- 1School of Chemical & Materials Engineering, National University of Sciences and Technology (NUST), Islamabad, Pakistan
- 2Department of Materials Science and Engineering, College of Engineering, Peking University, Beijing, China
- 3School of Materials and Energy, University of Electronic Science and Technology of China, Chengdu, China
- 4Department of Physics, University of Education, Faisalabad Campus, Faisalabad, Pakistan
- 5School of Chemical and Biomolecular Engineering, The University of Sydney, Sydney, NSW, Australia
Increased efforts have been devoted recently to develop high-energy-density supercapacitors (SC) without renouncing their power efficiency. Herein, a hierarchically mesoporous nanostructure of zinc-nickel-cobalt oxide (ZNCO) nanowires (NWs) is constructed by hierarchical aggregation of ZNCO nanoparticles. It is worth noting that cobalt and nickel rich lattice imparts higher charge storage capability by enhanced reversible Faradaic reaction while zinc provides structural stability and higher conductivity. Moreover, particulate nature of ZNCO NWs allows deep diffusion of electrolyte thus enabling reversible charge storage under higher current densities. The as-prepared ZNCO NWs exhibited excellent specific capacitance of 2082.21 F g−1 at the current density of 1 A g−1 with high stability up to 5,000 charge-discharge cycles. Further, the asymmetric SC device was assembled using ZNCO NWs (ZNCO NWs//MWCNTs) which exhibited high energy density of 37.89 Wh kg−1 and excellent capacitance retention up to 88.5% over 1,000 cycles. This work presents ways to construct multi-component high-energy-density materials for next-generation energy storage devices.
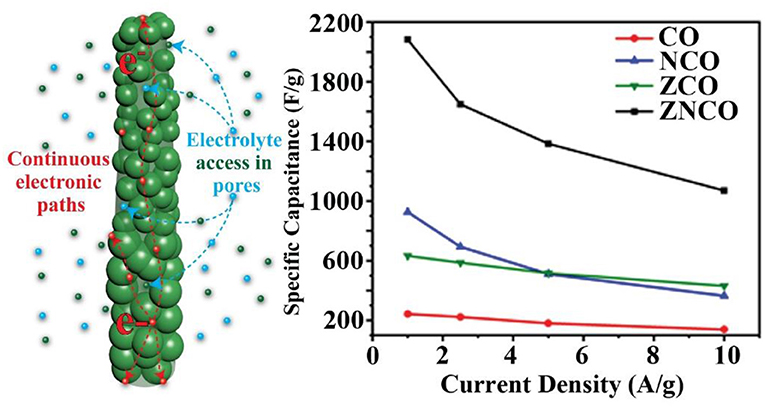
Graphical Abstract. The mixed metal oxides, having a combination of various transition metal-ion species have emerged as promising electrode materials owing to their higher electrochemical activities and relatively high electronic conductivities. Properly tuned nanostructures with hierarchical porosity provides opportunity to tailor high capacity mixed metal oxides.
Introduction
Supercapacitors (SCs) have attracted enormous interest due to high power density, fast charging/discharging and long service life and have found wide scale applications from portable electronic devices to large road markets (Wang et al., 2012; González et al., 2016; Han et al., 2018). However, the SCs generally exhibit lower energy densities (0-10 Wh Kg−1) which limit their applications at commercial scale (Pomerantseva and Gogotsi, 2017; Raza et al., 2018). The issue of relatively lower energy density has been addressed by enhancing either the capacitive performance i.e., increasing specific capacitance or by widening the operating voltage window (Xu et al., 2010; Kim et al., 2015). The specific capacitance and operating window are dependent upon the structure and properties of the constituent materials along with several other factors. Nanotechnology has opened up new horizons with the development of novel materials and Nanostructures. A number of electrode materials have been investigated for enhancing the capacitive performance of the SC devices including carbonaceous nanostructures, metallic species, metal/carbon hybrids etc (Mahmood et al., 2015, 2016; Tabassum et al., 2017, 2018; Jian et al., 2019). Among various electrode materials, the metal based materials have shown promising results owing to reversible Faradaic reactions happening on the metal electrode surface accounting for higher charge storage capability (Xu et al., 2010; Hu et al., 2017; Sun et al., 2018; Zhao et al., 2018; Chen and Lin, 2019; Chen et al., 2019). In particular, the mixed metal oxides, having a combination of various transition metal-ion species have emerged as promising electrode materials owing to their higher electrochemical activities and relatively high electronic conductivities. However, the large-scale applications of mixed metal oxides are limited by poor control over their inherent structure, composition and poor cyclic stability.
To improve the specific capacitance and cyclic stability of mixed metal oxides, the possibility of designing porous chemistries which can allow fast mass diffusion have been proposed recently. However, designing hierarchically porous chemistries to promote electrode/electrolyte interface with excellent control over composition of mixed metal oxides is highly challenging. The existence of meso- and micro- pores are generally considered to be beneficial for fast mass diffusion and improved electrode/electrolyte contact, respectively (Pan et al., 2006; Yuan et al., 2011; Wu et al., 2015; Fang et al., 2018; He et al., 2018; Sahoo et al., 2018). Moreover, the presence of various metallic species in crystal lattice provides a number of active sites for Faradaic redox reaction, enhances conductivity and offers the opportunity to operate under high current densities (Wu et al., 2015). Hence, designing mixed metal oxide nanostructures with excellent control over their structure is critical in order to realize the maximum specific capacitance with high cyclic life. Moreover, a good control over chemical composition is another challenge when tailoring the inherent structure of nanomaterials which needs to be addressed (Xiong et al., 2014; Lin et al., 2016; Maitra et al., 2017; Sahoo and Shim, 2017; Yan et al., 2017; Azman et al., 2018). Recently, trimetallic oxides have been proposed for SC applications owing to their enhanced electrochemical activities and longer stability (Zhao et al., 2015, 2016; Ma et al., 2016; Sun et al., 2018; Wang et al., 2018; Alqahtani et al., 2019). However, developing optimum nanostructure for trimetallic oxides with single phase product having optimum pore structure for enhanced and fast charge storage is highly challenging.
