- 1Department of Biomedicine, Aarhus Research Center for Innate Immunology, Aarhus University, Aarhus, Denmark
- 2Fox Chase Cancer Center, Philadelphia, PA, United States
Herpes simplex virus-2 (HSV-2) is a leading cause of sexually transmitted infections for which no effective vaccines or prophylactic treatment currently exist. Nuclear factor erythroid 2-related factor 2 (Nrf2) is a transcription factor involved in the detoxification of reactive oxygen species (ROS) and has been more recently shown to regulate inflammatory and antiviral responses. Here, we evaluated the importance of Nrf2 in the control of HSV-2 genital infection, and its role in the regulation of HSV-induced innate antiviral immunity. Comparison of antiviral gene expression profile by RNA-sequencing analysis of wild type and Nrf2-mutant (Nrf2AY/AY) murine macrophages showed an upregulation at the basal level of the type I interferon-associated gene network. The same basal increased antiviral profile was also observed in the spleen of Nrf2−/− mice. Interestingly, the lack of Nrf2 in murine cells was sufficient to increase the responsiveness to HSV-derived dsDNA and protect cells from HSV-2 infection in vitro. Surprisingly, there was no indication of an alteration in STING expression in murine cells as previously reported in cells of human origin. Additionally, genetic activation of Nrf2 in Keap1−/− mouse embryonic fibroblasts increased HSV-2 infectivity and replication. Finally, using an in vivo vaginal herpes infection model, we showed that Nrf2 controlled early innate immune responses to HSV-2 without affecting STING expression levels. Nrf2−/− mice exhibited reduced viral replication that was associated with higher level of type I interferons in vaginal washes. Nrf2−/− mice also displayed reduced weight loss, lower disease scores, and higher survival rates than wild type animals. Collectively, these data identify Nrf2 as a negative regulator of the interferon-driven antiviral response to HSV-2 without impairing STING mRNA and protein expression levels in murine cells.
Introduction
The Herpesviridae is a large and diverse family of enveloped, double-stranded DNA viruses with relatively complex genomes. Eight of the these herpes viruses are known human pathogens; in particular Herpes Simplex type-1 and−2 (HSV-1 and HSV-2) are leading causes of human viral disease affecting two-thirds of the world's population (1–3). Both of these viruses cause life-long infections due to their ability to establish latency in their hosts with the potential of reactivation. HSV-1 is most often associated with orolabial infection, whereas HSV-2 is generally affiliated with genital ulcers. In rare cases, HSV can cause infection of the central nervous system (CNS) and this can result in herpes simplex encephalitis (HSE) (4–6). Increased acquirement and transmission of human immunodeficiency virus (HIV) is observed in individuals infected with HSV-2 and additionally HSV infections are a major cause of morbidity and occasional mortality in immunocompromised patients (4–6). As no vaccine or prophylaxis exists for HSV-1/2, these agents remain significant public health concerns.
The immune system has evolved different types of early host defense mechanisms to limit viral infection. Toll-Like Receptors (TLRs), primarily present on the cell surface or in endosomal compartments, are involved in the early detection of HSV-derived glycoproteins or nucleic acids in the extracellular milieu (7). These receptors subsequently induce downstream signaling cascades, resulting in potent antiviral responses (7). Critical to sensing HSV in the cytoplasm of infected cells is the cyclic GMP-AMP synthase (cGAS). Upon nucleotide detection, cGAS produces the second messenger, 2′3′-cyclic GMP-AMP (2′3′-cGAMP), which binds to and induces conformational changes in the endoplasmic reticulum adaptor protein stimulator of interferon genes (STING). STING then dimerizes and traffics to the ER-Golgi intermediate compartment (ERGIC), where it recruits the TANK-binding kinase 1 (TBK1), which in turn phosphorylates IFN-regulatory factor 3 (IRF3). IRF3 then translocates to the nucleus as an active dimer that binds specific DNA regions, initiating the transcription of type I IFNs, and other antiviral genes (8, 9). Type I IFNs are secreted cytokines which work in auto- and paracrine manners via the IFNα receptor (IFNAR) to upregulate several hundred IFN-stimulated genes (ISGs) that target specific steps in the viral life cycle and inhibit replication. Concurrently, HSV has also evolved multiple strategies to suppress and evade host innate immune responses and facilitate viral infection (10–12). Several HSV gene products are known to counteract the cGAS/STING-mediated DNA sensing pathway. To outline a few, the Virion Host Shutoff (VHS) protein UL41 inhibits cGAS-STING signaling by degrading cGAS via its RNAse activity (13). Additionally, ICP0 and ICP27 can affect the stability and function of STING and inhibit host IRF3 nuclear signaling, thereby preventing type 1 IFN antiviral responses (14, 15).
Nuclear factor-erythroid-2 related factor 2 (Nrf2) is a bZIP transcription factor critical for the production of antioxidant and detoxifying proteins and maintenance of redox homeostasis, particularly in the contexts of stress and infection (16). At steady state, Nrf2 is kept inactive in the cytosol by binding to its repressor Kelch-Like ECH associated protein 1 (Keap1), which licenses it to proteasomal degradation by ubiquitination (17). In response to oxidative stress or electrophilic chemicals, Keap1 is inactivated and Nrf2 is released to freely translocate to the nucleus where it induces the transcription of Nrf2-responsive genes (17). Additionally, Nrf2 was described as an important regulator of the inflammatory response (18) and functions as a transcriptional repressor of inflammatory genes in murine macrophages (19). Moreover, Nrf2 was identified as a target of the immunosuppressive metabolite itaconate (20). In line with these observations, our recent work demonstrated that Nrf2 represses antiviral cytosolic sensing and the generation of type I IFN responses by suppressing the expression of the adaptor protein STING in human cells (21). This inhibition of STING by Nrf2 was sufficient to increase HSV-1 and HSV-2 infectivity in human cells (21).
A wide range of viruses including DENV, Marburg virus, CMV, and HCV have been reported to initiate Nrf2 transcriptional activity either as a result of increased oxidative stress conditions or more directly through the targeting of Nrf2 repressor Keap1 by viral proteins (22–28). Conversely, RSV infection induces a progressive reduction in Nrf2 levels, resulting in a decreased expression of antioxidant gene expression (29, 30). The reasons why some viruses activate Nrf2 while some others repress its antioxidant capacity has yet to be investigated. In the context of HSV-1 infection, murine herpes encephalitis triggered a robust accumulation of ROS in the brain of infected animals, associated with an increased expression of Nrf2-driven antioxidant enzymes, such as HO-1 and Gpx1. Intraperitoneal treatment of the infected animals with the chemical Nrf2 inducer sulforaphane was shown to reduce neurotoxicity and brain inflammation during infection without altering viral replication (31). No studies have yet investigated the role of the Nrf2/Keap1 signaling axis in the control of HSV-2-induced antiviral response and its involvement in the regulation of genital herpes outcome in mice.
In the present work, we demonstrate that Nrf2 suppresses HSV-derived dsDNA-induced antiviral immune responses and increases the susceptibility to HSV-2 genital infection in mice without altering STING expression. Using RNAseq analysis, we show a profound dysregulation of the antiviral gene expression profile between Nrf2AY/AY mutant macrophages, compared to their wild type counterparts. Interestingly, Nrf2 impairs the response to viral-derived dsDNA. Finally, using an experimental murine herpes genital infection model, we demonstrate that Nrf2 deficient animals have an overall better survival rate compared to the WT animals. Nrf2−/− mice exhibit reduced viral replication that is associated with higher level of type I IFNs in vaginal washes. Altogether, these findings demonstrate that the Nrf2 transcription factor limits the type I IFN response and increases the susceptibility to HSV-2 vaginal infection in mice.
