery: a novel spatial approach to identify and manage nurseries for coastal marine fauna. Fish Fish. 16, 362–371. doi: 10.1111/faf.12057
Paton, C., Hellstrom, J., Paul, B., Woodhead, J., and Hergt, J. (2011). Iolite: freeware for the visualisation and processing of mass spectrometric data. J. Analyt. Atom. Spectrom. 26, 2508–2511. doi: 10.1039/c1ja10172b
Pentreath, R. J. (1976). Some further studies on the accumulation and retention of 65Zn and 54Mn by the plaice, Pleuronectes platessa L. J. Exp. Mar. Biol. Ecol. 21, 179–189. doi: 10.1016/0022-0981(76)90038-1
Phillis, C. C., Ostrach, D. J., Ingram, B. L., and Weber, P. K. (2011). Evaluating otolith Sr/Ca as a tool for reconstructing estuarine habitat use. Can. J. Fish. Aquat. Sci. 68, 360–373. doi: 10.1139/F10-152
Pihl, L., Cattrijsse, A., Codling, I., Mathieson, S., McLusky, D. S., and Roberts, C. M. (2002). “Habitat use by fishes in estuaries and other brackish areas,” in Fishes in Estuaries, eds M. Elliott and K. L. Hemingway (Hoboken, NJ: Blackwell Publishing Ltd), 10–53.
Primo, A. L., Azeiteiro, U. M., Marques, S. C., Martinho, F., Baptista, J., and Pardal, M. A. (2013). Colonization and nursery habitat use patterns of larval and juvenile flatfish species in a small temperate estuary. Cell 76, 126–134. doi: 10.1016/j.seares.2012.08.002
Reis-Santos, P., Gillanders, B. M., Tanner, S. E., Vasconcelos, R. P., Elsdon, T. S., and Cabral, H. N. (2012). Temporal variability in estuarine fish otolith elemental fingerprints: implications for connectivity assessments. Estuar. Coast. Shelf Sci. 112, 216–224. doi: 10.1016/j.ecss.2012.07.027
Reis-Santos, P., Tanner, S. E., Elsdon, T. S., Cabral, H. N., and Gillanders, B. M. (2013). Effects of temperature, salinity and water composition on otolith elemental incorporation of Dicentrarchus labrax. J. Exp. Mar. Biol. Ecol. 446, 245–252. doi: 10.1016/j.jembe.2013.05.027
Reis-Santos, P., Vasconcelos, R. P., Ruano, M., Latkoczy, C., Gnther, D., Costa, M. J., et al. (2008). Interspecific variations of otolith chemistry in estuarine fish nurseries. J. Fish Biol. 72, 2595–2614. doi: 10.1111/j.1095-8649.2008.01871.x
Reis-Santos, P., Vasconcelos, R. P., Tanner, S. E., Fonseca, V. F., Cabral, H. N., and Gillanders, B. M. (2018). Extrinsic and intrinsic factors shape the ability of using otolith chemistry to characterize estuarine environmental histories. Mar. Environ. Res. 140, 332–341. doi: 10.1016/j.marenvres.2018.06.002
Schmidt, S., Diallo, I. I., Derriennic, H., Fallou, H., and Lepage, M. (2019). Exploring the susceptibility of turbid estuaries to hypoxia as a prerequisite to designing a pertinent monitoring strategy of dissolved oxygen. Front. Mar. Sci. 6:613. doi: 10.3389/fmars.2019.00352
Sheaves, M., Baker, R., and Johnston, R. (2006). Marine nurseries and effective juvenile habitats: an alternative view. Mar. Ecol. Prog. Ser. 318, 303–306. doi: 10.3354/meps318303
Smith, W. E., and Kwak, T. J. (2014). Otolith microchemistry of tropical diadromous fishes: spatial and migratory dynamics. J. Fish Biol. 84, 913–928. doi: 10.1111/jfb.12317
Stanley, R. R. E., Bradbury, I. R., DiBacco, C., Snelgrove, P. V. R., Thorrold, S. R., and Killen, S. S. (2015). Environmentally mediated trends in otolith composition of juvenile Atlantic cod (Gadus morhua). ICES J. Mar. Sci. 72, 2350–2363. doi: 10.1093/icesjms/fsv070
Sturrock, A. M., Hunter, E., Milton, J. A., Johnson, R. C., Waring, C. P., and Trueman, C. N. (2015). Quantifying physiological influences on otolith microchemistry. Methods Ecol. Evol. 6, 806–816. doi: 10.1111/2041-210X.12381
Sturrock, A. M., Trueman, C. N., Milton, J. A., Waring, C. P., Cooper, M. J., and Hunter, E. (2014). Physiological influences can outweigh environmental signals in otolith microchemistry research. Mar. Ecol. Prog. Ser. 500, 245–264. doi: 10.3354/meps10699
Swearer, S., Forrester, G., Steele, M., Brooks, A., and Lea, D. (2003). Spatio-temporal and interspecific variation in otolith trace-elemental fingerprints in a temperate estuarine fish assemblage. Estuar. Coast. Shelf Sci. 56, 1111–1123. doi: 10.1016/S0272-7714(02)00317-7
Tanner, S. E., Reis-Santos, P., Vasconcelos, R. P., Fonseca, V. F., Franca, S., Cabral, H. N., et al. (2013a). Does otolith geochemistry record ambient environmental conditions in a temperate tidal estuary? J. Exp. Mar. Biol. Ecol. 441, 7–15. doi: 10.1016/j.jembe.2013.01.009
Tanner, S. E., Reis-Santos, P., Vasconcelos, R. P., Thorrold, S. R., and Cabral, H. N. (2013b). Population connectivity of Solea solea and Solea senegalensis over time. J. Sea Res. 76, 82–88. doi: 10.1016/j.seares.2012.11.005
