- 1Institute for Glycomics, Griffith University, Southport, QLD, Australia
- 2School of Chemistry and Molecular Biosciences, The University of Queensland, Brisbane, QLD, Australia
- 3School of Pharmacy, The University of Queensland, Brisbane, QLD, Australia
- 4Institute for Molecular Bioscience, The University of Queensland, Brisbane, QLD, Australia
In the development of vaccines, the ability to initiate both innate and subsequent adaptive immune responses need to be considered. Live attenuated vaccines achieve this naturally, while inactivated and sub-unit vaccines generally require additional help provided through delivery systems and/or adjuvants. Liposomes present an attractive adjuvant/delivery system for antigens. Here, we review the key aspects of immunity against Plasmodium parasites, liposome design considerations and their current application in the development of a malaria vaccine.
Introduction
Malaria vaccine development has been a focus of research since the 1940s when inoculation with homologous inactivated sporozoites and/or serum resulted in control of parasitemia amongst immunized domestic fowls (1, 2). Follow-up studies also showed that monkeys and ducks were protected against Plasmodium (P) knowlesi and P. lophurae following vaccination with killed, adjuvanted parasites (3, 4). Additionally, in 1967, it was demonstrated for the first time, that immunization with irradiated sporozoites protected mice against P. berghei (5). Despite these early promising findings, an effective vaccine for malaria still eludes scientists with only one vaccine candidate, RTS, S, receiving a positive scientific opinion from European regulators and it is currently approved for use in pilot implementation trials in 3–5 epidemiologically distinct locations in sub-Saharan Africa (6, 7). The complexity of Plasmodium parasites, antigenic polymorphism and failure to maintain long-lived immune responses calls for continued efforts in the search for novel vaccines which can effectively prevent P. falciparum and P. vivax infections (8).
A major bottleneck in the development of vaccines against infectious diseases is the failure to initiate robust innate immune responses and subsequent potentiation and maintenance of downstream adaptive immune responses. This is achieved naturally with live attenuated vaccines while inactivated and sub-unit vaccines require delivery systems and/or adjuvants for efficient presentation to the immune system and additional stimulation to enhance potency (9). To address this, careful selection of adjuvants and delivery systems needs to be considered early in the vaccine development process. However, only a handful of adjuvants have been licensed or tested for use in human vaccines and these are summarized in Table 1.
First proposed by Gregoriadis and Allison in 1974 as immunological adjuvants (39), liposomes are a promising vaccine adjuvant/antigen delivery system. Historically well-known as drug carriers, liposomes are self-assembling phospholipid vesicles capable of incorporating and protecting antigens from degradation, as well as facilitating antigen delivery to professional antigen presenting cells (APCs) (9, 40–43). Liposomes generally act by depot formation resulting in enhanced uptake by APCs and subsequent induction of the desired immune responses. To date, the extensive use of liposomes can be attributed to their safety profile, biocompatibility, biodegradability, versatility, and plasticity and therefore they present an attractive platform for malaria vaccine development.
In a natural malaria infection, the acquisition of clinical immunity is slow, spanning several years of repeated exposure, and it is not sterile (44). An ideal vaccine capable of inducing sterile immunity against the different life-cycle stages of malaria will need to induce a qualitatively and/or quantitatively different immune response to that induced during natural infection immunity (45). Since stage-specific immunity to malaria requires humoral and cell-mediated immune responses, the ideal vaccine-induced responses should preferably be comprised of both forms of responses. However, for rational vaccine development, a clear understanding of the complex nature of immunity to malaria is required and this in turn may help to inform the selection of an appropriate adjuvant/delivery system. This review highlights key aspects of the immune response to malaria, design considerations of liposomes, and their current application in malaria vaccine development.
Immunity to Malaria
Plasmodium parasites, the causative agents of malaria, are obligate intracellular organisms which undergo a complex life-cycle in the vertebrate host broadly divided into: the mosquito stage which occurs in the vector; the pre-erythrocytic stage which occurs in the vertebrate host's liver; and the erythrocytic stage which occurs in the blood of the vertebrate host (46). At all life-cycle stages, the immune responses induced following infection differ significantly and a clear understanding of these responses will inform the vaccine development process.
Pre-erythrocytic Stage Immunity to Malaria
The pre-erythrocytic stage of malaria infection is clinically quiescent, probably due to the low number of sporozoites inoculated by the mosquito while taking a blood meal. During this stage, studies in mice have shown that antibodies can control infection through immobilization of sporozoites by inhibiting sporozoite motility and subsequent invasion of hepatocytes (47, 48). Following natural infection, studies demonstrated the existence of pre-erythrocytic antigen-specific antibodies to P. falciparum; however, their role remains unclear (49–51). To date, the best model that has enabled the study of pre-erythrocytic immune response mechanisms has utilized irradiated sporozoites in both humans and animals. Radiation-attenuated sporozoites retain the capacity to infect hepatocytes but cannot develop into an erythrocytic infection. Studies in rodent models involving inoculation of radiation-attenuated sporozoites demonstrated that antibodies were involved in the enhanced clearance of sporozoites, reduction in sporozoite motility and inhibition of hepatocyte invasion (52, 53). In clinical studies, induction of antibodies to the circumsporozoite protein (CSP) following immunization with the P. falciparum sporozoite (PfSPZ) vaccine has been shown to partially correlate with protection (54–58). Furthermore, following immunization with RTS, S/AS01, high CSP-specific antibody titers are induced and are a surrogate measure of protective efficacy for this vaccine candidate (16, 59–63).
Cell-mediated immunity following inoculation of radiation-attenuated sporozoites has also been shown to contribute to vaccine-induced sterilizing immunity to Plasmodium infection in both mice and humans (54–58, 64–72). Studies in rodent models, however, indicate that a network of cellular mechanisms mediates immunity to pre-erythrocytic infection (64–66, 68, 70–72). Initial studies in mice immunized with irradiated P. yoelii 17XNL sporozoites reported that cytotoxic CD8+ T cells mediated protection against a wild-type challenge of infectious sporozoites (64, 65). Further studies indicated that IL-12 from liver antigen presenting cells (APCs) stimulated CD8+ T cells and Natural killer (NK) cells to produce interferon (IFN)-γ. IFN-γ, in turn, induces the infected hepatocytes to produce nitric oxide (NO), which subsequently kills the parasite in the hepatocyte (66, 68, 71). In addition, the balance between IL-2, IL-10, IL-12, and IFN-γ results in an inflammatory response that contributes to pre-erythrocytic immunity (70, 72). In human volunteers, both CD4+ and CD8+ T cell responses against pre-erythrocytic antigens have been observed following immunization with irradiated sporozoites (54–58, 67, 69).
Controlled infection immunization (CII) studies, involving experimental sporozoite inoculation via infectious mosquito bites with concurrent chemoprophylaxis provides another model for investigating pre-erythrocytic immunity to malaria. These studies have shown that a protective polyfunctional T cell response to pre-erythrocytic antigens predominantly characterized by the production of IFN-γ, tumor necrosis factor (TNF), and IL-2 is induced following CII (73–76). Purified IgG against P. falciparum sporozoites, obtained from CII trial participants was also shown to inhibit liver stage infection in a humanized liver-chimeric mouse model (73, 75, 77).
Erythrocytic Stage Immunity to Malaria
During the erythrocytic stage, clinical signs, and symptoms of malaria manifest as the parasites invade and replicate in RBCs. Studies in animal models have demonstrated that the innate immune system is involved in the initial recognition of blood-stage parasites, promotion of inflammation, inhibition of parasite growth and potentiation of the adaptive immune response (78, 79). Plasmodium falciparum pathogen-associated molecular patterns (PAMPs) such as glycosylphosphatidylinositol (GPI) anchors (Toll-like receptor 2 [TLR2]), hemozoin (NOD-like receptor containing pyrin domain 3 [NLRP3] inflammasome), CpG-containing DNA motifs bound to hemozoin (TLR9) and AT-rich DNA motifs (unidentified cytosolic receptor) have been shown to trigger an inflammatory cascade by binding pattern recognition receptors (PRRs) on the surface of innate immune cells (79–83). This interaction of PAMPs and PRRs results in the production of pro-inflammatory cytokines (IL-12 [p70], IFN-γ and TNF) by APCs, as well as regulatory cytokines (IL-10 and TGF-β) that have been implicated in immunity and pathogenesis to blood-stage malaria infection (79, 84, 85).
Several innate immune cells such as dendritic cells (DCs), macrophages, mast cells, neutrophils, NK cells, natural killer T (NKT) cells, and γδ T cells have been implicated in this initial immune response (85–88). Plasmodium parasites have been shown to modulate DC maturation and function resulting in the induction of regulatory T cells which in turn modulates CD4+ T cell responses, suppressing protective immune responses while averting immune-mediated pathology (85). IL-12 production by DCs has also been implicated in the production of IFN-γ by NK cells and CD4+ Th1 cells resulting in the control of parasite growth (85). Understanding the balance between protective and immunopathologic responses following DC activation and maturation might have favorable implications in designing vaccines to prevent severe malaria (85). In vitro, NK cells have been shown to be an early source of IFN-γ, promoting the destruction of infected red blood cells by activated macrophages (79, 89). γδ T cells and monocytes on the other hand have been associated with elevated levels of TNF, IL- 10, IP-10, IL-6, macrophage inflammatory protein (MIP)-1β and MIP-1α which is linked with severe disease (90). Furthermore, downregulation of γδ T cell responses following repeated exposures to Plasmodia has also been implicated with better tolerance to clinical malaria (91, 92). Given their specificity for restricted TCR ligands, γδ T cells present an attractive target for a vaccine to protect against severe disease (45, 90).
Antibodies play a role in naturally acquired immunity to erythrocytic stage malaria, as it has been shown that the passive transfer of immunoglobulin from immune donors resulted in the reduction of parasitaemia and clinical disease among semi-immune recipients from East Africa as well as non-immune Thai patients (93–95). Antibodies may function by inhibiting merozoite invasion of RBCs (96), binding to pRBCs and enhancing clearance by the spleen (97, 98), as well as opsonizing pRBCs, resulting in phagocytosis by macrophages (99, 100).
Cell-mediated immunity against the erythrocytic stage is primarily mediated by CD4+ T cells, as demonstrated in both murine and human models. Studies showed that mice depleted of CD4+ T cells developed very high parasitaemia and were unable to control the infection compared to mice depleted of CD8+ T cells, which developed mild parasitaemia that subsequently resolved; this indicated a clear role of CD4+ T cells in erythrocytic stage immunity (101, 102). Additionally, adoptive transfer of CD4+ T cells was shown to confer protection and control parasitaemia in immunodeficient mice (103). Further investigation of the role of CD4+ T cells demonstrated that during the acute phase of infection, there was a significant upregulation of an IFN-γ-specific CD4+ T cell (Th1) response followed by an IL-4-specific CD4+ T cell-mediated (Th2) antibody response during the chronic phase (104). These data indicate that early activation of Th1 cells enables control of the infection via effector mechanisms such as macrophages, followed by a Th2 response which activates B cells to clear the parasite in the later stages of the infection in mice (105, 106).