Materials and Methods
Animals
Specific pathogen-free C57BL/6 (WT) mice were bred at Janvier Labs, France and B6.129X1-Nfe212tm1Ywk/J mice (Jackson) were bred at the animal facility, Department of Biomedicine, Aarhus University, Denmark. Animal experimentation was carried out at the animal facility, University of Aarhus. All mice used in this study were age-matched (7–9 week old) female mice on a C57BL/6 background. All described animal experiments have been reviewed and approved by Danish government authorities and comply with Danish laws.
Primary Cells, Cell Lines, and Culture Conditions
Murine bone marrow-derived macrophages (BMMs) were prepared from bone marrow isolated from femurs and tibiae of C57BL/6 mice. The cells were cultured at a density of 5 × 106 cells/plate, and differentiated over a period of 6 days using RPMI 1640 (Lonza) supplemented with 20–40% L929 cell supernatant (M-CSF producing murine fibroblast cell line), 10% heat-inactivated FCS (Sigma-Aldrich), 10 units/ml penicillin, 10 μg/ml Streptomycin, and 2 mM L-Glutamine (gibco).
Murine peritoneal macrophages were collected by washing peritoneal cavities of mice twice in HBSS. Cells were spun down afterwards and cultured in RPMI containing 10% heat-inactivated FCS (Sigma-Aldrich), 10 units/ml penicillin, 10 μg/ml Streptomycin, and 2 mM L-Glutamine (gibco). WT and Keap1 KO mouse embryonic fibroblasts (MEFs) were a kind gift of Antonio Cuadrado (Madrid, Spain), and were cultured in DMEM (Lonza) supplemented with 10% heat inactivated fetal calf serum, 200 IU.mL−1 penicillin, 100 μg.mL−1 streptomycin, and 600 μg.mL−1 glutamine.
dsDNA and cGAMP Stimulation of Cells
HSV-60 naked, a viral dsDNA motif and 2′3′-cGAMP, a STING ligand, were both obtained from Invivogen. Intracellular delivery of dsDNA and cGAMP was achieved using Lipofectamine 2000 (Invitrogen) diluted in serum-free medium with a ratio of Lipo.dsDNA/cGAMP of 1:1. Final concentration for both dsDNA and cGAMP was 4 μg.mL−1.
HSV Production, Quantification, and Infection
HSV-2 333 strain and HSV-2 MS strain were kindly provided by Søren R. Paludan (Aarhus University, Aarhus, Denmark). All HSVs were propagated in Vero cells, purified by ultra-centrifugation, and titrated by standard plaque assay as previously described (32). MEFs, BMMs, or peritoneal macrophages were infected with HSV at the indicated multiplicity of infection (MOI) in a small volume of serum-free medium for 1 h at 37°C. Prior to analysis, cells were incubated with DMEM complete medium containing antibiotics for an additional day of culture.
Western Blot and Semi-native WB Dimerization Assay
Western blotting was performed as previously reported (21). Antibodies and dilutions used are also mentioned in Olagnier et al. (21). Anti-ICP5 HSV antibody was obtained from (Abcam) (used at 1:3000).
qPCR Analysis
Gene expression was determined by real-time quantitative PCR, using TaqMan detection systems (Applied Biosciences). For in vitro experiments, RNA was extracted using the High Pure RNA Isolation kit (Roche) and RNA quality was assessed by Nanodrop spectrometry (Thermo Fisher). Total RNA from isolated organs (spleen and vagina) was extracted by use of the Qiagen RNase Plus Mini Kit (Qiagen) according to the manufacturer's guidelines and RNA quality assessed by Nanodrop spectrometry (Thermo Fisher). mRNA levels for murine Ifnb1 (Mm00439552_s1), Cxcl10 (Mm00445235_m1), Tmem173 (Mm01158117_m1), Ifit2 (Mm00492606_m1), Nfe2l2 (Nrf2) (Mm00477784_m1), and β-Actin (Mm02619580_g1) were analyzed using premade TaqMan assays (Thermo Fisher) and the RNA-to-Ct-1-Step kit according to the manufacturer's recommendations (Applied Biosciences).
RNAseq Analysis
RNA seq data set was obtained from a previously published manuscript and already available dataset from Otsuki et al. (33). The sequencing reads were aligned to Mm10 genome and the resulting binary alignment (BAM) files were used to calculate the gene counts that represent total number of sequencing reads aligned to a gene. To identify differentially expressed genes between WT vs. Nrf2AY/AY samples, DESeq2 algorithm was used (34). The list of differentially expressed genes from DESeq2 output were selected based on 10% adjusted P-value level and an FDR of 0.1. Among these, the highly significant genes (FDR <10%) were selected that are involved in pattern recognition signaling, antiviral signaling, and experimentally identified Nrf2 transcriptional targets (21). To depict these genes as a heatmap, count data was transformed using regularized-logarithm transformation (rld) (34), and the resulting values were mean-centered and plotted using pheatmap package available in bioconductor repository [Raivo Kolde (2015). pheatmap: Pretty Heatmaps. R package version1.0.8. http://CRAN.R-project.org/package=pheatmap]. Gene ontology and KEGG pathway enrichment analysis was done using DAVID bioinformatics resources portal (35). Genes involved in antiviral and interferon signaling differentially expressed genes between WT vs. Nrf2AY/AY samples were plotted as functional networks using Ingenuity Pathway Analysis software (QIAGEN Inc., https://www.qiagenbioinformatics.com/products/ingenuity-pathway-analysis).
Human orthologs were depicted after mapping mouse genes using IPA.
In vivo Vaginal HSV-2 Infection
All mice were pretreated by s.c injection of 2 mg of Depo-Provera. Five days later mice were anesthetized with Isoflourane (Sigma-Aldrich) and inoculated intra vaginally with 20 μL HSV-2 (333 strain) (3,33 × 105 PFU) diluted in PBS. To facilitate infection the mice were maintained under anesthesia and placed on their back for 10 min. Vaginal fluids were collected at the indicated time points post infection (p.i.) by pipetting 2 × 40 μL of PBS. The washes were diluted to a finale volume of 250 μL. In selected experiments mice were euthanized by cervical dislocation (c.d.) at different time points and vaginas and spleens were isolated and used for RNA extraction (Qiagen). The virus infected mice were monitored and examined daily and the severity of disease was scored using the following criterias: 0 = healthy, 1 = genital erythema, 2 = moderate genital inflammation, 3 = purulent genital lesion and/or generally bad conditions, 4 = neurological bad conditions and/or severe bad conditions. Mice were sacrificed by c.d. when reaching score 4. For survival studies, Log rank (Mantel-Cox) tests were performed on Kaplan-Meier survival graphs using Prism 7 (GraphPad). Ethical permission was obtained from the Danish Veterinary and Food Administration to perform the vaginal infections. All efforts were made to minimize suffering, and mice were monitored daily during infection.
Virus Plaque Assay
Virus titer in the collected vaginal fluids were determined on monolayers of Vero cells as previously described (36). Briefly, Vero cells were seeded in DMEM supplemented with 5% FCS at a density of 1.2 × 106 cells/petri dish and left overnight to settle. The next day the cells were infected with diluted samples of the collected vaginal fluids and incubated for 1 h. Subsequently 5 mL of DMEM supplemented with 0.2 % human Immunoglobulin were added and the cells were further incubated for 2–3 days. The cells were stained with 0.03% methylene blue and the plaque were counted.