Herein, we report controlled synthesis of hierarchically mesoporous Zinc-Nickel-Cobalt oxide (Zn0.6Ni0.8Co1.6O4, ZNCO) nanowires (NWs) by a facile hydrothermal method. Keeping in mind the high specific activity of Co, the Zn and Ni species have been introduced into the Co rich lattice to obtain Zn0.6Ni0.8Co1.6O4 with needle-like NW structure formed by hierarchical aggregation of ZNCO nanoparticles. The mesopores arising from hierarchical hetero-aggregation of nanoparticles provided shorter diffusion pathways for electrolytes, thus enabling the reversible operation at higher charge/discharge rates. Owing to unique mesoporous structure, the developed material exhibited excellent capacitive performance in 6 M KOH electrolyte delivering specific capacitance of 2082.21 F g−1 at scan rate of 1 A g−1 with remarkable cyclic stability of 120% after 5,000 cycles. The asymmetric device assembled using ZNCO NWs as positive and MWCNTs as negative electrode exhibited a high specific capacitance of 121.268 F g−1 at 1 A g−1, superior energy density of 37.89 Wh kg−1 at the corresponding power density of 749.8 W kg−1 and excellent cyclic stability of 90% after 1,000 cycles. The exceptional electrochemical performance of ZNCO NWs, as well as its high performance as an asymmetric device with wide operating window, indicates that it is one of the most promising candidates for future energy storage devices.
Experimentation
Synthesis
Co(NO3)2·6H2O>99% was purchased from UNICHEM, Zn(NO3)2·6H2O>98%, Ni(NO3)2·6H2O >97% pure, CO(NH2)2>98% and NH4F were purchased from DAEJUNG, KOH was purchased from MERK, nafion@deflourinated resin solution and absolute ethanol 99.8% pure were purchased from Sigma Aldrich and all chemical were utilized without further purification. The ZNCO NWs were synthesized by the simple hydrothermal method. In first step 0.3 M zinc nitrate hexahydrate (Zn(NO3)2·6H2O), 0.3 M nickel nitrate hexahydrate (Ni(NO3)2·6H2O), 0.6 M cobalt nitrate hexahydrate (Co(NO3)2·6H2O), 1.2 M urea (Co(NH2)2) and 0.4 M ammonium fluoride (NH4F) were added to the 50 ml of distilled water with continuous stirring for 2 h. The homogeneous mixture was transferred into the 100 ml of Teflon lined stainless steel autoclave. The autoclave was kept in the oven at 130OC for 5 h. Then precipitates were washed several times with distilled water, and absolute ethanol and precipitates were filtered out by vacuum filtration. Afterwards, the sample was dried at 60°C for 6 h and then annealed at 350°C at heating rate of 2oC/min in a muffle furnace for 2 h to obtain the crystal structure of ZNCO NWs. For comparison, nickel cobalt oxide (NCO), zinc cobalt oxide (ZCO), nickel oxide (NO), cobalt oxide (CO) were prepared by the same process.
Characterization
FEI Tecnai F30 (transmission electron microscope, TEM) and field emission scanning electron microscope (MIRA3 TESCAN) were used for the morphological characterization of the product. Moreover, selected area selected area electron diffraction (SAED) and high resolution TEM (HRTEM) analysis was also carried out by FEI Tecnai F30. Elemental composition and oxidation states of the material was analyzed by Energy dispersive spectroscopy detector equipped in (MIRA3 TESCAN) and X-ray photoelectron spectroscopy (XPS), Kratos Axis Ultra with monochromatized Al Kα radiation (1486.6 eV). X-ray Diffraction Analysis (STOE diffractometer) was used to determine the crystal structure of ZNCO NWs. In order to find the surface area of ZNCO NWs surface area analyzer Micromeritics Gemini VII 2390 was utilized.
Electrochemical Analysis
Electrochemical properties of the synthesized materials were carried out on electrochemical work station. A three electrode setup was used where the sample was first prepared by adding catalyst material &10 μl nafion in ethanol and sonicated for 1 h then drop cast on glassy carbon electrode of 3 mm diameter having surface area of 7.065 × 10−6 m2 and dried at 60°C, Platinum (Pt) wire as a counter electrode (CE) and Ag/AgCl as a reference electrode (RE). Cyclic voltammetry (CV) was performed at 100, 50, 20, and 10 mV s−1 scan rate with operating potential window of −0.1 to 0.5 V. Galvanostatic charge/discharge (GCD) test was done at 1, 2.5, 5, and 10 A g−1. Electrochemical impedance spectroscopy was executed from 100 to 1 MHz. All electrochemical tests were performed in 6M KOH solution.
Specific capacitance was calculated by following equation
Here, “t” is discharge time, “I” represents current and “m” is mass of active material, ΔV is the voltage drop during discharge.
Electrochemical tests of asymmetric SC (ASC) were carried out by using two electrode assembly. Electrochemical cell was constructed using ZNCO NWs as positive electrode and MWCNTs as negative electrode. All the tests were performed at a wide operating window of 0 V to 1.5 V. Asymmetric SC performance was strongly influenced by the charge balance between the positive and negative electrodes. Following charge balance equation utilized to calculate the loading of mass on electrode is
Where m is the mass (g) of the electrodes. C is the specific capacitance (F g−1), and ΔV is the potential window. Optimized mass to charge ratio for this asymmetric cell is 0.5.