In human studies, the role of CD4+ T cells was demonstrated when volunteers were infected with low doses of blood-stage P. falciparum followed by drug cure (107). In this study, volunteers appeared to be protected against a homologous challenge infection with immunity associated with an IFN-γ-specific CD4+ T cell response and nitric oxide synthase (NOS) production in the absence of detectable antibodies (107). However, a follow-up study suggested that residual drug may have contributed to the apparent protection (108). Another study showed that stimulation of T cells obtained from children living in Papua New Guinea resulted in parasite-specific IFN-γ and TNF responses, which were associated with protection against clinical episodes of malaria (109). More recently, studies in African children showed that CD4+ T cells may play an important modulatory role in the development of blood-stage immunity (110, 111). Higher IFN-γ/IL-10+ specific-CD4+ T cell responses were observed amongst children heavily exposed to malaria compared to children with low exposure indicating that CD4+ T cells may play an important immunomodulatory role in the pathogenesis of childhood malaria (110). Additionally, the induction of IL-10-producing CD4+ T cells amongst highly exposed children may interfere with the development of immunity, which may have implications for vaccine development (111).
T follicular helper cells (Tfh) are a subset of CD4+ T cells, capable of providing B cell help as well as activating follicular B-cell responses (112–114). Recent studies in mice showed that Tfh cells play a critical role in controlling P. chabaudi blood-stage infection via activation of IL-21 mediated responses (115). Therefore, since humoral responses are critical to the erythrocytic stages of Plasmodium, an in-depth understanding of the activation and maintenance of Tfh cells during malaria will be critical in designing blood-stage vaccines (115).
Regulatory T cells (Tregs) are another CD4+ T cell subset implicated in the maintenance of immune homeostasis and control of excessive pathogen-driven inflammatory responses (116, 117). Following Plasmodium infection, uncontrolled production of pro-inflammatory cytokines is associated with pathology in both mice and humans (118–121) and anti-inflammatory cytokines (TGF-β and IL-10) are known to be critical in the modulation of this inflammatory response (118, 120, 122, 123). The immunomodulatory function of IL-10 and TGF-β is associated with Tregs whose role in rodent malaria remains unclear. Some studies have shown that Tregs are critical in the control of pro-inflammatory responses associated with pathology (124, 125) while other studies have associated upregulation of Tregs with detrimental outcomes (124, 126, 127). These discrepancies may have been dependent on the rodent parasite strains utilized in the study (128). Tregs may inhibit protective immune responses resulting in enhanced parasite growth if induced early in infection but may also limit immune-mediated pathology (45, 128–130). Clinical studies have shown that acute infection with Plasmodium parasites resulted in upregulation of Tregs which positively correlated with augmented parasite load and subsequent disease severity amongst these individuals (131–134). Given this background, a vaccine capable of inducing Tregs with the ability to protect against the immunopathology associated with malaria infection would be desirable if parasite persistence is required for the maintenance of protective immune responses. However, since Tregs are known to inhibit protective immune responses, treatment with antimalaria drugs at the time of vaccination may be necessary to “normalize” the pre-existing immune response and ensure induction of the appropriate vaccine-specific responses (45).
The role of CD8+ T cells in defense against blood-stage parasites remains unclear due to the fact that mature RBCs do not express MHC class I molecules. However, in vitro studies have shown that both P. falciparum and P. vivax are able to invade erythroblasts—immature erythrocytes that possess a nucleus and express MHC class I molecules (135, 136). Additionally, studies in mice demonstrated that blood-stage parasite antigens were cross-presented by CD8-α+ DCs, inducing parasite-specific CD8+ T cell responses capable of lysing APCs (137). These findings indicated the possible relevance of CD8+ T cells in erythrocytic immunity to Plasmodium. Indeed, additional studies in mice demonstrated that parasitized erythroblasts activated CD8+ T cells in an antigen-specific manner (138). This contact-dependent Fas—Fas Ligand (FasL) interaction of CD8+ T cells with the parasitized erythroblasts results in the exposure of phosphatidylserine (PS) on the erythroblast surface (139). Cells displaying PS on their surface are rapidly phagocytosed by macrophages. Thus, CD8+ T cells in conjunction with macrophages are able to mediate immunity to a blood-stage malaria infection in mice (139). Earlier studies in a P. yoelii infection model demonstrated that professional APCs might cross-present parasite-derived peptides on MHC class I to CD8+ T cells leading to cytotoxicity through the production of IFN-γ, perforin and granzyme B (140). Furthermore, parasite-specific CD8+ T cells have also been shown to clear infected reticulocytes, which express MHC class I molecules, via the secretion of IFN-γ and expression of granzyme B (141).
These data highlighted in the studies above provide an insight into the complex nature of immune responses elicited following infection with malaria parasites. Understanding the balance between protective immunity and immunopathology is critical for the development of an ideal vaccine capable of inducing both humoral and cell-mediated immune responses against different life-cycle stages of the malaria parasite. To achieve this balance, careful selection of antigen delivery systems and adjuvants during the vaccine development process is paramount and the pliability of liposome-based platforms can be utilized for this purpose.
Design Considerations of Liposomal Vaccine Formulations
Based on their design, liposome vaccine formulations can be tailored to achieve desired immune responses and adjuvant properties by modification of vesicle physicochemical factors (summarized in Figure 1), such as lipid composition, charge, PEGylation, antigen encapsulation, and addition of immunomodulators (9, 40–43).
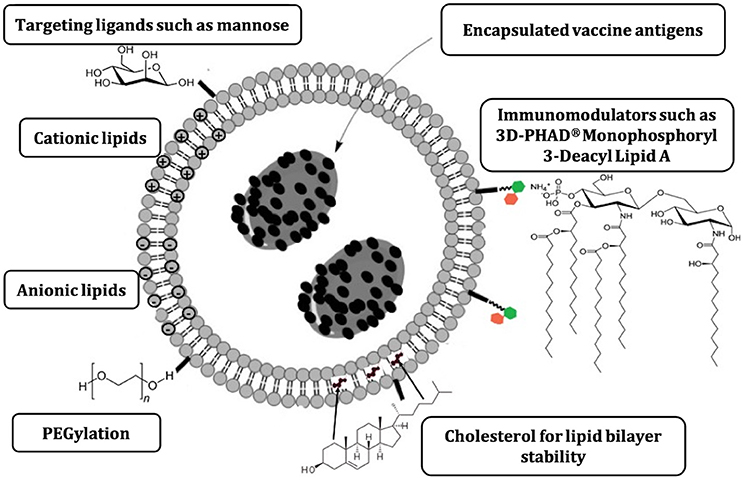
Figure 1. Major liposome physicochemical characteristics that can be modified to direct immune responses elicited following vaccination (9).
The choice of phospholipid has been shown to enhance the adjuvanticity of liposomes. Long chain lipids tend to form rigid ordered bilayer structures while those with shorter tails tend to form fluid and disordered vesicles (9). Immunization of animals with liposomes formulated with long chain lipids such as dimethyldioctadecylammonium bromide (DDAB) and distearyol derivative of L-α-phosphatidyl choline (DSPC) resulted in stronger antigen-specific antibody responses when compared to animals that received liposomes formulated with shorter chain lipids (142). Additionally, the stability of the lipid bilayer can be enhanced further with the addition of cholesterol within the liposome formulation resulting in improved antibody responses compared to formulations without cholesterol (143–145).
Positively charged (cationic) liposomes formulated with saturated acyl chain lipids (with a quaternary ammonium head group) have been shown to promote the binding of antigen at the site of injection stimulating interaction with APCs to elicit a robust Th1 cytokine response (146). In contrast, highly fluid unsaturated acyl chain liposomes that are rapidly cleared from the injection site result in lower activation of APCs as measured by the decreased expression of DC co-stimulatory molecules CD40 and CD86 (146). Additionally, cationic liposomes have been shown to promote retention of higher levels of antigen at the injection site, resulting in a depot-effect allowing continuous attraction of APCs and subsequent induction of robust cell-mediated immune responses comprised of IFN-γ, IL-2, TNF, and IL-17 (147). On the other hand, negatively charged (anionic) and neutral liposomes were rapidly cleared from the injection site resulting in lower activation of APCs and lower Th1 as well as Th17 cytokine responses (9, 147, 148).
The inclusion of polyethylene glycol (PEG), known as PEGylation, has been extensively used for the stabilization of liposomes (149). PEGylation has been shown to mask the charge, due to the hydrophilic chains of PEG extending out from the surface of the liposomes subsequently reducing the electrostatic retention of antigen to the surface of these vesicles (150, 151). Additionally, PEGylation influences lipid packaging reducing the number of bilayers and resulting in reduced vesicle size (151). This modification of liposome size and antigen adsorption properties by PEG was shown to reduce depot formation, resulting in reduced IgG2b antibody and Th1 (IFN-γ) cytokine responses as well as an elevated Th2 (IL-5) cytokine response compared to non- PEGylated liposome formulations (150, 151).
The particle size of liposomes has also been shown to impact adjuvanticity and direct the development of the resulting cell-mediated immune response (42). Studies have shown that the immune response induced following administration of small-sized liposome vesicles (10–100 nm) was skewed toward Th2 whilst larger vesicles (400–2,500 nm) induced a Th1 response characterized by augmented IFN-γ and IgG2a production (152). The differences in the profiles of the induced immune response of large vs. small vesicles could be due to differences in antigen processing and trafficking to lymph nodes. Large-sized vesicles (560 nm) were shown to be more efficiently phagocytosed and processed by macrophages compared to smaller vesicles (155 nm) (153). Additionally, trafficking of liposome particles to lymph nodes has been shown to be size dependent, with small-sized vesicles (20–200 nm) freely draining to and specifically targeting lymph node-resident cells, while large-sized vesicles (500–2,000 nm) require dendritic cells for trafficking from the injection site to lymph nodes (154). More recently, immunization using a formulation containing large-sized cationic liposomes (~500 nm) resulted in enhanced splenocyte proliferative responses and reduced IL-10 production compared to small sized liposomes (~100 nm) (155). Interestingly, smaller unilamellar liposomes (70 nm) were reported to stimulate higher IgG titers than larger unilamellar (400 nm) but not large submicron size multilamellar liposomes in mice (156). The potency of multilamellar liposomes can be explained by more efficient antigen protection against degradation in their multiple lipid bilayers.
Liposomes can be modified to incorporate additional lipophilic immunomodulators within or attached to the lipid bilayer to enhance adjuvanticity. Such immunomodulators are crucial in the activation of the cells of the innate immune system via PRRs which recognize PAMPs on the surface of pathogens, subsequently activating the adaptive immune system. The activation of innate immune cells such as dendritic cells and macrophages requires the use of Toll-like receptor (TLR) and NOD-like receptor (NLR) type PRRs to direct a robust immune response (157). Therefore, the use of synthetic PRR agonists has been predicted to be critical in the formulation of liposome-based vaccine adjuvants (9, 158).