Cytokine Measurements
MCP-1 in the vaginal fluids was measured by use of ELISA (R&D development system) according to the manufacturer's instructions. Type 1 IFNs bioactivity was measured by use of an IFN-α/β bioassay based on L929 cells as previously reported (36). UV-light inactivated vaginal fluid samples and IFN-α/β standard was transferred to 96-well plates in successive 2-fold dilutions. Subsequently L929 cells were added to each well and the plates were incubated overnight. The next day Vesicular Stomatis Virus was added to the relevant wells and the plates were incubated for a further 2–3 days. Read out was determined by the dilution mediating 50% protection and defined as 1 Unit of IFN-α/β/mL.
Statistical Analysis
Values were expressed as the mean ± SEM. Graphs and statistics were computed using Graph Pad Prism 7. An unpaired, two-tailed Student's t-test was used to determine significance of the difference between the control and each experimental condition. P-values of <0.05 were considered statistically significant, ***, p < 0.001; **, p < 0.01, and *, p < 0.05.
Results
Nrf2 Dampens Basal Antiviral Gene Levels in Murine Cells
To assess the involvement of the transcription factor Nrf2 in the regulation of antiviral gene expression, we analyzed a published RNA-sequencing dataset from Otsuki et al. (33), comparing the transcriptomes of Nrf2-mutated AY/AY peritoneal macrophages lacking functional Nrf2 to wild-type (WT) cells. The absolute gene counts were processed and analyzed using DESeq2, which estimates robust expression differences across conditions by considering discreteness, large dynamic range, and the presence of outliers. Since log-transformation of absolute gene counts could result in heteroskedasticity, we used regularized log-transformation of the counts by shrinking the values for genes with higher counts across samples. This step stabilizes the variances across samples. The rlog transformed values for 249 differentially expressed genes are depicted as heatmap. In heatmap, all of the 249 genes that are differentially expressed (Wald-test p < 0.001, Benjamini-Hochberg False Discovery Rate <0.06) are shown (Figure 1A). From this analysis, we noticed that pathways related to the innate antiviral immune response, including “Response to Virus, Innate immune Responses, or Cellular Responses to IFN-gamma,” were significantly enriched between WT and Nrf2AY/AY macrophages (Figure 1A). Amongst the differentially expressed genes were several involved in type I IFN signaling and immune responses, including the transcription factors Irf7 and Stat2 and the IFN-driven gene Oas2 (Figure 1B). In sharp contrast, RNA transcripts of known Nrf2-inducible genes, such as Nqo1, Gsr, and Gdm, were all downregulated in the absence of functional Nrf2 (Figure 1B). To map the interactions across these different genes, a functional clustering node analysis identified a significant intersection between Nrf2 signaling and antiviral-related pathways (Figure 1C). Amongst the most highly induced genes interacting with the Nrf2 antioxidant network were some key transcription factors involved in the early response to viruses including the transcription factors Irf1 and Irf7. Altogether, these data suggest an important link between Nrf2 and the regulation of basal antiviral genes in murine macrophages.
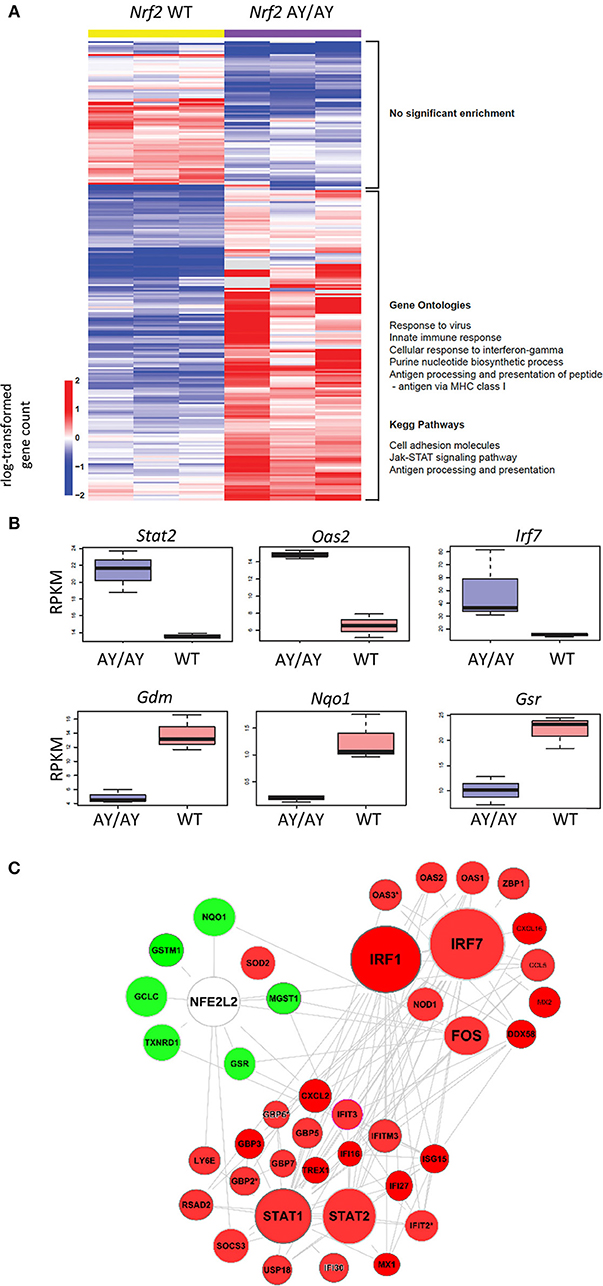
Figure 1. Nrf2 alters the basal level of ISGs in murine macrophages. Comparison of antiviral gene expression profile by RNA-sequencing (RNAseq) analysis of wild-type (WT) and Nrf2-mutated (AY/AY) bone marrow-derived macrophages (BMMs). Differentially expressed pathways and genes that satisfied p-value <0.001 and fold change cutoff >1 or < -1 were selected. The data are from one experiment performed in triplicate. (A) rlog-transformed gene counts and a listing of representative gene ontologies and KEGG pathways associated with these genes is represented in a heatmap. (B) Representation of RNA-reads for different interferon-driven and Nrf2-driven genes from RNAseq. Graph displays boxplots with boxes indicating mean, SEM as well as min and max values. (C) Cloud analysis of differentially expressed genes from Nrf2 AY/AY BMMs related to both Nrf2 and IFN-associated pathways is represented. The experiment is a re-analysis of the work of Otsuki et al. (33) (https://www.ncbi.nlm.nih.gov/pubmed/26677805).
Nrf2 Impairs Antiviral Gene Expression in Splenic Tissues
As Nrf2 repressed antiviral gene expression in murine macrophages, we further asked whether the lack of Nrf2 was also affecting basal antiviral gene levels in vivo. To test this hypothesis, spleen tissues from WT and Nrf2−/− mice were harvested and RNA isolated for qPCR analysis. The high sensitivity of qPCR gives precise measurements of a sample despite low mRNA level, especially in ex vivo studies. Notably, both Ifit2 (Isg54) and Ifnb1 mRNA levels were significantly heightened in absence of Nrf2 (Figures 2A,B). Surprisingly, STING gene level (Tmem173), a critical antiviral adaptor molecule, was unaltered in murine BMMs and in the spleen of Nrf2−/− animals compared to WT (Figure 2C and Supplementary Figure 1A), as well as in Keap1−/− mouse embryonic fibroblasts (MEFs) (Supplementary Figure 1B). Together, these data argue that Nrf2 may function as a negative regulator of basal antiviral gene expression in murine cells and tissues without altering STING expression.