Results and Discussions
The hierarchically mesoporous ZNCO NWs were synthesized by a simple two-step methodology using hydrothermal treatment followed by calcination in air as depicted in Figure 1. The XRD analysis was performed to determine the crystal structure of ZNCO NWs and the results are presented in Figure 2a and XRD of MWCNTs in Figure S4. The analysis of pure Co3O4 revealed that diffraction peaks correspond well with standard card (JCPDS: 03-065-3103) and the crystal structure belongs to the cubic and space group of Fd-3m having (111), (220), (222), (400), (422), (511), (440) planes. The analysis of NCO (Ni doped Co3O4) and Zn/Ni (Zn/Ni codoped Co3O4) exhibited similar diffraction pattern to pure Co3O4 confirming successful addition of Ni and Zn into the crystal lattice of Co3O4 without any phase impurity. All diffraction peaks of doped samples correspond well with standard card (JCPDS: 03-065-3103) confirming the phase purity in the product. It is important to note that the diffraction peaks are slightly shifted toward higher angle in comparison to reference pattern which is due to the difference in the cations of the Zn, Ni and Co (Wu et al., 2018). The crystallite size of ZNCO NWs was calculated by using the Scherrer Formula and the average crystallite size of ZNCO NWs was found to be ~8.1 nm. The Field emission scanning electron microscope (FESEM) was utilized to investigate the morphology of the developed products and the results are presented in Figures 2b,c and Figure S5. The FESEM analysis revealed that the CO, NCO and ZNCO NWs are composed of homogeneous 3D needle-like structures (Figure 2b and Figures S1, S2). High magnification analysis revealed that the NWs consisted of an average diameter of ca. 18–20 nm (Figure 2c). The existence of 3D needle-like morphology ensures large surface area and enhanced electrode-electrolyte interface for better charge storage.
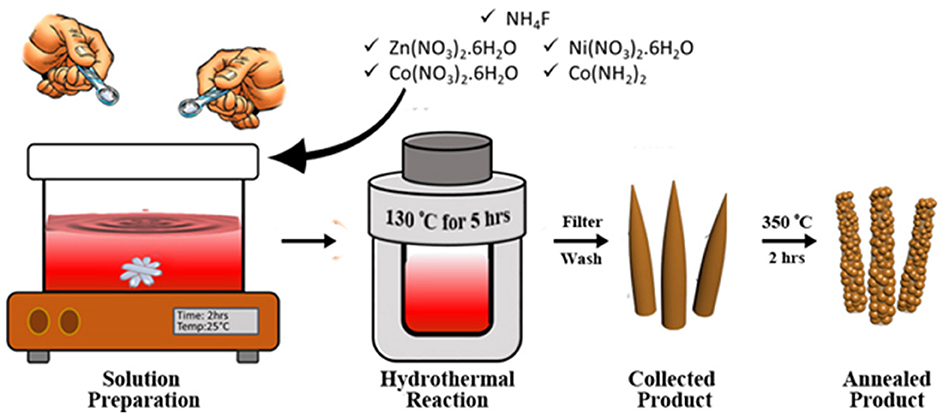
Figure 1. Schematic illustration of the synthesis process of ZNCO. A homogenous solution of precursors is prepared by magnetic stirring at room temperature. Hydrothermal reaction produces needle like structure which are then heat treated obtain hierarchically mesoporous ZNCO NWs.
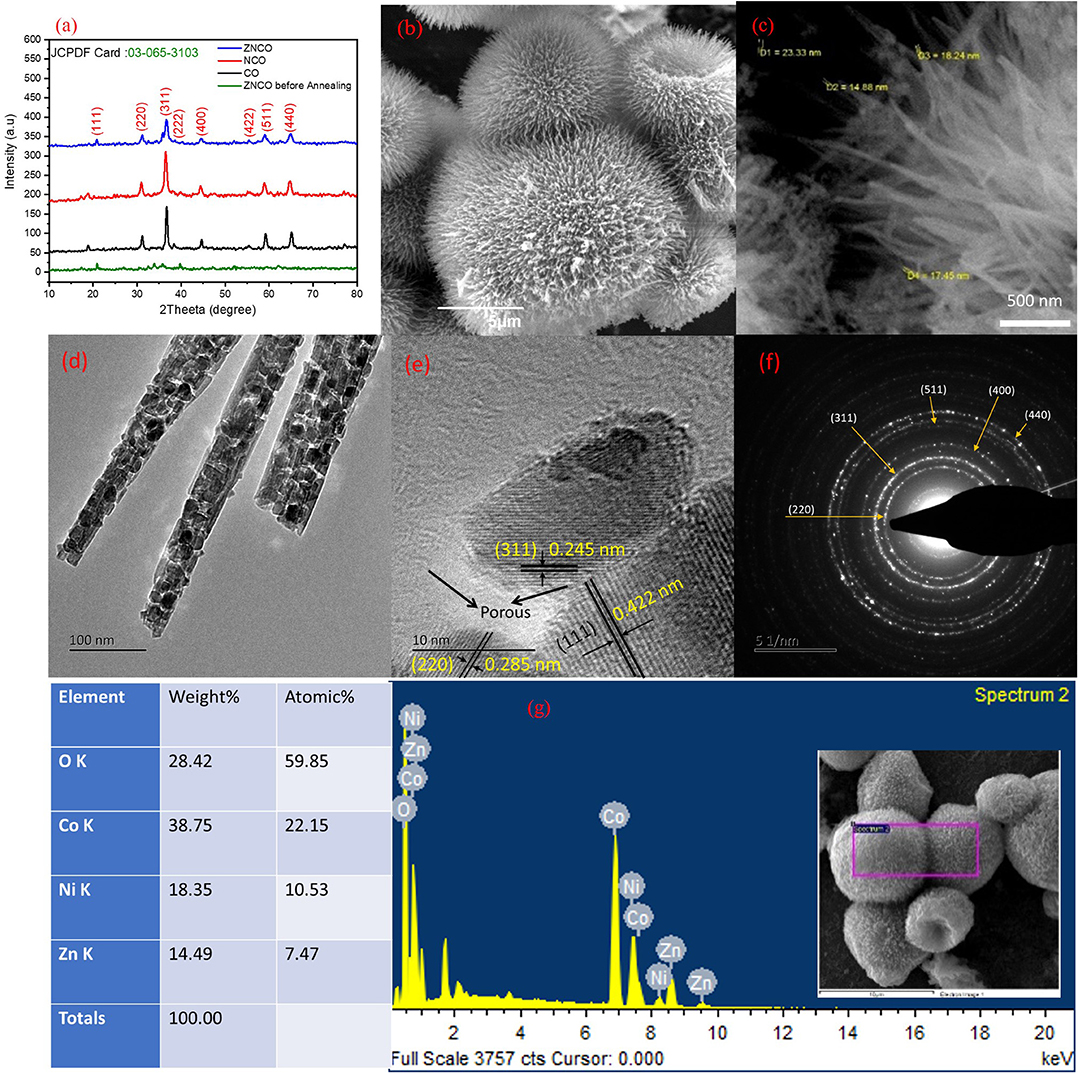
Figure 2. (a) XRD of Co, NCO, ZNCO and ZNCO before annealing (b) SEM of Hierarchically arranged ZNCO nanostructures (c) FESEM image of ZNCO NWs (d) TEM Image of ZNCO NWs (e) HRTEM of ZNCO crystal (f) SAED of ZNCO (g) EDS spectrum of ZNCO NWs.