Liposome formulations can be customized by incorporating PRR agonists that mediate activation and maturation of APCs which in turn facilitates the uptake and processing of liposome-associated antigens resulting in potent cell-mediated immune responses (9). The most widely used PRR agonist monophosphoryl lipid A (MPLA), a TLR-4 agonist, has been used in licensed vaccine formulations Fendrix (hepatitis B) (159) and Cervarix (human papillomavirus) (160). A synthetic analog of MPLA, 3′-O-desacyl-4′-monophosphoryl lipid A formulated with Quillaja saponaria Molina, fraction 21 (QS-21) saponin is included in the liposome-based GSK Adjuvant System 01 (AS01) and has been tested in human studies for the malaria vaccine RTS, S (Mosquirix) (16), as well as a shingles sub-unit vaccine HZ/su which demonstrated over 90% efficacy amongst elderly persons (15). Similarly, liposomes can be tagged with sugars such as mannose to target them to lectin-like molecules on APCs to facilitate phagocytic uptake thereby promoting MHC class II involvement and, via cross presentation, MHC class I. This targeting of liposomes to different uptake pathways may aid in directing the resulting immune response toward a mixed Th1/Th2 response (9, 161).
The route of administration of particulate antigen delivery systems such as liposomes has been shown to affect the type and magnitude of immune response induced. Interestingly liposomes can be even administered orally; however, they need to be extensively modified to improve their stability in the gastrointestinal tract and their mucosa adhesive properties (162). In a cross-sectional study in mice, the intramuscular, intradermal and intralymphatic routes of administration were associated with intermediate to high induction of IgG2a and IFN-γ cytokine production while the subcutaneous route was associated with low elicitation of IgG2a and IFN-γ cytokine production (163). These data indicate that the route of administration is critical in the generation of the desired immune response and should be considered while interpreting immunological data following immunization with liposome-based vaccine formulations. Together, it is evident that the versatility and plasticity of liposomes facilitates the tailoring of the desired immune responses, as well as enhanced adjuvanticity and this can be exploited for the development of a malaria vaccine.
Utility of Liposomes in Malaria Vaccine Development
Liposomes are increasingly becoming used in a number of malaria vaccine candidates targeting the different life-cycle stages. The use of liposomes in the development of sporozoite-stage malaria vaccines dates back to the mid-1980s where tetrapeptide antigens (asparagine-alanine-asparagine-proline) derived from the repetitive region of the circumsporozoite (CS) protein of P. falciparum sporozoites were conjugated to carrier proteins and were incorporated into liposomes. These studies demonstrated that liposomes containing carrier protein-conjugated peptide induced a potent humoral immune response which was further enhanced when lipid A was incorporated in the liposome formulation (164–169). The aforementioned studies laid the foundation for the development of RTS, S, the only vaccine against malaria that has received approval for use in pilot implementation trials in sub-Saharan Africa (7). The RTS, S vaccine construct is made of the central repeat region (amino acids 210-398) (R) and the C-terminal region containing the T-cell epitopes of CSP (T), fused to hepatitis B surface antigen (HBsAg) (S), co-expressed in Saccharomyces cerevisiae yeast and self-assembled with unfused HBsAg antigen (170, 171). These hybrid virus-like particles (VLPs) were co-formulated with GSK's proprietary liposome-based adjuvant system, AS01, which contains potent immunostimulants, MPLA and QS-21 that was selected over AS02, an oil-in-water emulsion adjuvant following evidence of enhanced antigen-specific antibody and CD4+ T-cell responses as well as improved efficacy in large-scale clinical studies (61, 172–175). The level of and mechanism of immunity induced by RTS, S in endemic settings are topics of much research. Over 4 years of follow up, the level of protection ranged from 18 to 36%, depending on the age of the child and whether the child received 3 or 4 doses of vaccine. Protection was clearly greater in the early months after vaccination, but waned rapidly after that and there was a ‘negative efficacy' during the 5th year in some children (176). This is a sub-optimal response and as such the mechanism of immunity came under great scrutiny. Evidence suggests that the level of antibody to the CS protein and serum levels of IFN-γ post vaccination both correlate with protection (177, 178). A major concern, has been the rapid diminution of antibody levels over time, in the face of parasite exposure. The problem is not that RTS,S is not immunogenic. The adjuvant system is one of the most potent there is for human use and is used elsewhere with great effect. It has made Shingrix a highly successful vaccine where even in the elderly there is 90% efficacy. Also, the problem is not with boosting per se, as each dose of RTS,S is accompanied by a rapid rise in antibody titer. In our view, the main problem is that even though RTS,S has a powerful adjuvant system, it is still not powerful enough. Titres wane after vaccination and natural infection will not boost. The main reasons for this appear to be: (i) antigenic polymorphism; and (ii) that the dose of sporozoites that individuals are exposed to (and which express the CS protein) are simply too low to boost or maintain antibody levels. T cell epitopes present on the CS protein which are incorporated into the vaccine are polymorphic (179), and this polymorphism does contribute to the low efficacy (180). The T-cell epitopes are known to be non-cross-reactive (181). An additional factor may relate to liposome design per se. Indeed, efforts are currently underway to remodel RTS, S such that each HBsAg particle expresses CSP thereby increasing the concentration of parasite antigens (182). Here, CSP-HBsAg fusion proteins were co-expressed in Pichia pastoris yeast which in the presence of a tightly regulated inducible alcohol oxidase (AOX1) promoter allows production of a higher density of hybrid VLPs (182, 183). This vaccine construct, co-formulated with Matrix-M ™, a saponin-based liposomal adjuvant is now undergoing clinical testing NCT029050191.
In the development of transmission-blocking vaccines, gel core liposomes encapsulating Pfs25, an antigen expressed on zygotes and ookinetes of P. falciparum and a leading transmission-blocking vaccine (TBV) candidate, have been tested in mouse models (184). Gel core liposomes are a stabilized form of liposomes bearing a core of biocompatible polymer inside the lipid vesicle which serves to prevent the rapid release of antigen content from liposomes (184, 185). Following 2 intramuscular injections with gel core liposomes, Pfs25-specific antibody responses were observed in immunized mice, and these were maintained for up to 8 weeks. Additionally, strong Th1 cytokine (IL-2 and IFN-γ) responses were elicited and these responses were augmented when the gel core liposomes were formulated with CpG oligodeoxynucleotide (CpG-ODN) (184, 185).
A cationic adjuvant liposome formulation (CAF01) consisting of DDAB, synthetic mycobacterial cordfactor as an immunomodulator, and merozoite surface protein 1 (MSP1) antigen derived from P. yoelii genomic DNA, PyMSP1, has been tested in pre-clinical studies as a blood-stage malaria vaccine (186). Compared to the Alum adjuvanted vaccine formulation, immunization with CAF01- Py-MSP-1 resulted in significantly higher antibody and IFN-γ cytokine responses. Furthermore, following challenge, immunization with CAF01- PyMSP1 resulted in significant control of parasite growth (186).
Tyagi et al. (187), utilized a liposome-mediated transdermal immunization approach to deliver P. falciparum merozoite surface protein-1 (PfMSP-1) antigens through intact skin to antigen presenting cells in the skin (187). Similar to observations following immunization with CAF01- PyMSP1(186), durable and stronger parasite-specific humoral responses were observed up to 10 weeks post-immunization. Additionally, robust cell-mediated responses critical in immunity against blood-stage malaria parasites were induced following transdermal administration of elastic liposomes loaded with PfMSP-1 antigens when compared to Alum based vaccine formulations (187). Collectively, these data underscore the substantial superiority of liposome-based formulations over aluminum-based vaccine adjuvant formulations in the induction of parasite-specific immune responses to malaria.
More recently, our group formulated liposomes with mannosylated lipid core peptides (MLCPs) as targeting ligands for the delivery of whole blood-stage parasite antigens to professional antigen presenting cells (188). Immunization with these mannosylated liposome formulations resulted in the induction of significant CD8+ T cell responses; immunized mice demonstrated better control of parasitemia as well as extended survival following challenge, when compared with control mice, availing an alternative delivery system for inactivated whole parasite antigens (188). Together, the studies above indicate that liposomes are being considerably used in malaria vaccine development for targeting all of the different life-cycle stages and their pliability can be further explored to develop a multi-stage vaccine.
Concluding Remarks
The quest for a vaccine against malaria continues despite the partial success with RTS, S/AS01, which showed modest efficacy in phase III clinical trials (16). Given the complex network of immune responses elicited following infection with Plasmodium parasites, an ideal vaccine should aim to induce the appropriate life-cycle stage-specific-antibody and cell-mediated responses capable of protecting against disease and immunopathology. The versatility of liposomes can be exploited to achieve an optimal formulation via the use of charged lipids to promote antigen retention at the injection site (depot-effect) (186), inclusion of targeting ligands to promote uptake by professional APCs (188), to control their stability, release of antigen, enhance antigen protection, and include immunomodulators (40, 43). Additionally, as the poor efficacy of most malaria vaccines evaluated thus far in field trials has been attributed to antigenic polymorphism, the use of whole parasite antigens (188) in liposome formulations needs to be explored further in malaria vaccine development. In summary, the modification of vesicle physicochemical properties may be further exploited to design an optimal liposome formulation with a high level of efficacy required for complete eradication of malaria by 2030 (8).
Author Contributions
AS, DS, and MG drafted and reviewed original manuscript. AG, MZ, MS, and IT critically reviewed original manuscript and provided important intellectual content.
Conflict of Interest Statement
The authors declare that the research was conducted in the absence of any commercial or financial relationships that could be construed as a potential conflict of interest.
Acknowledgments
We wish to thank Dr. Leanne M. Low for critically reviewing this manuscript. We acknowledge the generous support from Griffith University (Griffith University International Postgraduate Research Scholarship and Griffith University Postgraduate Research Scholarship) awarded to AS. We also acknowledge the grant support from a National Health and Medical Research Council Fellowship and Program Grant 1037304 awarded to IT and MG.
Footnotes
1. ^A Safety and Efficacy Study of R21 +/- ChAd63/MVA ME-TRAP. https://ClinicalTrials.gov/show/NCT02905019.