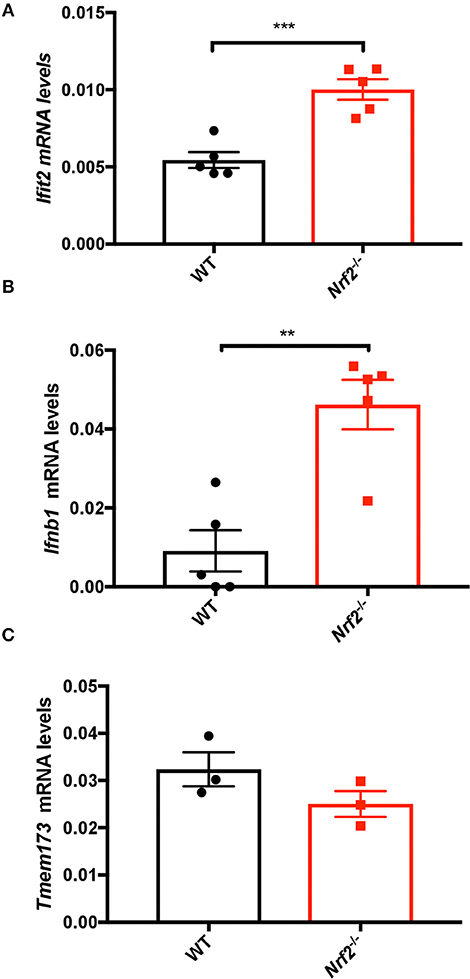
Figure 2. Nrf2 suppresses basal type I IFN and ISG levels in splenic tissues. Spleens from WT and Nrf2 knockout (KO) mice were harvested and RNA isolated. The basal levels of Ifit2 (Isg54) (A), Ifnβ1 (B), and Tmem173 (C) were quantified by qPCR, and normalized to Actin. The data are the mean ± SEM where each data point represents one animal.
Nrf2 Suppresses Innate Antiviral Responses Upon HSV-Derived DNA and cGAMP Stimulation
As Nrf2 repressed antiviral basal gene expression level, we further asked whether Nrf2 was also capable of affecting the responsiveness of cells to activation of the cGAS-STING pathway. WT and Nrf2 deficient BMMs were lipofected for 6 h with either HSV-derived double stranded DNA (HSV-DNA) or the STING ligand, cGAMP, and antiviral gene expression level was assessed by qPCR. The lack of Nrf2 in BMMs resulted in an increased responsiveness to HSV-DNA, as shown by the enhanced expression of Ifnb1, Cxcl10, and Ifit2 mRNA levels in cells deficient for Nrf2 compared to the WT (Figures 3A–C).
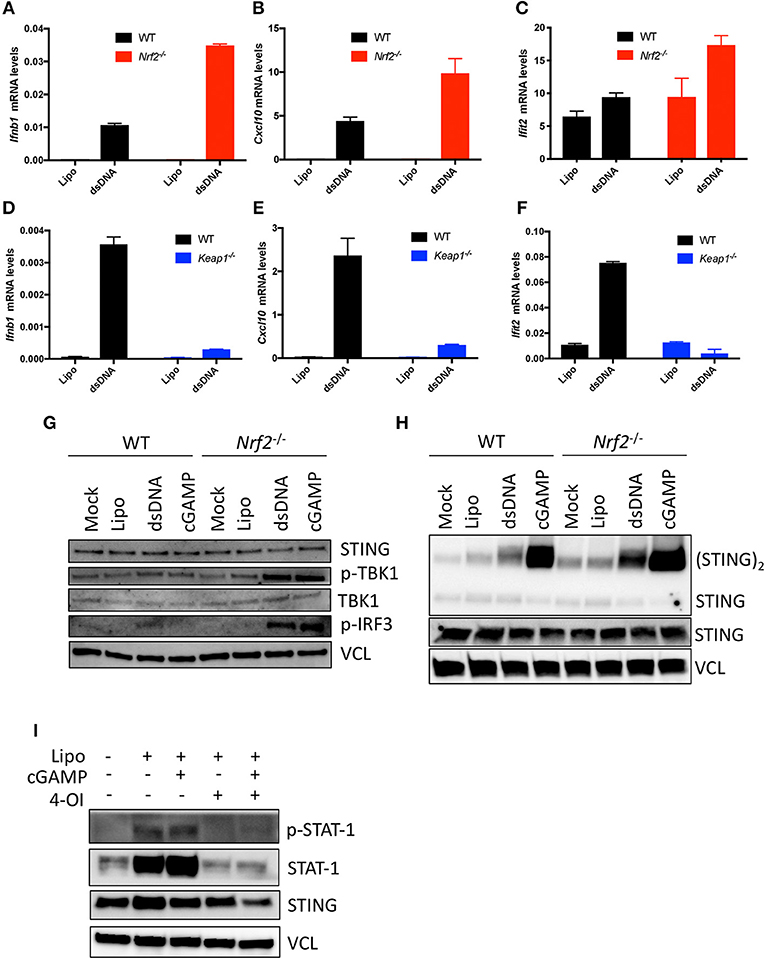
Figure 3. Nrf2 impairs antiviral innate immunity following HSV-DNA or cGAMP stimulation. (A–C) WT and Nrf2 KO BMMs and WT and (D–F) Keap1 KO MEFs were transfected for 6 h with Lipofectamine 2000 (Lipo.) alone or in combination with HSV-derived dsDNA (dsDNA) (4 μg.mL−1). RNA was isolated and analyzed for the levels of Ifnβ1, Cxcl10, and Ifit2 mRNA by qPCR. Data are the means ± SEM where each panel is representative of one experiment performed in duplicate or triplicate. (G–H) WT or Nrf2 KO BMMs were transfected for 3 h with Lipofectamine 2000 alone or in combination with dsDNA or cGAMP (4 μg.mL−1) and early antiviral signaling events or STING dimerization were determined by immunoblotting. (I) WT MEFs were pre-treated with 4-Octyl-itaconate (4-OI) (125 μM) for ~18 h and then subsequently transfected with Lipofectamine 2000 alone or in combination with cGAMP (4 μg.mL−1). Late antiviral signaling events were determined using immunoblotting where vinculin (VCL) is the loading control. The experiments was performed at least twice with similar results.
Conversely, the induction of Ifnb1 and the IFN-regulated genes Cxcl10 or Ifit2 was significantly reduced in Nrf2 active Keap1 deficient MEFs following HSV-DNA challenge (Figures 3D–F). To determine the step at which Nrf2 affected the responsiveness of the cGAS-STING pathway in murine cells, Nrf2−/− BMMs were stimulated with either HSV-DNA or cGAMP and assessed by immunoblotting for early innate immune signaling events. In response to both DNA and cGAMP, deficiency of Nrf2 affected downstream STING signaling events, as shown by the increased phosphorylation of some of the components in the cGAS-STING pathway TBK1 and IRF3 (Figure 3G). We previously reported Nrf2 to be a suppressor of STING expression in human cells. This did not, however, seem to be the case with murine cells, as no change in STING protein levels could be detected between WT and Nrf2−/− BMMs (Figure 3G). We then assessed whether Nrf2 deficiency affected the efficiency of cGAS and STING-dependent events in response to stimulation with dsDNA and cGAMP. Interestingly, STING dimerization was increased in Nrf2 lacking BMMs in response to HSV-DNA and cGAMP compared to WT BMMs (Figure 3H). Finally, we demonstrated that the strength of this Nrf2-dependent antiviral inhibitory response was sufficient to also inhibit downstream STAT1 signaling. Indeed, pre-treatment of WT MEFs with the newly described Nrf2 inducer 4-octyl-itaconate (4-OI) (21) drastically impaired phosphorylation of STAT1 transcription factor in response to cGAMP (Figure 3I).