To obtain more insights into the internal structure, the TEM analysis was carried out (Figure 2d). It is obvious from TEM results that the ZNCO NWs are formed by aggregation of crystalline particles. It can be assumed that the calcination at 350°C leads to growth of crystalline particles and removal of unreacted species which leaves various open spaces among particles and leads to the creation of porous region. This generation of pores not only ensures electrolyte infiltration leading to enhanced electrode/electrolyte interface but also guarantees fast mass diffusion whereby the developed electrode material could be utilized at higher charge/discharge rates. The high magnification analysis revealed existence of large pores in the NWs as shown in Figure 2e and Figure S10. Furthermore, the HRTEM analysis revealed interplanar d-spacing of 0.245, 0.422, and 0.285 nm corresponding to (311), (111) and (220) planes matched with the observed d-spacing of the XRD pattern (Figure 2e). It is worth mentioning that the crystallite size observed with HRTEM clearly matched with the size calculated from Scherrer Formula. The SAED pattern was recorded to gain insights into the crystal structure of ZNCO NWs and presented in Figure 2f. The SAED pattern affirmed the polycrystalline nature of ZNCO NWs with d-spacing of 0.285,0.249,0.207,0.158, and 0.146 nm referring to (220), (311), (400), (511) and (440) planes. The EDS analysis was used to estimate the elemental composition in ZNCO NWs, and the Zinc, Nickel, Cobalt, and oxygen were found to be in atomic weight percent of 7.47, 10.53, 22.15, 59.85, respectively (Figure 2g and Figure S11). The elemental composition further validates the existence of Zn0.6Ni0.8 Co1.6O4 phase in ZNCO NWs.
The XPS analysis was carried out to examine the detailed composition and oxidation state of ZNCO NWs. Low-resolution XPS survey verifies the presence of Zn, Ni, Co and O elements in a typical ZNCO NWs sample as shown in Figure S9. The Co 2p spectrum (Figure 3A) represents two broad peaks of Co 2p1/2 at high binding energy of 795.18 eV and Co 2p3/2 at low binding energy of 779.83 eV. The deconvolution of Co indicates the existence of Co2+ (in tetrahedral sites) and Co3+ (in octahedral sites) as well two shakeup satellites at 783.74 eV and 788 eV (Figure 3A) (Wang et al., 2018). Moreover, the spin-orbit splitting of 15.35 eV was observed for Co 2p1/2 and Co 2p3/2 indicating the coexistence of both Co2+/Co3+ (Chen et al., 2015). The deconvolution of Ni 2p spectrum indicates the presence of both Ni2+ and Ni3+ (Figure 3B; Wu et al., 2015; Sahoo and Shim, 2017). Similarly, the deconvolution of Zn exhibited two peaks of Zn 2p3/2 at 1020.5 eV and Zn 2p1/2 at 1043.5 eV in as shown in Figure 3C (Zhao et al., 2016). The deconvolution of O1s exhibited three oxygen metal bond peaks at binding energy of 529.1 eV, 531.1 eV and 531.9 eV correspond to zinc oxide, cobalt oxide, and nickel oxide, respectively, in Figure 3D (Bao et al., 2014; Ding et al., 2014; Bhagwan et al., 2019). All these results show that ZNCO NWs contain Co3+, Co2+, Ni3+, Ni2+, and Zn2+. The inherent porosity of the electrode materials plays key role in determining their respective charge storage capacity. The porosity of the developed products was investigated using Brunauer–Emmett–Teller (BET) analysis and presented in Figure 3E and Figure S7. Average pore diameter of ZNCO is 8.63 nm centered in Figures 3E,F. The ZNCO NWs exhibited pore volume of (0.447 cm3 g−1) and much higher surface area of 68.3066 m2 g−1 in comparison to CO (33.7236 m2 g−1) and ZCO (47.8949 m2 g−1). However, the ZNCO NWs surface area was slightly lower than NCO (75.0092 m2 g−1). The large specific area of ZNCO NWs results in the electrolyte transfer through the electrode material efficiently and increase the reactive sites which result in superior electrochemical performance.