References
1. Russell PF, Mohan BN. The immunisation of fowls against mosquito-borne Plasmodium Gallinaceum by injections of serum and of inactivated homologous sporozoites. J Exp Med. (1942) 76:477–95. doi: 10.1084/jem.76.5.477
2. Hill AV. Vaccines against malaria. Philos Trans R Soc Lond B Biol Sci. (2011) 366:2806–14. doi: 10.1098/rstb.2011.0091
3. Freund J, Sommer HE, Walter AW. Immunisation against malaria: vaccination of ducks with killed parasites incorporated with adjuvants. Science (1945) 102:200–2. doi: 10.1126/science.102.2643.200
4. Freund J, Thomson KJ, Sommer HE, Walter AW, Schenkein EL. Immunisation of rhesus monkeys against malarial infection (P. knowlesi) with killed parasites and adjuvants. Science (1945) 102:202–4. doi: 10.1126/science.102.2643.202
5. Nussenzweig RS, Vanderberg J, Most H, Orton C. Protective immunity produced by the injection of x-irradiated sporozoites of Plasmodium berghei. Nature (1967) 216:160–2. doi: 10.1038/216160a0
6. Kaslow DC, Biernaux S. RTS,S: toward a first landmark on the malaria vaccine technology roadmap. Vaccine (2015) 33:7425–32. doi: 10.1016/j.vaccine.2015.09.061
7. World Health Organization. Malaria vaccine: WHO position paper, January 2016-Recommendations. Vaccine (2018) 36:3576–7. doi: 10.1016/j.vaccine.2016.10.047
9. Perrie Y, Crofts F, Devitt A, Griffiths HR, Kastner E, Nadella V. Designing liposomal adjuvants for the next generation of vaccines. Adv Drug Deliv Rev (2016) 99(Pt A):85–96. doi: 10.1016/j.addr.2015.11.005
10. Brito LA, O'Hagan DT. Designing and building the next generation of improved vaccine adjuvants. J Control Release (2014) 190:563–79. doi: 10.1016/j.jconrel.2014.06.027
11. Nevagi RJ, Toth I, Skwarczynski M. Peptide-based vaccines. In: Koutsopoulos S, editor. Peptide Applications in Biomedicine, Biotechnology and Bioengineering. Oxford: Elsevier Inc. (2018). p. 327–58.
12. Baylor NW, Egan W, Richman P. Aluminum salts in vaccines–US perspective. Vaccine (2002) 20 (Suppl. 3):S18#x02013;23. doi: 10.1016/S0264-410X(02)00166-4
13. O'Hagan DT, Ott GS, De Gregorio E, Seubert A. The mechanism of action of MF59 - an innately attractive adjuvant formulation. Vaccine (2012) 30:4341–8. doi: 10.1016/j.vaccine.2011.09.061
14. Durando P, Icardi G, Ansaldi F. MF59-adjuvanted vaccine: a safe and useful tool to enhance and broaden protection against seasonal influenza viruses in subjects at risk. Expert Opin Biol Ther. (2010) 10:639–51. doi: 10.1517/14712591003724662
15. Lal H, Cunningham AL, Godeaux O, Chlibek R, Diez-Domingo J, Hwang SJ, et al. Efficacy of an adjuvanted herpes zoster subunit vaccine in older adults. N Engl J Med. (2015) 372:2087–96. doi: 10.1056/NEJMoa1501184
16. RTSS Clinical Trials Partnership (2015). Efficacy and safety of RTS,S/AS01 malaria vaccine with or without a booster dose in infants and children in Africa: final results of a phase 3, individually randomised, controlled trial. Lancet 386:31–45. doi: 10.1016/S0140-6736(15)60721-8
17. Regules JA, Cummings JF, Ockenhouse CF. The RTS,S vaccine candidate for malaria. Expert Rev Vaccines (2011) 10:589–99. doi: 10.1586/erv.11.57
18. Nohynek H, Jokinen J, Partinen M, Vaarala O, Kirjavainen T, Sundman J, et al. AS03 adjuvanted AH1N1 vaccine associated with an abrupt increase in the incidence of childhood narcolepsy in Finland. PLoS ONE (2012) 7:e33536. doi: 10.1371/journal.pone.0033536
19. Morel S, Didierlaurent A, Bourguignon P, Delhaye S, Baras B, Jacob V, et al. Adjuvant System AS03 containing alpha-tocopherol modulates innate immune response and leads to improved adaptive immunity. Vaccine (2011) 29:2461–73. doi: 10.1016/j.vaccine.2011.01.011
20. Kundi M, Kundi M. New hepatitis B vaccine formulated with an improved adjuvant system. Expert Rev Vaccines (2007) 6:133–40. doi: 10.1586/14760584.6.2.133
21. Paavonen J, Jenkins D, Bosch FX, Naud P, Salmerón J, Wheeler CM, et al. Efficacy of a prophylactic adjuvanted bivalent L1 virus-like-particle vaccine against infection with human papillomavirus types 16 and 18 in young women: an interim analysis of a phase III double-blind, randomised controlled trial. Lancet (2007) 369:2161–70. doi: 10.1016/S0140-6736(07)60946-5
22. Garçon N, Chomez P, Van Mechelen M. GlaxoSmithKline adjuvant systems in vaccines: concepts, achievements and perspectives. Expert Rev Vaccines (2007) 6:723–39. doi: 10.1586/14760584.6.5.723
23. Rodríguez PC, Rodríguez G, González G, Lage A. Clinical development and perspectives of CIMAvax EGF, Cuban vaccine for non-small-cell lung cancer therapy. MEDICC Rev. (2010) 12:17–23.
24. Bovier PA. Recent advances with a virosomal hepatitis A vaccine. Expert Opin Biol Ther. (2008) 8:1177–85. doi: 10.1517/14712598.8.8.1177
25. Mischler R, Metcalfe IC. Inflexal V a trivalent virosome subunit influenza vaccine: production. Vaccine (2002) 20 (Suppl. 5):B17–23 doi: 10.1016/S0264-410X(02)00512-1
26. Román VR, Jensen KJ, Jensen SS, Leo-Hansen C, Jespersen S, da Silva Te D, et al. Therapeutic vaccination using cationic liposome-adjuvanted HIV type 1 peptides representing HLA-supertype-restricted subdominant T cell epitopes: safety, immunogenicity, and feasibility in Guinea-Bissau. AIDS Res Hum Retroviruses (2013) 29:1504–12. doi: 10.1089/AID.2013.0076
27. van Dissel JT, Joosten SA, Hoff ST, Soonawala D, Prins C, Hokey DA, et al. A novel liposomal adjuvant system, CAF01, promotes long-lived Mycobacterium tuberculosis-specific T-cell responses in human. Vaccine (2014) 32:7098–107. doi: 10.1016/j.vaccine.2014.10.036
28. Luabeya AK, Kagina BM, Tameris MD, Geldenhuys H, Hoff ST, Shi Z, et al. First-in-human trial of the post-exposure tuberculosis vaccine H56:IC31 in Mycobacterium tuberculosis infected and non-infected healthy adults. Vaccine (2015) 33:4130–40. doi: 10.1016/j.vaccine.2015.06.051
29. Norrby M, Vesikari T, Lindqvist L, Maeurer M, Ahmed R, Mahdavifar S, et al. Safety and immunogenicity of the novel H4:IC31 tuberculosis vaccine candidate in BCG-vaccinated adults: Two phase I dose escalation trials. Vaccine (2017) 35:1652–61. doi: 10.1016/j.vaccine.2017.01.055
30. Hussein J, Zewdie M, Yamuah L, Bedru A, Abebe M, Dagnew AF, et al. A phase I, open-label trial on the safety and immunogenicity of the adjuvanted tuberculosis subunit vaccine H1/IC31(R) in people living in a TB-endemic area. Trials (2018) 19:24. doi: 10.1186/s13063-017-2354-0
31. Levine AS, Levy HB. Phase I-II trials of poly IC stabilized with poly-L-lysine. Cancer Treat Rep. (1978) 62:1907–12.
32. Daayana S, Elkord E, Winters U, Pawlita M, Roden R, Stern PL, et al. Phase II trial of imiquimod and HPV therapeutic vaccination in patients with vulval intraepithelial neoplasia. Br J Cancer (2010) 102:1129–36. doi: 10.1038/sj.bjc.6605611
33. Drane D, Gittleson C, Boyle J, Maraskovsky E. ISCOMATRIX adjuvant for prophylactic and therapeutic vaccines. Expert Rev Vaccines (2007) 6:761–72. doi: 10.1586/14760584.6.5.761
34. Maraskovsky E, Schnurr M, Wilson NS, Robson NC, Boyle J, Drane D. Development of prophylactic and therapeutic vaccines using the ISCOMATRIX adjuvant. Immunol Cell Biol. (2009) 87:371–6. doi: 10.1038/icb.2009.21
35. Morelli AB, Becher D, Koernig S, Silva A, Drane D, Maraskovsky E. ISCOMATRIX: a novel adjuvant for use in prophylactic and therapeutic vaccines against infectious diseases. J Med Microbiol. (2012) 61 (Pt 7):935–43. doi: 10.1099/jmm.0.040857-0
36. Behzad H, Huckriede AL, Haynes L, Gentleman B, Coyle K, Wilschut JC, et al. GLA-SE, a synthetic toll-like receptor 4 agonist, enhances T-cell responses to influenza vaccine in older adults. J Infect Dis. (2012) 205:466–73. doi: 10.1093/infdis/jir769
37. McKenzie A, Watt M, Gittleson C. ISCOMATRIX() vaccines: safety in human clinical studies. Hum Vaccin (2010) 6:237–46. doi: 10.4161/hv.6.3.10754
38. Stratmann T. Cholera Toxin Subunit B as adjuvant–an accelerator in protective immunity and a break in autoimmunity. Vaccines (2015) 3:579–96. doi: 10.3390/vaccines3030579
39. Allison AG, Gregoriadis G. Liposomes as immunological adjuvants. Nature (1974) 252:252. doi: 10.1038/252252a0
40. Schwendener RA. Liposomes as vaccine delivery systems: a review of the recent advances. Ther Adv Vaccines (2014) 2:159–82. doi: 10.1177/2051013614541440
41. Marasini N, Ghaffar KA, Skwarczynski M, Toth I. Liposomes as a vaccine delivery system. In: Skwarczynski M, Toth I, editors. Micro and Nanotechnologies for Vaccine Development. Oxford, UK: Elsevier Inc. (2017). p. 221–39. doi: 10.1016/B978-0-323-39981-4.00012-9
42. Ghaffar KA, Giddam AK, Zaman M, Skwarczynski M, Toth I. Liposomes as nanovaccine delivery systems. Curr Top Med Chem. (2014) 14:1194–208. doi: 10.2174/1568026614666140329232757
43. Giddam AK, Zaman M, Skwarczynski M, Toth I. Liposome-based delivery system for vaccine candidates: constructing an effective formulation. Nanomedicine (2012) 7:1877–93. doi: 10.2217/nnm.12.157
44. Tran TM, Li S, Doumbo S, Doumtabe D, Huang CY, Dia S, et al. An intensive longitudinal cohort study of Malian children and adults reveals no evidence of acquired immunity to Plasmodium falciparum infection. Clin Infect Dis. (2013) 57:40–7. doi: 10.1093/cid/cit174
45. Stanisic DI, Good MF. Examining cellular immune responses to inform development of a blood-stage malaria vaccine. Parasitology (2016) 143:208–23. doi: 10.1017/S0031182015001092
46. Fujioka H, Aikawa M. The malaria parasite and its life-cycle. In: editors M. Wahlgren and P. Perlmann, Malaria Molecular and Clinical Aspects. Amsterdam: Harwood Academic Publishers (1999). p. 19–53.