Lack of Nrf2 Elevates Type I IFN Response and Decreases Herpes Genital Infection in Mice
To further investigate the role of Nrf2 in the control of HSV-2-induced type I IFN and HSV-2 replication, mRNA levels of Ifnβ1 were monitored by qPCR following virus infection in vitro (HSV-2, strain MS, titer 1 × 108 PFU/mL). Peritoneal macrophages lacking Nrf2 responded more vigorously to HSV-2, as denoted by the ~3-fold increase in Ifnβ1 gene expression (Figure 4A). Modulation of HSV-2-induced type I IFN response by Nrf2 was sufficient to repress the expression of HSV-2 ICP5 protein expression in WT MEFs. ICP5 is a major capsid protein of the herpes virus produced in the cytoplasm and translocated to the nucleus upon viral assembly. Conversely, the absence of Keap1 led to an increased accumulation of the viral protein in MEFs (Figure 4B). Finally, we established that genetic activation of Nrf2 through Keap1 deletion promoted HSV-2 replication in vitro, as determined by the increased number of replicating virus by plaque assay (Figure 4C), thus implicating Nrf2 in increasing the susceptibility to HSV-2 in vitro.
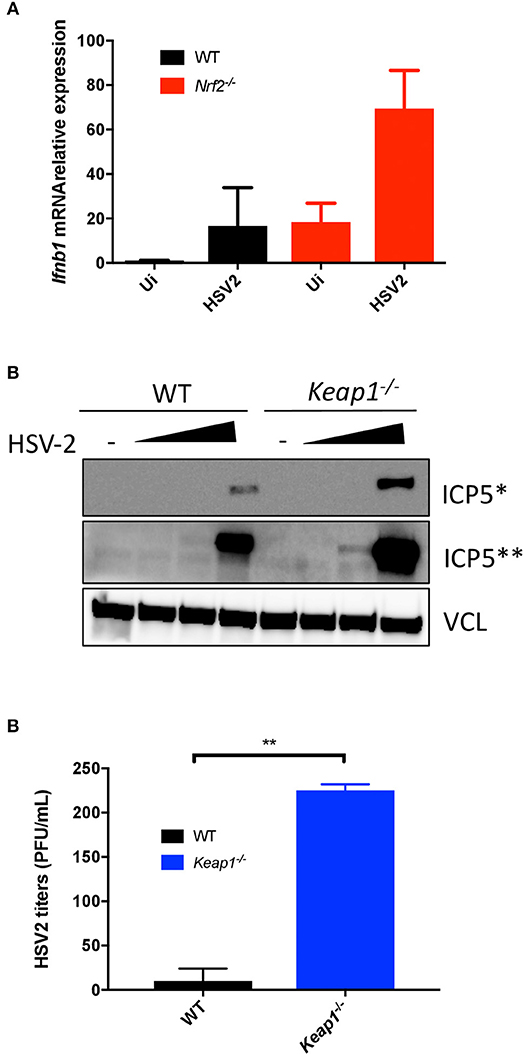
Figure 4. Nrf2 increases the susceptibility to HSV-2 infection in vitro. (A) WT and Nrf2 KO peritoneal macrophages were infected with HSV-2, MS strain for 6 h and mRNA level of Ifnβ1 was detected by qPCR. (B) WT and Keap1 KO MEFs were infected with HSV-2, MS strain (MOI 0.01, 0.1 and 1, titer 1 × 108 PFU/mL) for ~24 h. ICP5 Viral protein expression was detected by immunoblotting and vinculin (VCL) was used as a loading control. (C) The supernatants from samples in (B) were used for plaque assay to determine viral replication. The plaque assay was performed on duplicated samples at an MOI of 1.
To test whether the effect of Nrf2 on the antiviral response is sufficient to affect susceptibility to HSV-2 in vivo, we used a herpes genital infection model in mice lacking Nrf2 (infected intravaginally with HSV-2, strain 333, 3.33 × 105 PFU/mouse). Overall, Nrf2−/− mice had higher survival rates and reduced weight loss, as well as displayed lower disease scores than their WT counterparts throughout the course of the experiment (Figures 5A–E). Strikingly, the absence of Nrf2 significantly prevented the weight loss observed in WT animals (Figure 5C) and reduced the symptoms of infection after 6 days post-inoculation with the virus (Figure 5E). Additionally, Nrf2−/− mice exhibited reduced vaginal viral replication, as determined by plaque assay, that was associated with higher level of type I IFNs in vaginal washes at day 1 post infection (Figures 5F,G). No such increase in chemokine monocyte chemoattractant protein-1 (MCP-1) release, a key regulator in the migration and filtration of monocytes and macrophages, could be observed in the vaginal washes from the same infected animals (Supplementary Figure 2). Furthermore, the increase in type I IFN observed in Nrf2−/− animals could not be explained by an enhanced basal level of Ifnb1, as seen in the spleen (Figure 2), as no such correlation could be made in the vagina of uninfected WT and Nrf2−/− mice (Supplementary Figure 3).
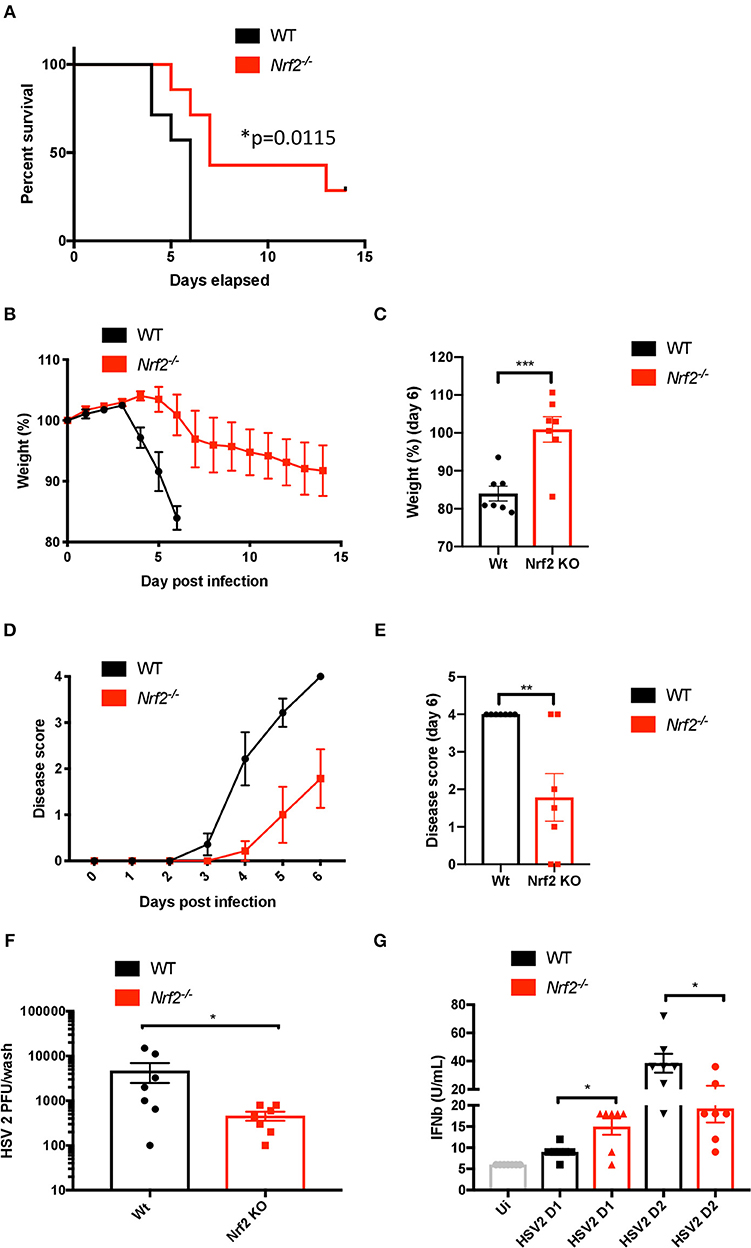
Figure 5. Lack of Nrf2 protects mice from HSV-2 vaginal infection and delays physiopathology. WT and Nrf2 KO mice were infected intravaginally with HSV-2, strain 333 (3.33 × 105 PFU/mouse). (A) Survival, (B,C) weight loss and (D,E) disease evolution score were measured over time (n = 7 mice). (F) After 1 day of infection, vaginal washes were collected for use in plaque assay to estimate HSV-2 titers (n = 7). (G) Type I IFN bioactivity in the vaginal washes was assessed by in vitro bioassay (n = 7).