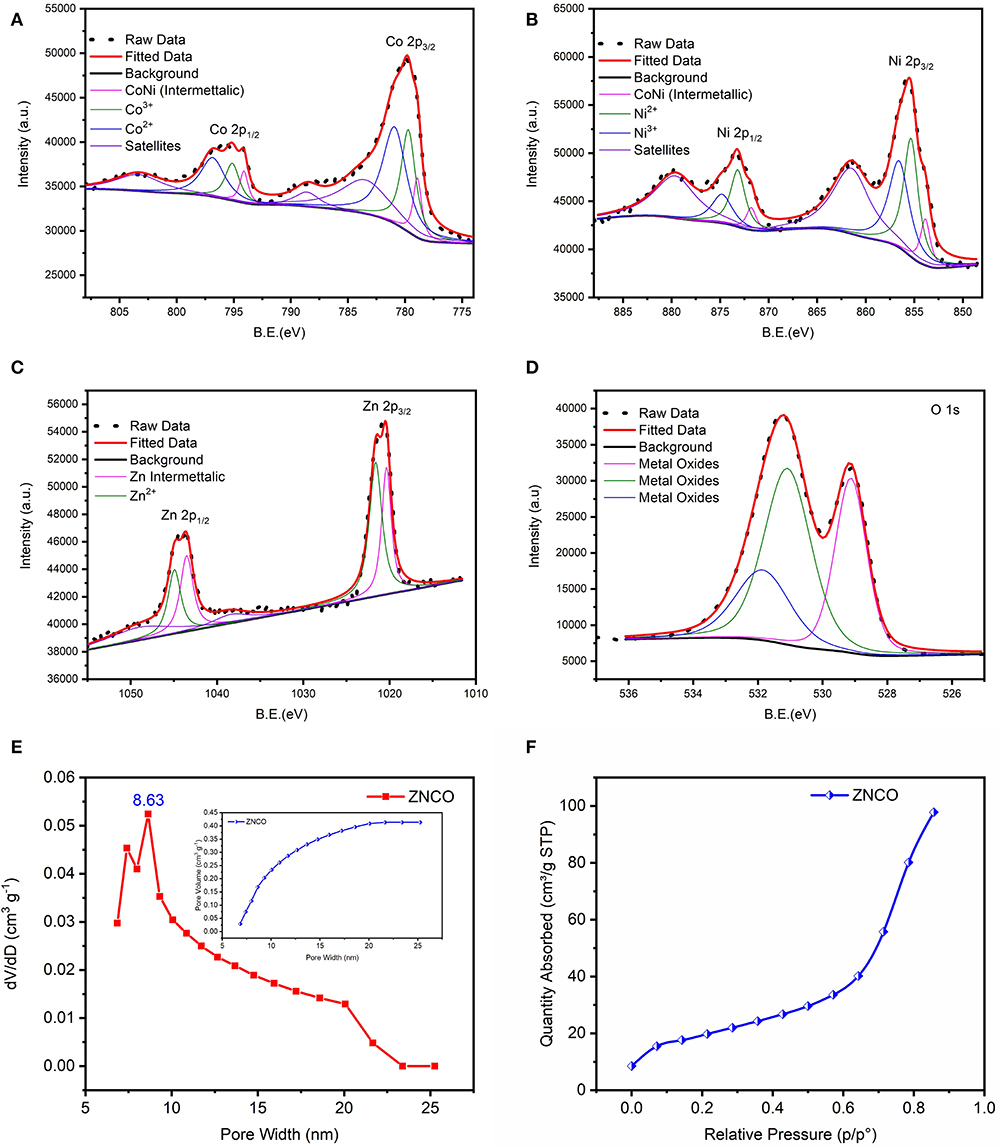
Figure 3. XPS spectra of ZNCO NWs (A) Co 2p (B) Ni 2p (C) Zn 2p (D) O 1s (E) BET pore volume and pore size distribution of ZNCO NWs (F) N2 absorption isotherm at 77 K of ZNCO NWs.
The designed ternary metal oxide NWs were studied as electrode for SC because of unique structural and compositional features. The ZNCO NWs are expected to exhibit more stability in comparison to CO and other counterparts because of the presence of structure stabilizing species in the form of Zn and Ni which not only stabilize the crystal lattice but also provide higher electronic conductivity. Highly porous nature of NWs provides large electrode/electrolyte interface while mesoporosity ensure presence of large channels for fast diffusion of electrolytes, thus enabling the operation under higher charge/discharge rate. Moreover, the small size of ZNCO nanoparticles (~15–20 nm) in the NW allows deep diffusion of electrolyte into the crystal lattice and ensures utilization of all active species for charge storage. The electrochemical performance was studied in 3-electrode system using ZNCO NWs as working electrode, Pt wire as counter and Ag/AgCl as reference electrode in 6 M KOH electrolyte solution. Figure 4A shows the CV analysis of various products in the voltage window of −0.1–0.5 V at the scan rate of 10 mV s−1. Among various products, the ZNCO NWs exhibited the highest area under CV curve and a sharp redox peak with strong oxidation (0.35 V) and reduction (0.22 V) as shown in Figure 4A. While the other products in comparison CO NCO and ZCO show less area under the curve as well as only oxidation or reduction peak. Both the oxidation and reduction phenomena in the ZNCO sample during CV testing leads toward the better electrochemical performance as compared to CO NCO ZCO samples. The electrochemical behavior of ZNCO NWs was further analyzed by CV analysis at different scan rates (10, 20, 50, and 100 mV s−1) and the results are presented in Figure 4B. Increasing the scan rate from 10 to 100 mV s−1 caused slight peak shift to higher potential and the peaks are not clearly visible as the limitation of diffusion reaction with the increase of scan rate and less redox reaction occurs as less diffusion occurs so the redox peaks become less prominent at higher scan rates.