47. Chatterjee S, Wery M, Sharma P, Chauhan VS. A conserved peptide sequence of the Plasmodium falciparum circumsporozoite protein and antipeptide antibodies inhibit Plasmodium berghei sporozoite invasion of Hep-G2 cells and protect immunized mice against P. berghei sporozoite challenge. Infect Immun. (1995) 63:4375–81.
48. Sultan AA, Thathy V, Frevert U, Robson KJ, Crisanti A, Nussenzweig V, et al. TRAP is necessary for gliding motility and infectivity of Plasmodium sporozoites. Cell (1997) 90:511–22. doi: 10.1016/S0092-8674(00)80511-5
49. John CC, Zickafoose JS, Sumba PO, King CL, Kazura JW. Antibodies to the Plasmodium falciparum antigens circumsporozoite protein, thrombospondin-related adhesive protein, and liver-stage antigen 1 vary by ages of subjects and by season in a highland area of Kenya. Infect Immun. (2003) 71:4320–5. doi: 10.1128/IAI.71.8.4320-4325.2003
50. John CC, Moormann AM, Pregibon DC, Sumba PO, McHugh MM, Narum DL, et al. Correlation of high levels of antibodies to multiple pre-erythrocytic Plasmodium falciparum antigens and protection from infection. Am J Trop Med Hyg. (2005) 73:222–8. doi: 10.4269/ajtmh.2005.73.222
51. Offeddu V, Thathy V, Marsh K, Matuschewski K. Naturally acquired immune responses against Plasmodium falciparum sporozoites and liver infection. Int J Parasitol. (2012) 42:535–48. doi: 10.1016/j.ijpara.2012.03.011
52. Vanderberg JP, Frevert U. Intravital microscopy demonstrating antibody-mediated immobilisation of Plasmodium berghei sporozoites injected into skin by mosquitoes. Int J Parasitol. (2004) 34:991–6. doi: 10.1016/j.ijpara.2004.05.005
53. Sinnis P, Coppi A. A long and winding road: the Plasmodium sporozoite's journey in the mammalian host. Parasitol Int. (2007) 56:171–8. doi: 10.1016/j.parint.2007.04.002
54. Seder RA, Chang LJ, Enama ME, Zephir KL, Sarwar UN, Gordon IJ, et al. Protection against malaria by intravenous immunisation with a nonreplicating sporozoite vaccine (PfSPZ). Science (2013) 341:1359–65. doi: 10.1126/science.1241800
55. Ishizuka AS, Lyke KE, DeZure A, Berry AA, Richie TL, Mendoza FH, et al. Protection against malaria at 1 year and immune correlates following PfSPZ vaccination. Nat Med. (2016) 22:614–23. doi: 10.1038/nm.4110
56. Epstein JE, Paolino KM, Richie TL, Sedegah M, Singer A, Ruben AJ, et al. Protection against Plasmodium falciparum malaria by PfSPZ vaccine. JCI Insight (2017) 2:e89154. doi: 10.1172/jci.insight.89154
57. Lyke KE, Ishizuka AS, Berry AA, Chakravarty S, DeZure A, Enama ME, et al. Attenuated PfSPZ Vaccine induces strain-transcending T cells and durable protection against heterologous controlled human malaria infection. Proc Natl Acad Sci USA. (2017) 114:2711–6. doi: 10.1073/pnas.1615324114
58. Sissoko MS, Healy SA, Katile A, Omaswa F, Zaidi I, Gabriel EE, et al. Safety and efficacy of PfSPZ Vaccine against Plasmodium falciparum via direct venous inoculation in healthy malaria-exposed adults in Mali: a randomised, double-blind phase 1 trial. Lancet Infect Dis. (2017) 17:498–509. doi: 10.1016/S1473-3099(17)30104-4
59. Cohen J, Nussenzweig V, Nussenzweig R, Vekemans J, Leach A. From the circumsporozoite protein to the RTS, S/AS01 candidate vaccine. Hum Vaccine (2010) 6:90–6. doi: 10.4161/hv.6.1.9677
60. Agnandji ST, Lell B, Soulanoudjingar SS, Fernandes JF, Abossolo BP, et al. First results of phase 3 trial of RTS,S/AS01 malaria vaccine in African children. N Engl J Med. (2011) 365:1863–75. doi: 10.1056/NEJMoa1102287
61. Olotu A, Moris P, Mwacharo J, Vekemans J, Kimani D, Janssens M, et al. Circumsporozoite-specific T cell responses in children vaccinated with RTS,S/AS01E and protection against P. falciparum clinical malaria. PLoS ONE (2011) 6:e25786. doi: 10.1371/journal.pone.0025786
62. White MT, Verity R, Griffin JT, Asante KP, Owusu-Agyei S, Greenwood B, et al. Immunogenicity of the RTS,S/AS01 malaria vaccine and implications for duration of vaccine efficacy: secondary analysis of data from a phase 3 randomised controlled trial. Lancet Infect Dis. (2015) 15:1450–8. doi: 10.1016/S1473-3099(15)00239-X
63. Agnandji ST, Lell B, Fernandes JF, Abossolo BP, Methogo BG, et al. A phase 3 trial of RTS,S/AS01 malaria vaccine in African infants. N Engl J Med. (2012) 367:2284–95. doi: 10.1056/NEJMoa1208394
64. Schofield L, Villaquiran J, Ferreira A, Schellekens H, Nussenzweig R, Nussenzweig V. Gamma interferon, CD8+ T cells and antibodies required for immunity to malaria sporozoites. Nature (1987) 330:664–6. doi: 10.1038/330664a0
65. Weiss WR, Sedegah M, Beaudoin RL, Miller LH, Good MF. CD8+ T cells (cytotoxic/suppressors) are required for protection in mice immunized with malaria sporozoites. Proc Natl Acad Sci USA. (1988) 85:573–6. doi: 10.1073/pnas.85.2.573
66. Seguin MC, Klotz FW, Schneider I, Weir JP, Goodbary M, Slayter M, et al. Induction of nitric oxide synthase protects against malaria in mice exposed to irradiated Plasmodium berghei infected mosquitoes: involvement of interferon gamma and CD8+ T cells. J Exp Med. (1994) 180:353–8. doi: 10.1084/jem.180.1.353
67. Krzych U, Lyon JA, Jareed T, Schneider I, Hollingdale MR, Gordon DM, et al. T-lymphocytes from volunteers immunized with irradiated Plasmodium falciparum sporozoites recognize liver and blood-stage malaria antigens. J Immunol. (1995) 155:4072–7.
68. Doolan DL, Hoffman SL. IL-12 and NK cells are required for antigen-specific adaptive immunity against malaria initiated by CD8+ T cells in the Plasmodium yoelii model. J Immunol. (1999) 163:884–92.
69. Hoffman SL, Goh LM, Luke TC, Schneider I, Le TP, Doolan D, et al. Protection of humans against malaria by immunisation with radiation-attenuated Plasmodium falciparum sporozoites. J Infect Dis. (2002) 185:1155–64. doi: 10.1086/339409
70. Hollingdale MR, Krzych U. Immune responses to liver-stage parasites: implications for vaccine development. In: Perlmann P, Troye-Blomberg M, editors. Malaria Immunology. Basel: Karger (2002). p. 27.
71. Sinnis P, Nardin E. Sporozoite antigens: biology and immunology of the circumsporozoite protein and thrombospondin-related anonymous protein. In: Perlmann P, Troye-Blomberg M, editors. Malaria Immunology Basel: Karger (2002). p. 26.