Discussion
Recent work has shown that Nrf2 is an important regulator of inflammatory responses (18). Moreover, we have previously reported that Nrf2 suppresses the antiviral type I IFN response in human cells (21). However, the link between Nrf2 and the regulation of early innate immune response to virus infections in vivo has remained unknown. In the present study, we demonstrated and concluded (i) based on the data of Otsuki et al., that Nrf2AY/AY mutant macrophages displayed a profound dysregulation of the antiviral gene expression profile compared to WT murine cells, (ii) that Nrf2 impaired the response to herpes virus-derived dsDNA without affecting STING expression in murine cells, (iii) that the genetic activation of Nrf2 increased HSV-2 replication in vitro, and (iv) that Nrf2−/− mice are less susceptible to vaginal infection with HSV-2 due to heightened type I IFN response.
Type I IFNs are known as potent antiviral molecules, and are essential in promoting the expression of ISGs and restricting viral replication (37, 38). Our recent work in human cells demonstrated that Nrf2 restricted type I IFN responses by altering the mRNA stability of the adaptor molecule STING (21).
In the current report, we present data on murine cells, both in vitro and in vivo, suggesting that Nrf2 also impairs the host antiviral immune response, however, without affecting STING expression. Higher expression of type I IFN and other ISGs were detected in murine cells lacking a functional Nrf2, but no difference in STING level was noticed in murine macrophages (Figure 1). The same observation was made in vivo, where the basal levels of ISGs were enhanced in spleens from Nrf2−/− animals, but the expression of STING was unaltered (Figure 2). Interestingly, although type I IFN responses are affected by Nrf2 in the mice experiments, the effect of Nrf2 on STING expression seemed to be restricted to human cells. There was no alteration of STING mRNA or protein observed in Nrf2 lacking murine cells. Altogether, this collection of data suggests that Nrf2 uses an alternative way of controlling the antiviral response in murine cells compared with human cells.
Our work demonstrated a dysregulation in the basal levels of type I IFNs and other antiviral associated genes in murine cells mutated or lacking Nrf2. Interestingly, the responsiveness to STING ligands, including cGAMP or HSV-derived dsDNA, was also magnified in absence of Nrf2. Surprisingly, dimerization of STING was enhanced in absence of Nrf2 following cGAMP or dsDNA stimulation, despite no basal change in STING protein level (Figure 3). This observation suggests that Nrf2 could function in controlling STING dimer formation or stabilization. HSV-1 was previously shown to elevate oxidative stress in the cells and trigger S-glutathionylation of TRAF3 and 6 which potentiated PRR signaling (39). As Nrf2 is a master regulator involved in detoxification processes and redox homeostasis in the cell, it is tempting to speculate that either S-gluthationylation or oxidation of STING protein could increase the dimer formation or its stability in absence of Nrf2, and hence potentiates downstream signaling events. Another parameter that could also influence the overall magnified innate immune response to STING ligands observed in absence of Nrf2 is the production of the second messenger cyclic GMP-AMP (cGAMP). Indeed, Cyclic guanosine monophosphate (GMP)–adenosine monophosphate (AMP) synthase (cGAS) is a DNA sensor that triggers innate immune responses through production of cGAMP, which binds and activates the adaptor protein STING (40). Thus, a modulation of cGAS activity by Nrf2 could also lead to an enhanced engagement of STING dimer formation. These different hypotheses will further be tested to uncover the precise mechanistic machinery beyond the effect of Nrf2 on the cGAS-STING pathway in murine cells.
The importance of Nrf2 as a regulator of infection has been previously reported for different viruses (41). For instance, Nrf2-mediated signaling was shown to protect mice against Respiratory syncytial virus (RSV) infection (42). In line with these findings, it was also shown that Nrf2 had a protective role upon Influenza A virus (IAV) and dengue virus (DENV) infection (43, 44). With the DNA virus HSV-2, however, our data suggest that the presence of Nrf2 facilitates HSV-2 replication through the dampening of the antiviral response (Figure 4). Mice lacking Nrf2 exhibited reduced viral replication that was associated with higher level of type I IFNs in vaginal washes. Overall, Nrf2−/− mice displayed reduced weight loss, lower disease scores, and higher survival rates than WT animals (Figure 5). Thus, depending on the pathogen and the route of infection, Nrf2 can play either a protective or exacerbating role during host responses to virus infections. Knowledge gained from an in vivo model cannot always be translated to humans, and the same is also valid when using murine cells in vitro (45, 46). One of the best examples related to STING remains the cleavage of the antiviral adaptor protein by the NS2B3 protease of DENV in human cells, which does not happen in murine or non-human primate cells (47, 48). The use of the herpes genital infection model in mice is based on the investigation of a primary infection with HSV-2. Here, mice are easy to handle, have low costs, and it is a well-described model when it comes to studying herpes genital infection in vivo (36). However, this animal model has limitations, including the impossibility of studying recurrent genital infections. In contrast, the guinea pig model provides an excellent tool for studying both primary and recurrent genital herpes infections (49). Additionally, humans share other unique traits with guinea pigs, especially at the immune system level (50). Although findings from our in vivo study give a tendency on the possible effect Nrf2 plays in human herpes infection, it is impossible to speculate or predict anything on the outcome Nrf2 might have in a real human vaginal infectious context. For these reasons, expansion of some of our data to a guinea pig model is needed to properly understand the role Nrf2 plays in the course of HSV infection.
In conclusion, our study proposes a model in which Nrf2 transcription factor regulates the early antiviral response to HSV-2. We also unveil the key role of Nrf2 in the physiopathology of HSV-2 vaginal infection, which suggests a possible new therapeutic signaling route to intervene on herpes vaginal infections.
Data Availability
Sequencing data were previously uploaded dataset to GEO with the following accession number (GSE75176).
Ethics Statement
The animal study was reviewed and approved by the Danish Veterinary and Food Administration.
Author Contributions
DO and CH conceived the project, designed the experiments, and supervised the project. CG performed most of the in vitro experiments. MI performed all the in vivo experiments. SP and SB performed the RNAseq analysis. DO and CG wrote the manuscript. DO assembled the figures. All authors edited and agreed on the submitted version of the manuscript. AT assisted CG in the in vitro experiments.
Funding
This research work was supported by Riisfortfonden, Agnes and Poul Friis Fond, Dagmar Marshalls Fond, Magda Sofie og Aase Lütz's mindelegat Fond, Tømrermester Jørgen Holm og hustru Elisa F. Hansens indelegat Fond, Fabrikant Einar Willumsens mindelegat Fond, Læge Sofus Carl Emil Friis og Hustru Olga Doris Friis Legat to DO and by Hørslevsfonden, Agnes and Poul Friis Fond, Brdr. Hartmanns fond, Oda og Hans Svenningsens Fond, Augustinus Fonden and Hede Nielsens Fond, to CH. Salaries were supported by a Carlsbergfonden International Research Fellowship and an Associate Professor fellowship from the Johan and Hanne Weimann Fond to DO, a Lundbeck Ph.D. fellowship to CG and a Lundbeck postdoctoral fellowship to MI.