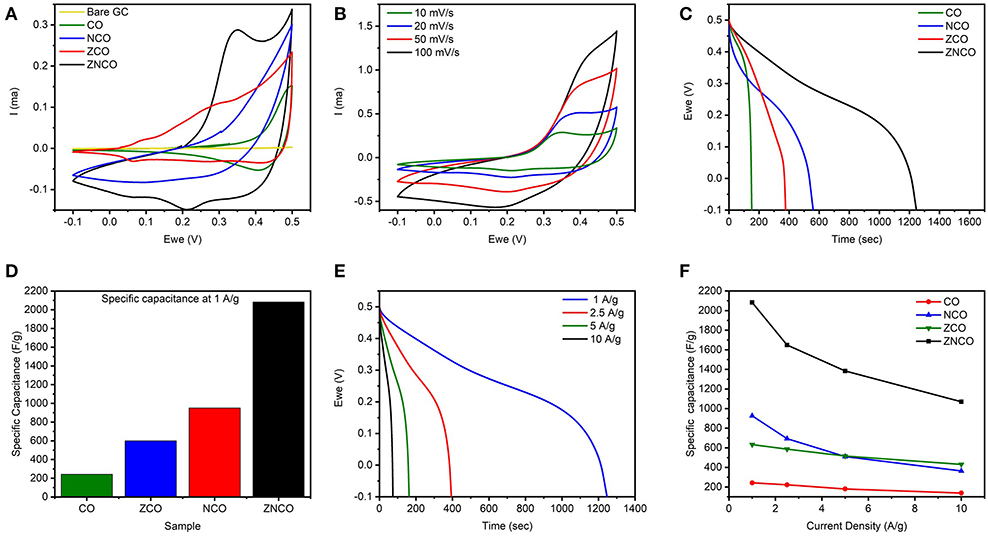
Figure 4. (A) CV Comparison of CO, NCO, ZCO, ZNCO and Bare glassy carbon at 10 mV s−1 (B) CV curves of ZNCO nanostructures at different scan rates (C) Discharge curves comparison of CO, NCO, ZCO, ZNCO at 1 A g−1 (D) Specific Capacitance comparison of CO, ZCO, NCO and ZNCO at 1 A g−1 (E) GCD cycles of ZNCO nanostructures at different current densities (F) Specific capacitance of CO, ZCO, NCO and ZNCO at different current densities.
To investigate actual capacitance values, the Galvanostatic charge-discharge (GCD) analysis was carried out to gain more insights into the performance of the electrode materials. As shown in Figure 4C, all products exhibit a clear plateau in the charge/discharge profile clearly indicating the undergoing redox reaction at current density of 1 A g−1. However, ZNCO NWs exhibits longest discharge time indicating high charge storage capacity in comparison to CO, ZCO and NCO which is consistent with CV analysis. Figure 4D represents the comparison of capacitive performance of ZNCO NWs with CO, ZCO and NCO. It is quite clear that ZNCO NWs exhibits much larger capacitance of 2082.21 F g−1 at a current density of 1 A g−1 as compared to the capacitive performances arising from CO (242.88 F g−1), ZCO (633.06 F g−1) and NCO (925.7 F g−1) as summarized in Table S2. The higher performance for ZNCO NWs can be attributed to relatively larger number of active sites for Faradaic redox reaction, larger surface area and particulate morphology which allows deep diffusion of electrolyte thus enabling the active sites deep inside the particle to participate in the charge storage process. The rate capability of the ZNCO NWs was further studied at various current densities and the results are presented in Figures 4E,F and Table S3. Rate capability of CO,NCO and ZCO are presented in Figures S3d–f. It is interesting to note that the charge-discharge curves retain non-linear nature even at high current densities of 10 A g−1. The presence of well-defined plateau at higher current densities clearly indicate the reversible Faradaic redox reaction even at much higher discharge rates. Owing to highly reversible Faradaic redox reaction at higher current densities, the ZNCO NWs exhibited excellent capacitance retention of 1648.37, 1363.86, 1070 F g−1 at 2.5, 5, and 10 A g−1 as shown in Figure 4F.
The cyclic stability of developed electrode material was analyzed GCD cycles and the results are presented in Figure 5A and Figure S8. The ZNCO NWs exhibited excellent cyclic stability for over 5,000 charge/discharge cycles retaining over 120 % of initial capacitance at current density of 50 Ag−1. It is important to note that the capacitance increased for the first cycles because of activation of electrode materials whereby the extremely high current density limited the quick activation. Moreover, the Columbic Efficiency was almost ~100% after 5,000 cycles which shows excellent correlation between charge/discharge which is clearly evident from inset of Figure 5A. The EIS analysis was performed to evaluate the charge transfer resistance (Rct) as well as the series resistance (Rs). The ZNCO NWs exhibited lowest Rct value of 17.61 Ω as compared to ZCO, NCO, CO as shown in Figure 5B and Table S1. Relatively lower Rct values for ZNCO NWs clearly indicated that the ZNCO NWs is more conductive in comparison to other counterparts. The Rct values increased after cycling probably due to slighly change in morphology of material during the cyclic performance at high current density of 50 A g−1 as shown in Figure 5C. Furthermore, the diffusion coefficient of K+ ions (Dk) was calculated by using low frequency region of Nyquist plot (Mahmood et al., 2019).
Where T is the absolute temperature, R is the ideal gas constant, A is the surface area of electrode, n is number of electrons, F is faradays constant, C is the concentration of ions and σ is the Warburg coefficient. σ was calculated from linear plot of Z ' and ω−1/2 in Figure 5D. ZNCO NWs exhibits Dk of 1.04 × 10−11 which indicates enhanced diffusion of electrolyte ions through electrode materials. However, the electrolyte diffusion decreased slightly (4.99 × 10−12) after 5,000 cycles probably due to aggregation of particles upon cycling.