Tanner, S. E., Vasconcelos, R. P., Cabral, H. N., and Thorrold, S. R. (2012). Testing an otolith geochemistry approach to determine population structure and movements of European hake in the northeast Atlantic Ocean and Mediterranean Sea. Fish. Res. 12, 198–205. doi: 10.1016/j.fishres.2012.02.013
Thomas, O. R. B., Swearer, S. E., Kapp, E. A., Peng, P., Tonkin Hill, G. Q., Papenfuss, A., et al. (2018). The inner ear proteome of fish. FEBS J. 286, 66–81. doi: 10.1111/febs.14715
Thorrold, S. R., Latkoczy, C., Swart, P. K., and Jones, C. M. (2001). Natal homing in a marine fish metapopulation. Science 291, 297–299. doi: 10.1126/science.291.5502.297
Turner, D. R., Whitfield, M., and Dickson, A. G. (1981). The equilibrium speciation of dissolved components in freshwater and sea water at 25°C and 1 atm pressure. Geochim. Cosmochim. Acta 45, 855–881. doi: 10.1016/0016-7037(81)90115-0
Vasconcelos, R. P., Reis-Santos, P., Costa, M. J., and Cabral, H. N. (2011). Connectivity between estuaries and marine environment: integrating metrics to assess estuarine nursery function. Ecol. Indic. 11, 1123–1133. doi: 10.1016/j.ecolind.2010.12.012
Vasconcelos, R. P., Reis-Santos, P., Maia, A., Fonseca, V. F., Franca, S., Wouters, N., et al. (2010). Nursery use patterns of commercially important marine fish species in estuarine systems along the Portuguese coast. Estuar. Coast. Shelf Sci. 86, 613–624. doi: 10.1016/j.ecss.2009.11.029
Vinagre, C., Maia, A., Reis-Santos, P., Costa, M. J., and Cabral, H. N. (2009). Small-scale distribution of Solea solea and Solea senegalensis juveniles in the Tagus estuary (Portugal). Estuar. Coast. Shelf Sci. 81, 296–300. doi: 10.1016/j.ecss.2008.11.008
Walther, B. D. (2019). The art of otolith chemistry: interpreting patterns by integrating perspectives. Mar. Freshw. Res. 70:1643. doi: 10.1071/MF18270
Walther, B. D., and Limburg, K. E. (2012). The use of otolith chemistry to characterize diadromous migrations. J. Fish Biol. 81, 796–825. doi: 10.1111/j.1095-8649.2012.03371.x
Walther, B. D., and Nims, M. K. (2014). Spatiotemporal variation of trace elements and sable isotopes in subtropical estuaries: I. Freshwater endmembers and mixing curves. Estuar. Coast. Shelf Sci. 38, 754–768. doi: 10.1007/s12237-014-9881-7
Williams, J., Jenkins, G. P., Hindell, J. S., and Swearer, S. E. (2018). Fine-scale variability in elemental composition of estuarine water and otoliths: developing environmental markers for determining larval fish dispersal histories within estuaries. Limnol. Oceanogr. 63, 262–277. doi: 10.1002/lno.10627
Woodcock, S. H., Munro, A. R., Crook, D. A., and Gillanders, B. M. (2012). Incorporation of magnesium into fish otoliths: determining contribution from water and diet. Geochim. Cosmochim. Acta 94, 12–21. doi: 10.1016/j.gca.2012.07.003
Keywords: connectivity, flatfish, nursery areas, estuaries, migrations, otoliths
Citation: Martinho F, Pina B, Nunes M, Vasconcelos RP, Fonseca VF, Crespo D, Primo AL, Vaz A, Pardal MA, Gillanders BM, Tanner SE and Reis-Santos P (2020) Water and Otolith Chemistry: Implications for Discerning Estuarine Nursery Habitat Use of a Juvenile Flatfish. Front. Mar. Sci. 7:347. doi: 10.3389/fmars.2020.00347
Received: 31 January 2020; Accepted: 24 April 2020;
Published: 26 May 2020.
Edited by:
Esteban Avigliano, Consejo Nacional de Investigaciones Científicas y Técnicas (CONICET), ArgentinaReviewed by:
Pia Schuchert, Agri-Food and Biosciences Institute (AFBI), United KingdomCopyright © 2020 Martinho, Pina, Nunes, Vasconcelos, Fonseca, Crespo, Primo, Vaz, Pardal, Gillanders, Tanner and Reis-Santos. This is an open-access article distributed under the terms of the Creative Commons Attribution License (CC BY). The use, distribution or reproduction in other forums is permitted, provided the original author(s) and the copyright owner(s) are credited and that the original publication in this journal is cited, in accordance with accepted academic practice. No use, distribution or reproduction is permitted which does not comply with these terms.
*Correspondence: Filipe Martinho, Zm1kbUBjaS51Yy5wdA==; Zm1hcnRpbmhvQGdtYWlsLmNvbQ==
†These authors have contributed equally to this work
‡These authors share senior authorship
Disclaimer: All claims expressed in this article are solely those of the authors and do not necessarily represent those of their affiliated organizations, or those of the publisher, the editors and the reviewers. Any product that may be evaluated in this article or claim that may be made by its manufacturer is not guaranteed or endorsed by the publisher.
Research integrity at Frontiers
Learn more about the work of our research integrity team to safeguard the quality of each article we publish.