Conflict of Interest Statement
The authors declare that the research was conducted in the absence of any commercial or financial relationships that could be construed as a potential conflict of interest.
Supplementary Material
The Supplementary Material for this article can be found online at: https://www.frontiersin.org/articles/10.3389/fimmu.2019.02101/full#supplementary-material
References
1. Melchjorsen J, Matikainen S, Paludan SR. Activation and evasion of innate antiviral immunity by herpes simplex virus. Viruses. (2009) 1:737–59. doi: 10.3390/v1030737
2. Suazo PA, Ibanez FJ, Retamal-Diaz AR, Paz-Fiblas MV, Bueno SM, Kalergis AM, et al. Evasion of early antiviral responses by herpes simplex viruses. Mediat Inflamm. (2015) 2015:593757. doi: 10.1155/2015/593757
3. Olagnier D, Lababidi RR, Hadj SB, Sze A, Liu Y, Naidu SD, et al. Activation of Nrf2 signaling augments vesicular stomatitis virus oncolysis via autophagy-driven suppression of antiviral immunity. Mol Ther. (2017) 25:1900–16. doi: 10.1016/j.ymthe.2017.04.022
4. Paludan SR, Bowie AG, Horan KA, Fitzgerald KA. Recognition of herpesviruses by the innate immune system. Nat Rev Immunol. (2011) 11:143–54. doi: 10.1038/nri2937
6. Whitley RJ, Roizman B. Herpes simplex virus infections. Lancet. (2001) 357:1513–8. doi: 10.1016/S0140-6736(00)04638-9
7. Ma Y, He B. Recognition of herpes simplex viruses: toll-like receptors and beyond. J Mol Biol. (2014) 426:1133–47. doi: 10.1016/j.jmb.2013.11.012
8. Ishikawa H, Barber GN. The STING pathway and regulation of innate immune signaling in response to DNA pathogens. Cell Mol Life Sci. (2011) 68:1157–65. doi: 10.1007/s00018-010-0605-2
9. Holm CK, Rahbek SH, Gad HH, Bak RO, Jakobsen MR, Jiang Z, et al. Influenza A virus targets a cGAS-independent STING pathway that controls enveloped RNA viruses. Nat Commun. (2016) 7:10680. doi: 10.1038/ncomms10680
10. Su C, Zhan G, Zheng C. Evasion of host antiviral innate immunity by HSV-1, an update. Virol J. (2016) 13:38. doi: 10.1186/s12985-016-0495-5
11. Zhang J, Zhao J, Xu S, Li J, He S, Zeng Y, et al. Species-specific deamidation of cGAS by herpes simplex virus UL37 protein facilitates viral replication. Cell Host Microbe. (2018) 24:234–48 e5. doi: 10.1016/j.chom.2018.07.004
12. Mogensen TH. Pathogen recognition and inflammatory signaling in innate immune defenses. Clin Microbiol Rev. (2009) 22:240–73. doi: 10.1128/CMR.00046-08
13. Su C, Zheng C. Herpes simplex virus 1 abrogates the cGAS/STING-mediated cytosolic DNA-sensing pathway via its virion host shutoff protein, UL41. J Virol. (2017) 91:e02414–16. doi: 10.1128/JVI.02414-16
14. Christensen MH, Jensen SB, Miettinen JJ, Luecke S, Prabakaran T, Reinert LS, et al. HSV-1 ICP27 targets the TBK1-activated STING signalsome to inhibit virus-induced type I IFN expression. EMBO J. (2016) 35:1385–99. doi: 10.15252/embj.201593458
15. Kalamvoki M, Roizman B. HSV-1 degrades, stabilizes, requires, or is stung by STING depending on ICP0, the US3 protein kinase, and cell derivation. Proc Natl Acad Sci USA. (2014) 111:E611–7. doi: 10.1073/pnas.1323414111
16. Soares MP, Ribeiro AM. Nrf2 as a master regulator of tissue damage control and disease tolerance to infection. Biochem Soc Trans. (2015) 43:663–8. doi: 10.1042/BST20150054
17. Hayes JD, Dinkova-Kostova AT. The Nrf2 regulatory network provides an interface between redox and intermediary metabolism. Trends Biochem Sci. (2014) 39:199–218. doi: 10.1016/j.tibs.2014.02.002
18. Thimmulappa RK, Lee H, Rangasamy T, Reddy SP, Yamamoto M, Kensler TW, et al. Nrf2 is a critical regulator of the innate immune response and survival during experimental sepsis. J Clin Invest. (2006) 116:984–95. doi: 10.1172/JCI25790
19. Kobayashi EH, Suzuki T, Funayama R, Nagashima T, Hayashi M, Sekine H, et al. Nrf2 suppresses macrophage inflammatory response by blocking proinflammatory cytokine transcription. Nat Commun. (2016) 7:11624. doi: 10.1038/ncomms11624
20. Mills EL, Ryan DG, Prag HA, Dikovskaya D, Menon D, Zaslona Z, et al. Itaconate is an anti-inflammatory metabolite that activates Nrf2 via alkylation of KEAP1. Nature. (2018) 556:113–7. doi: 10.1038/nature25986
21. Olagnier D, Brandtoft AM, Gunderstofte C, Villadsen NL, Krapp C, Thielke AL, et al. Nrf2 negatively regulates STING indicating a link between antiviral sensing and metabolic reprogramming. Nat Commun. (2018) 9:3506. doi: 10.1038/s41467-018-05861-7
22. Olagnier D, Peri S, Steel C, van Montfoort N, Chiang C, Beljanski V, et al. Cellular oxidative stress response controls the antiviral and apoptotic programs in dengue virus-infected dendritic cells. PLoS Pathog. (2014) 10:e1004566. doi: 10.1371/journal.ppat.1004566
23. Cheng YL, Lin YS, Chen CL, Tsai TT, Tsai CC, Wu YW, et al. Activation of Nrf2 by the dengue virus causes an increase in CLEC5A, which enhances TNF-alpha production by mononuclear phagocytes. Sci Rep. (2016) 6:32000. doi: 10.1038/srep32000
24. Edwards MR, Johnson B, Mire CE, Xu W, Shabman RS, Speller LN, et al. The Marburg virus VP24 protein interacts with Keap1 to activate the cytoprotective antioxidant response pathway. Cell Rep. (2014) 6:1017–25. doi: 10.1016/j.celrep.2014.01.043
25. Page A, Volchkova VA, Reid SP, Mateo M, Bagnaud-Baule A, Nemirov K, et al. Marburgvirus hijacks nrf2-dependent pathway by targeting nrf2-negative regulator keap1. Cell Rep. (2014) 6:1026–36. doi: 10.1016/j.celrep.2014.02.027
26. Lee J, Koh K, Kim YE, Ahn JH, Kim S. Upregulation of Nrf2 expression by human cytomegalovirus infection protects host cells from oxidative stress. J Gen Virol. (2013) 94 (Pt 7):1658–68. doi: 10.1099/vir.0.052142-0
27. Smirnova OA, Ivanova ON, Mukhtarov FS, Tunitskaya VL, Jansons J, Isaguliants MG, et al. Analysis of the domains of hepatitis C virus core and NS5A proteins that activate the Nrf2/ARE cascade. Acta Naturae. (2016) 8:123–7. doi: 10.32607/20758251-2016-8-3-123-127
28. Ivanov AV, Smirnova OA, Ivanova ON, Masalova OV, Kochetkov SN, Isaguliants MG. Hepatitis C virus proteins activate NRF2/ARE pathway by distinct ROS-dependent and independent mechanisms in HUH7 cells. PLoS ONE. (2011) 6:e24957. doi: 10.1371/journal.pone.0024957