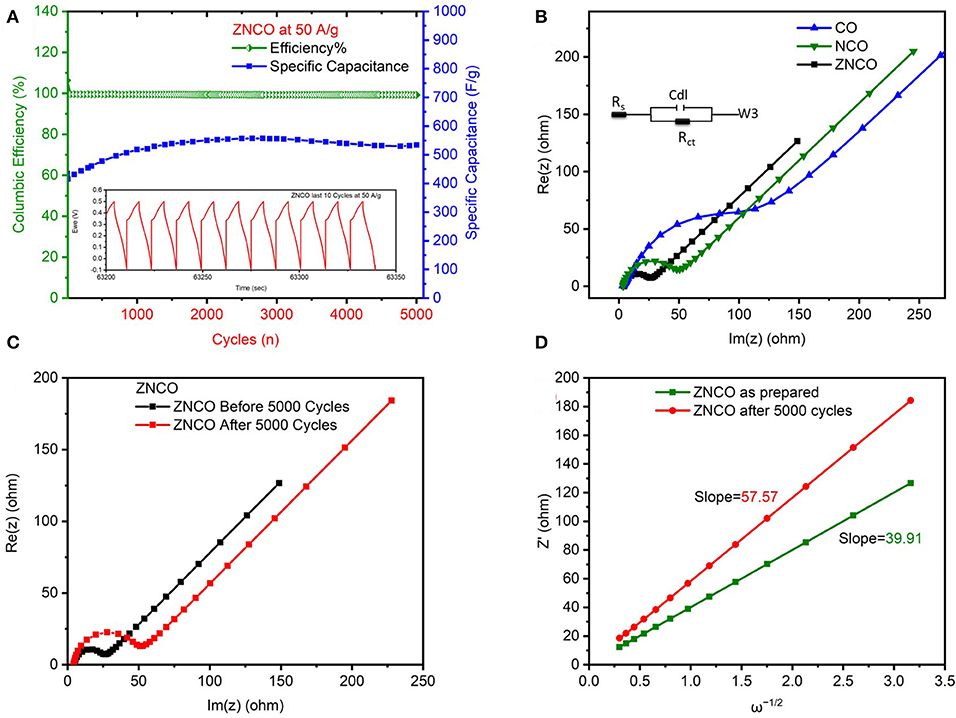
Figure 5. (A) Cyclic Stability and columbic efficiency of ZNCO nanostructure at 50 A g−1 (B) Nyquist plot of ZNCO, CO, NCO (C) Nyquist plot of ZNCO before and after cyclic performance (D) linear fits of the Z′ vs. ω−1/2.
To evaluate the performance of ZNCO NWs in SC device, the aqueous ASC was assembled using ZNCO NWs as positive electrode due to its high capacitance and MWCNTs as negative electrode due to their high power density. To obtain wide voltage window of 1.5 V, the operating voltage window of MWCNTs was adjusted to −1.0−0.1 V while the operating voltage window of ZNCO NWs was tested in the voltage window of −0.1–0.5 V as shown in Figure 6A. Moreover, prior to ASC testing, the capacitive performance of MWCNTs was investigated and presented in Figure S6. The CV and GCD analysis was used to investigate the capacitive performance of the assembled ASC (ZNCO NWs//MWCNTs) device and presented in Figures 6B,C. The CV analysis at different scan rates did not show any significant distortions clearly indicating good rate capability of the assembled device (Figure 6B). Moreover, the GCD show symmetric charge/discharge profile indicating ideal capacitive behavior. The ASC exhibited high specific capacitance of 121.26 F g−1 at 1 A g−1 and retained up to 85.2 F g−1 at 3.3 A g−1 in Figure 6D and Table S4. In addition, the assembled device exhibited high stability up to 1,000 cycles at current density of 10 A g−1 as shown in Figure 6E. An increase in capacitance was observed during first few cycles due to gradually improved wettability of the electrode materials which induce the participation of more active sites for charge storage. The ZNCO NWs//MWCNTs exhibited an overall 90 % capacitance retention after 1,000 charge/discharge cycles which clearly shows excellent stability of ZNCO NWs when applied in device as well. A slight loss of capacity can be ascribed to partial agglomeration of particles upon reversible cycling.
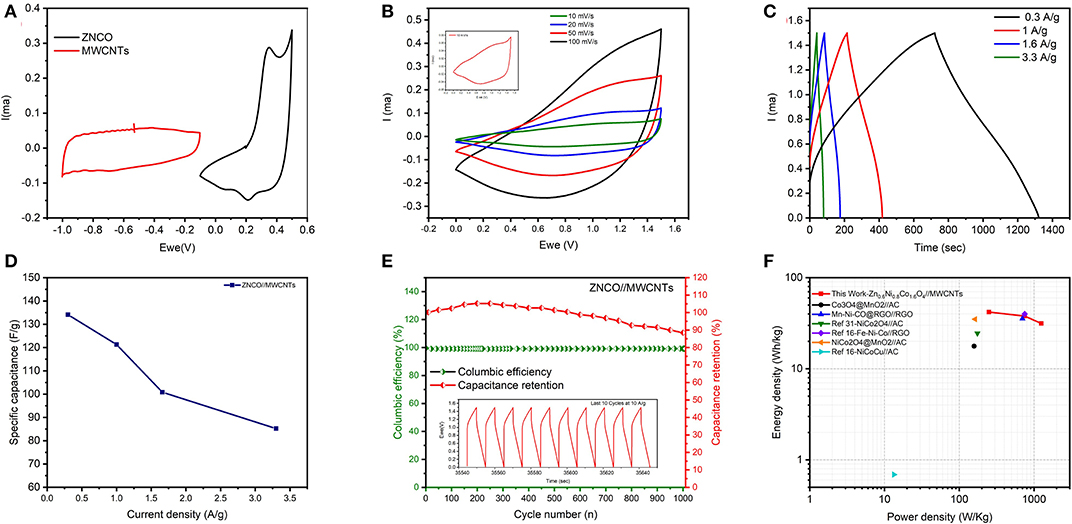
Figure 6. (A) CV curves of MWCNTs and ZNCO nanostructures at 10 mV s−1 (B) CV curves of ZNCO/MWCNTs ASC at different scan rates (C) Charge-discharge curves of ZNCO//MWCNTs asymmetric device at different current densities (D) specific capacitance of ZNCO//MWCNTs (E) Cyclic performance of ZNCO/MWCNTs at 10 A g−1 (F) Ragone plot of ZNCO//MWCNTs (this work), Co3O4@MnO2//AC (Huang et al., 2014), Mn-Ni-CO@RGO//RGO (Wu et al., 2017), NiCo2O4//AC (Zhong et al., 2012), Fe-Ni-Co//RGO (Sahoo et al., 2018), NiCo2O4@MnO2//AC (Xu et al., 2014), NiCoCu//AC (Sun et al., 2018).