72. Steers N, Schwenk R, Bacon DJ, Berenzon D, Williams J, Krzych U. The immune status of Kupffer cells profoundly influences their responses to infectious Plasmodium berghei sporozoites. Eur J Immunol. (2005) 35:2335–46. doi: 10.1002/eji.200425680
73. Roestenberg M, McCall M, Hopman J, Wiersma J, Luty AJ, van Gemert GJ, et al. Protection against a malaria challenge by sporozoite inoculation. N Engl J Med. (2009) 361:468–77. doi: 10.1056/NEJMoa0805832
74. Roestenberg M, Teirlinck AC, McCall MB, Teelen K, Makamdop KN, Wiersma J, et al. Long-term protection against malaria after experimental sporozoite inoculation: an open-label follow-up study. Lancet (2011) 377:1770–6. doi: 10.1016/S0140-6736(11)60360-7
75. Bijker EM, Bastiaens GJ, Teirlinck AC, van Gemert GJ, Graumans W, van de Vegte-Bolmer M, et al. Protection against malaria after immunisation by chloroquine prophylaxis and sporozoites is mediated by preerythrocytic immunity. Proc Natl Acad Sci USA. (2013) 110:7862–7. doi: 10.1073/pnas.1220360110
76. Mordmüller B, Surat G, Lagler H, Chakravarty S, Ishizuka AS, Lalremruata A, et al. Sterile protection against human malaria by chemoattenuated PfSPZ vaccine. Nature (2017) 542:445–9. doi: 10.1038/nature21060
77. Behet MC, Foquetm L, van Gemert GJ, Bijker EM, Meuleman P, Leroux-Roels G. Sporozoite immunisation of human volunteers under chemoprophylaxis induces functional antibodies against pre-erythrocytic stages of Plasmodium falciparum. Malar J. (2014) 13:136. doi: 10.1186/1475-2875-13-136
78. Stevenson MM, Riley EM. Innate immunity to malaria. Nat Rev Immunol. (2004) 4:169–80. doi: 10.1038/nri1311
79. Crompton PD, Moebius J, Portugal S, Waisberg M, Hart G, Garver LS, et al. Malaria immunity in man and mosquito: insights into unsolved mysteries of a deadly infectious disease. Annu Rev Immunol. (2014) 32:157–87. doi: 10.1146/annurev-immunol-032713-120220
80. Krishnegowda G, Hajjar AM, Zhu J, Douglass EJ, Uematsu S, Akira S, et al. Induction of proinflammatory responses in macrophages by the glycosylphosphatidylinositols of Plasmodium falciparum: cell signaling receptors, glycosylphosphatidylinositol (GPI) structural requirement, and regulation of GPI activity. J Biol Chem. (2005) 280:8606–16. doi: 10.1074/jbc.M413541200
81. Parroche P, Lauw FN, Goutagny N, Latz E, Monks BG, Visintin A, et al. Malaria hemozoin is immunologically inert but radically enhances innate responses by presenting malaria DNA to Toll-like receptor 9. Proc Natl Acad Sci USA. (2007) 104:1919–24. doi: 10.1073/pnas.0608745104
82. Shio MT, Eisenbarth SC, Savaria M, Vinet AF, Bellemare MJ, Harder KW, et al. Malarial hemozoin activates the NLRP3 inflammasome through Lyn and Syk kinases. PLoS Pathog. (2009) 5:e1000559. doi: 10.1371/annotation/abca067d-b82b-4de6-93c5-0fcc38e3df05
83. Sharma S, DeOliveira RB, Kalantari P, Parroche P, Goutagny N, Jiang Z, et al. Innate immune recognition of an AT-rich stem-loop DNA motif in the Plasmodium falciparum genome. Immunity (2011) 35:194–207. doi: 10.1016/j.immuni.2011.05.016
84. Ing R, Stevenson MM. Dendritic cell and NK cell reciprocal cross talk promotes gamma interferon-dependent immunity to blood-stage Plasmodium chabaudi AS infection in mice. Infect Immun. (2009) 77:770–82. doi: 10.1128/IAI.00994-08
85. Stevenson MM, Ing R, Berretta F, Miu J. Regulating the adaptive immune response to blood-stage malaria: role of dendritic cells and CD4(+)Foxp3(+) regulatory T cells. Int J Biol Sci. (2011) 7:1311–22. doi: 10.7150/ijbs.7.1311
86. Urban BC, Ing R, Stevenson MM. Early interactions between blood-stage Plasmodium parasites and the immune system. Curr Top Microbiol Immunol. (2005) 297:25–70. doi: 10.1007/3-540-29967-X_2
87. Hansen DS, D'Ombrain MC, Schofield L. The role of leukocytes bearing natural killer complex receptors and killer immunoglobulin-like receptors in the immunology of malaria. Curr Opin Immunol. (2007) 19:416–23. doi: 10.1016/j.coi.2007.07.011
88. Porcherie A, Mathieu C, Peronet R, Schneider E, Claver J, Commere PH, et al. Critical role of the neutrophil-associated high-affinity receptor for IgE in the pathogenesis of experimental cerebral malaria. J Exp Med. (2011) 208:2225–36. doi: 10.1084/jem.20110845
89. Horowitz A, Newman KC, Evans JH, Korbel DS, Davis DM, Riley EM. Cross-talk between T cells and NK cells generates rapid effector responses to Plasmodium falciparum-infected erythrocytes. J Immunol. (2010) 184:6043–52. doi: 10.4049/jimmunol.1000106
90. Stanisic DI, Cutts J, Eriksson E, Fowkes FJ, Rosanas-Urgell A, Siba P, et al. γδ T cells and CD14+ monocytes are predominant cellular sources of cytokines and chemokines associated with severe malaria. J Infect Dis. 210:295–305. (2014). doi: 10.1093/infdis/jiu083
91. Jagannathan P, Kim CC, Greenhouse B, Nankya F, Bowen K, Eccles-James I, et al. Loss and dysfunction of Vdelta2(+) gammadelta T cells are associated with clinical tolerance to malaria. Sci Transl Med. (2014) 6:251ra117. doi: 10.1126/scitranslmed.3009793
92. Kurup SP, Harty JT. γδ T cells and immunity to human malaria in endemic regions. Ann Transl Med. (2015) 3(Suppl. 1):S22. doi: 10.3978/j.issn.2305-5839.2015.02.22
93. Cohen S, Mc Gregor IA, Carrington S. Gamma-globulin and acquired immunity to human malaria. Nature (1961) 192:733–7. doi: 10.1038/192733a0
94. McGregor IA, Carrington SP, Cohen S. Treatment of East African P. falciparum malaria with West African human γ-globulin. Trans R Soc Trop Med Hyg. (1963) 57:170–5. doi: 10.1016/0035-9203(63)90058-0
95. Sabchareon A, Burnouf T, Ouattara D, Attanath P, Bouharoun-Tayoun H, Chantavanich P, et al. Parasitologic and clinical human response to immunoglobulin administration in falciparum malaria. Am J Trop Med Hyg. (1991) 45:297–308. doi: 10.4269/ajtmh.1991.45.297
96. Wåhlin B, Wahlgren M, Perlmann H, Berzins K, Björkman A, Patarroyo ME, et al. Human antibodies to a Mr 155,000 Plasmodium falciparum antigen efficiently inhibit merozoite invasion. Proc Natl Acad Sci USA. (1984) 81:7912–6.
97. Udeinya IJ, Schmidt JA, Aikawa M, Miller LH, Green I. Falciparum malaria-infected erythrocytes specifically bind to cultured human endothelial cells. Science (1981) 213:555–7. doi: 10.1126/science.7017935
98. Treutiger CJ, Hedlund I, Helmby H, Carlson J, Jepson A, Twumasi P, et al. Rosette formation in Plasmodium falciparum isolates and anti-rosette activity of sera from Gambians with cerebral or uncomplicated malaria. Am J Trop Med Hyg. (1992) 46:503–10. doi: 10.4269/ajtmh.1992.46.503
99. Bouharoun-Tayoun H, Oeuvray C, Lunel F, Druilhe P. Mechanisms underlying the monocyte-mediated antibody-dependent killing of Plasmodium falciparum asexual blood stages. J Exp Med. (1995) 182:409–18. doi: 10.1084/jem.182.2.409
100. Perlmann P, Troye-Blomberg M. Malaria and the immune system in humans. In: Perlmann P, Troye-Blomberg M, editors. Malaria Immunology. Basel: Kager (2002). p. 229–242. doi: 10.1159/000058846
101. Süss G, Eichmann K, Kury E, Linke A, Langhorne J. Roles of CD4- and CD8-bearing T lymphocytes in the immune response to the erythrocytic stages of Plasmodium chabaudi. Infect Immun. (1988) 56:3081–8.
102. Podoba JE, Stevenson MM. CD4+ and CD8+ T lymphocytes both contribute to acquired immunity to blood-stage Plasmodium chabaudi AS. Infect Immun. (1991) 59:51–8.
103. Amante FH, Good MF. Prolonged Th1-like response generated by a Plasmodium yoelii-specific T cell clone allows complete clearance of infection in reconstituted mice. Parasite Immunol. (1997) 19:16. doi: 10.1046/j.1365-3024.1997.d01-187.x
104. Langhorne J, Gillard S, Simon B, Slade S, Eichmann K. Frequencies of CD4+ T cells reactive with Plasmodium chabaudi chabaudi: distinct response kinetics for cells with Th1 and Th2 characteristics during infection. Int Immunol. (1989) 1:416–24. doi: 10.1093/intimm/1.4.416
105. Langhorne J, Meding SJ, Eichmann K, Gillard SS. The response of CD4+ T cells to Plasmodium chabaudi chabaudi. Immunol Rev. (1989) 112:71–94. doi: 10.1111/j.1600-065X.1989.tb00553.x
106. Perez-Mazliah D, Langhorne J. CD4 T-cell subsets in malaria: TH1/TH2 revisited. Front Immunol. (2015) 5:671. doi: 10.3389/fimmu.2014.00671
107. Pombo DJ, Lawrence G, Hirunpetcharat C, Rzepczyk C, Bryden M, Cloonan N, et al. Immunity to malaria after administration of ultra-low doses of red cells infected with Plasmodium falciparum. Lancet (2002) 360:610–7. doi: 10.1016/S0140-6736(02)09784-2
108. Edstein MD, Kotecka BM, Anderson KL, Pombo DJ, Kyle DE, Rieckmann KH, et al. Lengthy antimalarial activity of atovaquone in human plasma following atovaquone-proguanil administration. Antimicrob Agents Chemother. (2005) 49:4421–2. doi: 10.1128/AAC.49.10.4421-4422.2005
109. Robinson LJ, D'Ombrain MC, Stanisic DI, Taraika J, Bernard N, Richards JS, et al. Cellular tumor necrosis factor, gamma interferon, and interleukin-6 responses as correlates of immunity and risk of clinical Plasmodium falciparum malaria in children from Papua New Guinea. Infect Immun. (2009) 77:3033–43. doi: 10.1128/IAI.00211-09
110. Jagannathan P, Eccles-James I, Bowen K, Nankya F, Auma A, Wamala S, et al. IFNγ/IL-10 co-producing cells dominate the CD4 response to malaria in highly exposed children. PLoS Pathog. (2014) 10:e1003864. doi: 10.1371/journal.ppat.1003864
111. Boyle MJ, Jagannathan P, Bowen K, McIntyre TI, Vance HM, Farrington LA, et al. Effector phenotype of Plasmodium falciparum-specific CD4(+) T cells is influenced by both age and transmission intensity in naturally exposed populations. J Infect Dis. (2015) 212:416–25. doi: 10.1093/infdis/jiv054
112. Breitfeld D, Ohl L, Kremmer E, Ellwart J, Sallusto F, Lipp M, et al. Follicular B helper T cells express CXC chemokine receptor 5, localize to B cell follicles, and support immunoglobulin production. J Exp Med. (2000) 192:1545–52. doi: 10.1084/jem.192.11.1545
113. Schaerli P, Willimann K, Lang AB, Lipp M, Loetscher P, Moser B. CXC chemokine receptor 5 expression defines follicular homing T cells with B cell helper function. J Exp Med. (2000) 192:1553–62. doi: 10.1084/jem.192.11.1553
114. Kim CH, Rott LS, Clark-Lewis I, Campbell DJ, Wu L, Butcher EC. Subspecialization of CXCR5(+) T cells: B helper activity is focused in a germinal center-localized subset of CXCR5(+) T cells. J Exp Med. (2001) 193:1373–81. doi: 10.1084/jem.193.12.1373
115. Pérez-Mazliah D, Nguyen MP, Hosking C, McLaughlin S, Lewis MD, Tumwine I, et al. Follicular helper T cells are essential for the elimination of Plasmodium infection. EBio Med. (2017) 24:216–30. doi: 10.1016/j.ebiom.2017.08.030
116. Stockinger B, Kassiotis G, Bourgeois C. Homeostasis and T cell regulation. Curr Opin Immunol. (2004) 16:775–9. doi: 10.1016/j.coi.2004.09.003
117. Tang Q, Bluestone JA. The Foxp3+ regulatory T cell: a jack of all trades, master of regulation. Nat Immunol. (2008) 9:239–44. doi: 10.1038/ni1572
118. Omer FM, Riley EM. Transforming growth factor beta production is inversely correlated with severity of murine malaria infection. J Exp Med. (1998) 188:39–48. doi: 10.1084/jem.188.1.39
119. Day NP, Hien TT, Schollaardt T, Loc PP, Chuong LV, Chau TT, et al. The prognostic and pathophysiologic role of pro- and antiinflammatory cytokines in severe malaria. J Infect Dis. (1999) 180:1288–97. doi: 10.1086/315016
120. Li C, Corraliza I, Langhorne J. A defect in interleukin-10 leads to enhanced malarial disease in Plasmodium chabaudi chabaudi infection in mice. Infect Immun. (1999) 67:4435–42.