29. Komaravelli N, Ansar M, Garofalo RP, Casola A. Respiratory syncytial virus induces NRF2 degradation through a promyelocytic leukemia protein - ring finger protein 4 dependent pathway. Free Radic Biol Med. (2017) 113:494–504. doi: 10.1016/j.freeradbiomed.2017.10.380
30. Komaravelli N, Tian B, Ivanciuc T, Mautemps N, Brasier AR, Garofalo RP, et al. Respiratory syncytial virus infection down-regulates antioxidant enzyme expression by triggering deacetylation-proteasomal degradation of Nrf2. Free Radic Biol Med. (2015) 88 (Pt B):391–403. doi: 10.1016/j.freeradbiomed.2015.05.043
31. Schachtele SJ, Hu S, Lokensgard JR. Modulation of experimental herpes encephalitis-associated neurotoxicity through sulforaphane treatment. PLoS ONE. (2012) 7:e36216. doi: 10.1371/journal.pone.0036216
32. Reinert LS, Harder L, Holm CK, Iversen MB, Horan KA, Dagnaes-Hansen F, et al. TLR3 deficiency renders astrocytes permissive to herpes simplex virus infection and facilitates establishment of CNS infection in mice. J Clin Invest. (2012) 122:1368–76. doi: 10.1172/JCI60893
33. Otsuki A, Suzuki M, Katsuoka F, Tsuchida K, Suda H, Morita M, et al. Unique cistrome defined as CsMBE is strictly required for Nrf2-sMaf heterodimer function in cytoprotection. Free Radic Biol Med. (2016) 91:45–57. doi: 10.1016/j.freeradbiomed.2015.12.005
34. Love MI, Huber W, Anders S. Moderated estimation of fold change and dispersion for RNA-seq data with DESeq2. Genome Biol. (2014) 15:550. doi: 10.1186/s13059-014-0550-8
35. Huang DW, Sherman BT, Tan Q, Collins JR, Alvord WG, Roayaei J, et al. The DAVID gene functional classification tool: a novel biological module-centric algorithm to functionally analyze large gene lists. Genome Biol. (2007) 8:R183. doi: 10.1186/gb-2007-8-9-r183
36. Iversen MB, Reinert LS, Thomsen MK, Bagdonaite I, Nandakumar R, Cheshenko N, et al. An innate antiviral pathway acting before interferons at epithelial surfaces. Nat Immunol. (2016) 17:150–8. doi: 10.1038/ni.3319
37. Teijaro JR. Type I interferons in viral control and immune regulation. Curr Opin Virol. (2016) 16:31–40. doi: 10.1016/j.coviro.2016.01.001
38. Gerlach N, Schimmer S, Weiss S, Kalinke U, Dittmer U. Effects of type I interferons on Friend retrovirus infection. J Virol. (2006) 80:3438–44. doi: 10.1128/JVI.80.7.3438-3444.2006
39. Gonzalez-Dosal R, Horan KA, Rahbek SH, Ichijo H, Chen ZJ, Mieyal JJ, et al. HSV infection induces production of ROS, which potentiate signaling from pattern recognition receptors: role for S-glutathionylation of TRAF3 and 6. PLoS Pathog. (2011) 7:e1002250. doi: 10.1371/journal.ppat.1002250
40. Motwani M, Pesiridis S, Fitzgerald KA. DNA sensing by the cGAS-STING pathway in health and disease. Nat Rev Genet. (2019). doi: 10.1038/s41576-019-0151-1. [Epub ahead of print].
41. Ramezani A, Nahad MP, Faghihloo E. The role of Nrf2 transcription factor in viral infection. J Cell Biochem. (2018) 119:6366–82. doi: 10.1002/jcb.26897
42. Cho HY, Imani F, Miller-DeGraff L, Walters D, Melendi GA, Yamamoto M, et al. Antiviral activity of Nrf2 in a murine model of respiratory syncytial virus disease. Am J Respir Crit Care Med. (2009) 179:138–50. doi: 10.1164/rccm.200804-535OC
43. Kosmider B, Messier EM, Janssen WJ, Nahreini P, Wang J, Hartshorn KL, et al. Nrf2 protects human alveolar epithelial cells against injury induced by influenza A virus. Respir Res. (2012) 13:43. doi: 10.1186/1465-9921-13-43
44. Yageta Y, Ishii Y, Morishima Y, Masuko H, Ano S, Yamadori T, et al. Role of Nrf2 in host defense against influenza virus in cigarette smoke-exposed mice. J Virol. (2011) 85:4679–90. doi: 10.1128/JVI.02456-10
45. Shih AY, Damm-Ganamet KL, Mirzadegan T. Dynamic structural differences between human and mouse STING lead to differing sensitivity to DMXAA. Biophys J. (2018) 114:32–9. doi: 10.1016/j.bpj.2017.10.027
46. Rongvaux A, Willinger T, Martinek J, Strowig T, Gearty SV, Teichmann LL, et al. Development and function of human innate immune cells in a humanized mouse model. Nat Biotechnol. (2014) 32:364–72. doi: 10.1038/nbt.2858
47. Stabell AC, Meyerson NR, Gullberg RC, Gilchrist AR, Webb KJ, Old WM, et al. Dengue viruses cleave STING in humans but not in nonhuman primates, their presumed natural reservoir. Elife. (2018) 7:e31919. doi: 10.7554/eLife.31919
48. Aguirre S, Maestre AM, Pagni S, Patel JR, Savage T, Gutman D, et al. DENV inhibits type I IFN production in infected cells by cleaving human STING. PLoS Pathog. (2012) 8:e1002934. doi: 10.1371/journal.ppat.1002934
49. Stanberry LR, Kern ER, Richards JT, Overall JC Jr. Recurrent genital herpes simplex virus infection in guinea pigs. Intervirology. (1985) 24:226–31. doi: 10.1159/000149647
Keywords: Nrf2, antiviral immunity, innate immune responses, type I IFN, herpes virus, HSV, STING, genital infection
Citation: Gunderstofte C, Iversen MB, Peri S, Thielke A, Balachandran S, Holm CK and Olagnier D (2019) Nrf2 Negatively Regulates Type I Interferon Responses and Increases Susceptibility to Herpes Genital Infection in Mice. Front. Immunol. 10:2101. doi: 10.3389/fimmu.2019.02101
Received: 12 June 2019; Accepted: 20 August 2019;
Published: 06 September 2019.
Edited by:
Lbachir Benmohamed, University of California, Irvine, United StatesReviewed by:
Jia Zhu, University of Washington, United StatesAziz Alami Chentoufi, Centre Hospitalier Universitaire Mohammed VI, Morocco
Copyright © 2019 Gunderstofte, Iversen, Peri, Thielke, Balachandran, Holm and Olagnier. This is an open-access article distributed under the terms of the Creative Commons Attribution License (CC BY). The use, distribution or reproduction in other forums is permitted, provided the original author(s) and the copyright owner(s) are credited and that the original publication in this journal is cited, in accordance with accepted academic practice. No use, distribution or reproduction is permitted which does not comply with these terms.
*Correspondence: Christian Kanstrup Holm, aG9sbUBiaW9tZWQuYXUuZGs=; David Olagnier, b2xhZ25pZXJAYmlvbWVkLmF1LmRr
†These authors have contributed equally to this work
‡Share senior authorship