121. Dodoo D, Omer FM, Todd J, Akanmori BD, Koram KA, Riley EM. Absolute levels and ratios of proinflammatory and anti-inflammatory cytokine production in vitro predict clinical immunity to Plasmodium falciparum malaria. J Infect Dis. (2002) 185:971–9. doi: 10.1086/339408
122. Couper KN, Blount DG, Wilson MS, Hafalla JC, Belkaid Y, Kamanaka M, et al. IL-10 from CD4CD25Foxp3CD127 adaptive regulatory T cells modulates parasite clearance and pathology during malaria infection. PLoS Pathog. (2008) 4:e1000004. doi: 10.1371/journal.ppat.1000004
123. Scholzen A, Mittag D, Rogerson SJ, Cooke BM, Plebanski M. Plasmodium falciparum-mediated induction of human CD25Foxp3 CD4 T cells is independent of direct TCR stimulation and requires IL-2, IL-10 and TGFbeta. PLoS Pathog. (2009) 5:e1000543. doi: 10.1371/journal.ppat.1000543
124. Nie CQ, Bernard NJ, Schofield L, Hansen DS. CD4+ CD25+ regulatory T cells suppress CD4+ T-cell function and inhibit the development of Plasmodium berghei-specific TH1 responses involved in cerebral malaria pathogenesis. Infect Immun. (2007) 75:2275–82. doi: 10.1128/IAI.01783-06
125. Cambos M, Bélanger B, Jacques A, Roulet A, Scorza T. Natural regulatory (CD4+CD25+FOXP+) T cells control the production of pro-inflammatory cytokines during Plasmodium chabaudi adami infection and do not contribute to immune evasion. Int J Parasitol. (2008) 38:229–38. doi: 10.1016/j.ijpara.2007.07.006
126. Hisaeda H, Maekawa Y, Iwakawa D, Okada H, Himeno K, Kishihara K, et al. Escape of malaria parasites from host immunity requires CD4+ CD25+ regulatory T cells. Nat Med. (2004) 10:29–30. doi: 10.1038/nm975
127. Amante FH, Stanley AC, Randall LM, Zhou Y, Haque A, McSweeney K, et al. A role for natural regulatory T cells in the pathogenesis of experimental cerebral malaria. Am J Pathol. (2007) 171:548–59. doi: 10.2353/ajpath.2007.061033
128. Finney OC, Riley EM, Walther M. Regulatory T cells in malaria - friend or foe? Trends Immunol. (2010) 31:63–70. doi: 10.1016/j.it.2009.12.002
129. Hansen DS, Schofield L. Natural regulatory T cells in malaria: host or parasite allies? PLoS Pathog (2010) 6:e1000771. doi: 10.1371/journal.ppat.1000771
130. Scholzen A, Minigo G, Plebanski M. Heroes or villains? T regulatory cells in malaria infection. Trends Parasitol. (2010) 26:16–25. doi: 10.1016/j.pt.2009.10.004
131. Walther M, Tongren JE, Andrews L, Korbel D, King E, Fletcher H, et al. Upregulation of TGF-beta, FOXP3, and CD4+CD25+ regulatory T cells correlates with more rapid parasite growth in human malaria infection. Immunity (2005) 23:287–96. doi: 10.1016/j.immuni.2005.08.006
132. Jangpatarapongsa K, Chootong P, Sattabongkot J, Chotivanich K, Sirichaisinthop J, Tungpradabkul S, et al. Plasmodium vivax parasites alter the balance of myeloid and plasmacytoid dendritic cells and the induction of regulatory T cells. Eur J Immunol. (2008) 38:2697–705. doi: 10.1002/eji.200838186
133. Minigo G, Woodberry T, Piera KA, Salwati E, Tjitra E, Kenangalem E, et al. Parasite-dependent expansion of TNF receptor II-positive regulatory T cells with enhanced suppressive activity in adults with severe malaria. PLoS Pathog. (2009) 5:e1000402. doi: 10.1371/journal.ppat.1000402
134. Bueno LL, Morais CG, Araújo FF, Gomes JA, Corrêa-Oliveira R, Soares IS, et al. Plasmodium vivax: induction of CD4+CD25+FoxP3+ regulatory T cells during infection are directly associated with level of circulating parasites. PLoS ONE (2010) 5:e9623. doi: 10.1371/journal.pone.0009623
135. Panichakul T, Sattabongkot J, Chotivanich K, Sirichaisinthop J, Cui L, Udomsangpetch R. Production of erythropoietic cells in vitro for continuous culture of Plasmodium vivax. Int J Parasitol. (2007) 37:1551–7. doi: 10.1016/j.ijpara.2007.05.009
136. Tamez PA, Liu H, Fernandez-Pol S, Haldar K, Wickrema A. Stage-specific susceptibility of human erythroblasts to Plasmodium falciparum malaria infection. Blood (2009) 114:3652–5. doi: 10.1182/blood-2009-07-231894
137. Lundie RJ, de Koning-Ward TF, Davey GM, Nie CQ, Hansen DS, Lau LS, et al. Blood-stage Plasmodium infection induces CD8+ T lymphocytes to parasite-expressed antigens, largely regulated by CD8alpha+ dendritic cells. Proc Natl Acad Sci USA. (2008) 105:14509–14. doi: 10.1073/pnas.0806727105
138. Imai T, Ishida H, Suzue K, Hirai M, Taniguchi T, Okada H, et al. CD8(+) T cell activation by murine erythroblasts infected with malaria parasites. Sci Rep. (2013) 3:1572. doi: 10.1038/srep01572
139. Imai T, Ishida H, Suzue K, Taniguchi T, Okada H, Shimokawa C, et al. Cytotoxic activities of CD8(+) T cells collaborate with macrophages to protect against blood-stage murine malaria. Elife (2015) 4:e04232. doi: 10.7554/eLife.04232
140. Imai T, Shen J, Chou B, Duan X, Tu L, Tetsutani K, et al. Involvement of CD8+ T cells in protective immunity against murine blood-stage infection with Plasmodium yoelii 17XL strain. Eur J Immunol. (2010) 40:1053–61. doi: 10.1002/eji.200939525
141. Horne-Debets JM, Faleiro R, Karunarathne DS, Liu XQ, Lineburg KE, Poh CM, et al. PD-1 dependent exhaustion of CD8+ T cells drives chronic malaria. Cell Rep. (2013) 5:1204–13. doi: 10.1016/j.celrep.2013.11.002
142. Mazumdar T, Anam K, Ali N. Influence of phospholipid composition on the adjuvanticity and protective efficacy of liposome-encapsulated Leishmania donovani antigens. J Parasitol. (2005) 91:269–74. doi: 10.1645/GE-356R1
143. van Houte AJ, Snippe H, Schmitz MG, Willers JM. Characterization of immunogenic properties of haptenated liposomal model membranes in mice. V Effect of membrane composition on humoral and cellular immunogenicity. Immunology (1981) 44:561–8.
144. Bakouche O, Gerlier D. Enhancement of immunogenicity of tumour virus antigen by liposomes: the effect of lipid composition. Immunology (1986) 58:507–13.
145. Mannock DA, Lee MY, Lewis RN, McElhaney RN. Comparative calorimetric and spectroscopic studies of the effects of cholesterol and epicholesterol on the thermotropic phase behaviour of dipalmitoylphosphatidylcholine bilayer membranes. Biochim Biophys Acta (2008) 1778:2191–202. doi: 10.1016/j.bbamem.2008.05.004
146. Christensen D, Henriksen-Lacey M, Kamath AT, Lindenstrøm T, Korsholm KS, Christensen JP, et al. A cationic vaccine adjuvant based on a saturated quaternary ammonium lipid have different in vivo distribution kinetics and display a distinct CD4 T cell-inducing capacity compared to its unsaturated analog. J Control Release (2012) 160:468–76. doi: 10.1016/j.jconrel,.2012.03.016
147. Henriksen-Lacey M, Christensen D, Bramwell VW, Lindenstrøm T, Agger EM, Andersen P, et al. Liposomal cationic charge and antigen adsorption are important properties for the efficient deposition of antigen at the injection site and ability of the vaccine to induce a CMI response. J Control Release (2010) 145:102–8. doi: 10.1016/j.jconrel.2010.03.027
148. Henriksen-Lacey M, Bramwell VW, Christensen D, Agger EM, Andersen P, Perrie Y, et al. Liposomes based on dimethyldioctadecylammonium promote a depot effect and enhance immunogenicity of soluble antigen. J Control Release (2010) 142:180–6. doi: 10.1016/j.jconrel.2009.10.022
149. Basile L, Passirani C, Huynh NT, Béjaud J, Benoit JP, Puglisi G, et al. Serum-stable, long-circulating paclitaxel-loaded colloidal carriers decorated with a new amphiphilic PEG derivative. Int J Pharm. (2012) 426:231–8. doi: 10.1016/j.ijpharm.2012.01.038
150. Levchenko TS, Rammohan R, Lukyanov AN, Whiteman KR, Torchilin VP. Liposome clearance in mice: the effect of a separate and combined presence of surface charge and polymer coating. Int J Pharm. (2002) 240:95–102. doi: 10.1016/S0378-5173(02)00129-1
151. Kaur R, Bramwell VW, Kirby DJ, Perrie Y. Pegylation of DDA:TDB liposomal adjuvants reduces the vaccine depot effect and alters the Th1/Th2 immune responses. J Control Release (2012) 158:72–7. doi: 10.1016/j.jconrel.2011.10.012
152. Mann JF, Shakir E, Carter KC, Mullen AB, Alexander J, Ferro VA. Lipid vesicle size of an oral influenza vaccine delivery vehicle influences the Th1/Th2 bias in the immune response and protection against infection. Vaccine (2009) 27:3643–9. doi: 10.1016/j.vaccine.2009.03.040
153. Brewer JM, Pollock KG, Tetley L, Russell DG. Vesicle size influences the trafficking, processing, and presentation of antigens in lipid vesicles. J Immunol (2004) 173:6143–50. doi: 10.4049/jimmunol.173.10.6143
154. Manolova V, Flace A, Bauer M, Schwarz K, Saudan P, Bachmann MF. Nanoparticles target distinct dendritic cell populations according to their size. Eur J Immunol. (2008) 38:1404–13. doi: 10.1002/eji.200737984
155. Henriksen-Lacey M, Devitt A, Perrie Y. The vesicle size of DDA:TDB liposomal adjuvants plays a role in the cell-mediated immune response but has no significant effect on antibody production. J Control Release (2011) 154:131–7. doi: 10.1016/j.jconrel.2011.05.019
156. Ghaffar KA, Marasini N, Giddam AK, Batzloff MR, Good MF, Skwarczynski M, et al. The role of size in development of mucosal liposome-lipopeptide vaccine candidates against group A Streptococcus. Med Chem. (2016) 13:22–7. doi: 10.2174/1573406412666160720093138
157. Janeway CA. How the immune system works to protect the host from infection: a personal view. Proc Natl Acad Sci USA. (2001) 98:7461–8. doi: 10.1073/pnas.131202998
158. Gnjatic S, Sawhney NB, Bhardwaj N. Toll-like receptor agonists: are they good adjuvants? Cancer J. (2010) 16:382–91. doi: 10.1097/PPO.0b013e3181eaca65
159. Tong NK, Beran J, Kee SA, Miguel JL, Sánchez C, Bayas JM, et al. Immunogenicity and safety of an adjuvanted hepatitis B vaccine in pre-hemodialysis and hemodialysis patients. Kidney Int. (2005) 68:2298–303. doi: 10.1111/j.1523-1755.2005.00689.x
160. Monie A, Hung CF, Roden R, Wu TC. Cervarix: a vaccine for the prevention of HPV 16, 18-associated cervical cancer. Biologics (2008) 2:97–105.
161. Vyas SP, Goyal AK, Khatri K. Mannosylated liposomes for targeted vaccines delivery. Methods Mol Biol. (2010) 605:177–88. doi: 10.1007/978-1-60327-360-2_12
162. Marasini N, Giddam AK, Ghaffar KA, Batzloff MR, Good MF, Skwarczynski M, et al. Multilayer engineered nanoliposomes as a novel tool for oral delivery of lipopeptide-based vaccines against group A Streptococcus. Nanomedicine (2016) 11:1223–36. doi: 10.2217/nnm.16.36
163. Mohanan D, Slütter B, Henriksen-Lacey M, Jiskoot W, Bouwstra JA, Perrie Y, et al. Administration routes affect the quality of immune responses: a cross-sectional evaluation of particulate antigen-delivery systems. J Control Release (2010) 147:342–9.
164. Ballou WR, Rothbard J, Wirtz R, Gordon D, Williams J, Gore R, et al. Immunogenicity of synthetic peptides from circumsporozoite protein of Plasmodium falciparum. Science (1985) 228:996–9. doi: 10.1126/science.2988126
165. Alving CR, Richards RL, Moss J, Alving LI, Clements JD, Shiba T, et al. Effectiveness of liposomes as potential carriers of vaccines: applications to cholera toxin and human malaria sporozoite antigen. Vaccine (1986) 4:166–72. doi: 10.1016/0264-410X(86)90005-8
166. Richards RL, Hayre MD, Hockmeyer WT, Alving CR. Liposomes, lipid A, and aluminum hydroxide enhance the immune response to a synthetic malaria sporozoite antigen. Infect Immun. (1988) 56:682–6.
167. Alving CR, Richards RL, Hayre MD, Hockmeyer WT, Wirtz RA. Liposomes as carriers of vaccines: development of a liposomal malaria vaccine. In: Gregoriadis G, Allison AC, Poste G, editors. Immunological Adjuvants and Vaccines. Boston, MA: Springer (1989). p. 123–31. doi: 10.1007/978-1-4757-0283-5_13
168. Fries LF, Gordon DM, Richards RL, Egan JE, Hollingdale MR, Gross M, et al. Liposomal malaria vaccine in humans: a safe and potent adjuvant strategy. Proc Natl Acad Sci USA. (1992) 89:358–62. doi: 10.1073/pnas.89.1.358
169. Richards RL, Swartz GM, Schultz C, Hayre MD, Ward GS, Ballou WR, et al. Immunogenicity of liposomal malaria sporozoite antigen in monkeys: adjuvant effects of aluminium hydroxide and non-pyrogenic liposomal lipid A. Vaccine (1989) 7:506–12. doi: 10.1016/0264-410X(89)90274-0
170. Rutgers T, Gordon D, Gathoye AM, Hollingdale M, Hockmeyer W, Rosenberg M, et al. Hepatitis B surface antigen as carrier matrix for the repetitive epitope of the circumsporozoite protein of Plasmodium Falciparum. Nat Biotechnol. (1988) 6:1065–70. doi: 10.1038/nbt0988-1065
171. Gordon DM, McGovern TW, Krzych U, Cohen JC, Schneider I, LaChance R, et al. Safety, immunogenicity, and efficacy of a recombinantly produced Plasmodium falciparum circumsporozoite protein-hepatitis B surface antigen subunit vaccine. J Infect Dis. (1995) 171:1576–85. doi: 10.1093/infdis/171.6.1576
172. Ansong D, Asante KP, Vekemans J, Owusu SK, Owusu R, Brobby NA, et al. T cell responses to the RTS,S/AS01(E) and RTS,S/AS02(D) malaria candidate vaccines administered according to different schedules to Ghanaian children. PLoS ONE (2011) 6:e18891. doi: 10.1371/journal.pone.0018891
173. Owusu-Agyei S, Ansong D, Asante K, Kwarteng Owusu S, Owusu R, Wireko Brobby NA, et al. Randomized controlled trial of RTS,S/AS02D and RTS,S/AS01E malaria candidate vaccines given according to different schedules in Ghanaian children. PLoS ONE (2009) 4:e7302. doi: 10.1371/journal.pone.0007302
174. Kester KE, Cummings JF, Ofori-Anyinam O, Ockenhouse CF, Krzych U, Moris P, et al. Randomized, double-blind, phase 2a trial of falciparum malaria vaccines RTS,S/AS01B and RTS,S/AS02A in malaria-naive adults: safety, efficacy, and immunologic associates of protection. J Infect Dis. (2009) 200:337–46. doi: 10.1086/600120
175. Leroux-Roels G, Leroux-Roels I, Clement F, Ofori-Anyinam O, Lievens M, Jongert E, et al. Evaluation of the immune response to RTS,S/AS01 and RTS,S/AS02 adjuvanted vaccines: randomized, double-blind study in malaria-naive adults. Hum Vaccin Immunother (2014) 10:2211–9. doi: 10.4161/hv.29375
176. Olotu A, Fegan G, Wambua J, Nyangweso G, Leach A, Lievens M, et al. Seven-year efficacy of RTS,S/AS01 malaria vaccine among young african children. N Engl J Med. (2016) 374:2519–29. doi: 10.1056/NEJMoa1515257
177. Kazmin D, Nakaya HI, Lee EK, Johnson MJ, van der Most R., van den Berg RA, et al. Systems analysis of protective immune responses to RTS,S malaria vaccination in humans. Proc Natl Acad Sci USA. (2017) 114:2425–30. doi: 10.1073/pnas.1621489114
178. van den Berg RA, Coccia M, Ballou WR, Kester KE, Ockenhouse CF, Vekemans J, et al. Predicting RTS,S vaccine-mediated protection from transcriptomes in a malaria-challenge clinical trial. Front Immunol. (2017) 8:557. doi: 10.3389/fimmu.2017.00557
179. Good MF, Pombo D, Quakyi IA, Riley EM, Houghten RA, Menon A, et al. Human T-cell recognition of the circumsporozoite protein of Plasmodium falciparum: immunodominant T-cell domains map to the polymorphic regions of the molecule. Proc Natl Acad Sci USA. (1988) 85:1199–203. doi: 10.1073/pnas.85.4.1199
180. Neafsey DE, Juraska M, Bedford T, Benkeser D, Valim C, Griggs A, et al. Genetic Diversity and protective efficacy of the RTS,S/AS01 malaria vaccine. N Engl J Med. (2015) 373:2025–37. doi: 10.1056/NEJMoa1505819
181. Zevering Y, Khamboonruang C, Good MF. Natural amino acid polymorphisms of the circumsporozoite protein of Plasmodium falciparum abrogate specific human CD4+ T cell responsiveness. Eur J Immunol. (1994) 24:1418–25. doi: 10.1002/eji.1830240627
182. Collins KA, Snaith R, Cottingham MG, Gilbert SC, Hill AVS. Enhancing protective immunity to malaria with a highly immunogenic virus-like particle vaccine. Sci Rep. (2017) 7:46621. doi: 10.1038/srep46621
183. Ahmad M, Hirz M, Pichler H, Schwab H. Protein expression in Pichia pastoris: recent achievements and perspectives for heterologous protein production. Appl Microbiol Biotechnol. (2014) 98:5301–17. doi: 10.1007/s00253-014-5732-5
184. Tiwari S, Goyal AK, Mishra N, Khatri K, Vaidya B, Mehta A, et al. Development and characterization of novel carrier gel core liposomes based transmission blocking malaria vaccine. J Control Release (2009) 140:157–65. doi: 10.1016/j.jconrel.2009.08.004
185. Tiwari S, Goyal AK, Khatri K, Mishra N, Vyas SP. Gel core liposomes: an advanced carrier for improved vaccine delivery. J Microencapsul (2009) 26:75–82. doi: 10.1080/02652040802170897
186. Agger EM, Rosenkrands I, Hansen J, Brahimi K, Vandahl BS, Aagaard C, et al. Cationic liposomes formulated with synthetic mycobacterial cordfactor (CAF01): a versatile adjuvant for vaccines with different immunological requirements. PLoS ONE (2008) 3:e3116. doi: 10.1371/journal.pone.0003116
187. Tyagi RK, Garg NK, Jadon R, Sahu T, Katare OP, Dalai SK, et al. XElastic liposome-mediated transdermal immunisation enhanced the immunogenicity of P. falciparum surface antigen, MSP-119. Vaccine (2017) 33:4630–8. doi: 10.1016/j.vaccine.2015.06.054
Keywords: malaria, Plasmodium, immunity, adjuvant, liposomes, vaccine
Citation: Ssemaganda A, Giddam AK, Zaman M, Skwarczynski M, Toth I, Stanisic DI and Good MF (2019) Induction of Plasmodium-Specific Immune Responses Using Liposome-Based Vaccines. Front. Immunol. 10:135. doi: 10.3389/fimmu.2019.00135
Received: 19 September 2018; Accepted: 16 January 2019;
Published: 01 February 2019.
Edited by:
Ashraful Haque, QIMR Berghofer Medical Research Institute, AustraliaReviewed by:
Peter Timmerman, Pepscan, NetherlandsAdrian John Frederick Luty, Institut de Recherche Pour le Développement (IRD), France
Copyright © 2019 Ssemaganda, Giddam, Zaman, Skwarczynski, Toth, Stanisic and Good. This is an open-access article distributed under the terms of the Creative Commons Attribution License (CC BY). The use, distribution or reproduction in other forums is permitted, provided the original author(s) and the copyright owner(s) are credited and that the original publication in this journal is cited, in accordance with accepted academic practice. No use, distribution or reproduction is permitted which does not comply with these terms.
*Correspondence: Danielle I. Stanisic, ZC5zdGFuaXNpY0BncmlmZml0aC5lZHUuYXU=
Michael F. Good, bWljaGFlbC5nb29kQGdyaWZmaXRoLmVkdS5hdQ==
†Present Address: Ashwini Kumar Giddam, Q-Pharm, Royal Brisbane and Women's Hospital, Herston, QLD, Australia