The energy and power density are important parameters in determining the application of ASC device. The ASC devices generally exhibit much lower energy densities (~0.1–10 Wh kg−1) which must be improved to realize high performance ASCs. The ZNCO NWs//MWCNTs ASC device exhibited high energy density of 37.89 Wh kg−1 at a corresponding power density of 749.8 W kg−1 which is higher/comparable to the reported literature as shown in Figure 6F. At a high-power density of 1249.94 W kg−1, the ASC device could retain up to 31.5 Wh kg−1 as seen in Ragone plot in Figure 6F. The higher energy density with corresponding higher power densities can be attributed to hierarchically porous structure of ZNCO NWs which allows large electrode/electrolyte interface and fast mass transport enabling operation at higher current densities and presence of more than one metallic sites whereby Zn and Ni not only stabilize the structure but also impart higher conductivity and decrease the overall cost of the product.
Conclusions
In summary, the hierarchical mesoporous ZNCO NWs were successfully synthesized using two-step methodology (i.e., wet-chemical synthesis followed by high temperature calcination). As prepared ZNCO NWs were composed of small nanoparticles whereby hierarchical aggregation generated mesoporosity in the product. Owing to this mesoporous nature, the ZNCO NWs exhibited high surface area of 68.3066 m2 g−1. The ZNCO NWs exhibited outstanding electrochemical performance with a specific capacitance of 2082.21 F g−1 at 1 A g−1 with remarkable cyclic life of 5,000 cycles with capacitance retention of 120% owing to ternary metal oxide and hierarchically mesoporous structure. The asymmetric ASC device of ZNCO NWs//MWCNTs exhibited high specific capacitance of 121.26 F g−1 at 1 A g−1 as well as high energy density of 37.89 Wh kg−1. Superior electrochemical performance of ZNCO NWs//MWCNTs illustrates that the developed material provide opportunity to tailor new materials to meet the modern-day energy applications demand and the presented example could be one of the desired materials for next generation electrochemical devices.
Data Availability Statement
All datasets generated for this study are included in the article/Supplementary Material.
Author Contributions
The manuscript was written through contributions of all authors. All authors have given approval to the final version of the manuscript.
Funding
This work was supported by Higher Education Commission of Pakistan research grant NRPU#9998.
Conflict of Interest
The authors declare that the research was conducted in the absence of any commercial or financial relationships that could be construed as a potential conflict of interest.
Acknowledgments
The authors would like to acknowledge the technical support provided by The University of Sydney for successful completion of this work.
Supplementary Material
The Supplementary Material for this article can be found online at: https://www.frontiersin.org/articles/10.3389/fchem.2020.00487/full#supplementary-material
References
Alqahtani, D. M., Zequine, C., Ranaweera, C. K., Siam, K., Kahol, P. K., Poudel, T. P., et al. (2019). Effect of metal ion substitution on electrochemical properties of cobalt oxide. J. Alloys Comp. 771, 951–959. doi: 10.1016/j.jallcom.2018.09.014
Azman, N. H. N., Mamat Mat Nazir, M. S., Ngee, L. H., and Sulaiman, Y. (2018). Graphene-based ternary composites for supercapacitors. Int. J. Energy Res. 42, 2104–2116. doi: 10.1002/er.4001
Bao, F., Wang, X., Zhao, X., Wang, Y., Ji, Y., Zhang, H., et al. (2014). Controlled growth of mesoporous ZnCo2O4 nanosheet arrays on Ni foam as high-rate electrodes for supercapacitors. RSC Adv. 4, 2393–2397. doi: 10.1039/C3RA46439C
Bhagwan, J., Nagaraju, G., Ramulu, B., Sekhar, S. C., and Yu, J. S. (2019). Rapid synthesis of hexagonal NiCo2O4 nanostructures for high-performance asymmetric supercapacitors. Electrochim. Acta 299, 509–517. doi: 10.1016/j.electacta.2018.12.174
Chen, H., Zhang, Q., Han, X., Cai, J., Liu, M., Yang, Y., et al. (2015). 3D hierarchically porous zinc–nickel–cobalt oxide nanosheets grown on Ni foam as binder-free electrodes for electrochemical energy storage. J. Mater. Chem. A 3, 24022–24032. doi: 10.1039/C5TA07258A
Chen, M., Ge, Q., Qi, M., Liang, X., Wang, F., and Chen, Q. (2019). Cobalt oxides nanorods arrays as advanced electrode for high performance supercapacitor. Surf. Coat. Technol. 360, 73–77. doi: 10.1016/j.surfcoat.2018.12.128
Chen, T.-Y., and Lin, L.-Y. (2019). Morphology variation for the nickel cobalt molybdenum copper oxide with different metal ratios and their application on energy storage. Electrochim. Acta 298, 745–755. doi: 10.1016/j.electacta.2018.12.157
Ding, R., Qi, L., Jia, M., and Wang, H. (2014). Facile synthesis of mesoporous spinel NiCo 2 O 4 nanostructures as highly efficient electrocatalysts for urea electro-oxidation. Nanoscale 6, 1369–1376. doi: 10.1039/C3NR05359H
Fang, L., Wang, F., Zhai, T., Qiu, Y., Lan, M., Huang, K., et al. (2018). Hierarchical CoMoO4 nanoneedle electrodes for advanced supercapacitors and electrocatalytic oxygen evolution. Electrochim. Acta 259, 552–558. doi: 10.1016/j.electacta.2017.11.012
González, A., Goikolea, E., Barrena, J. A., and Mysyk, R. (2016). Review on supercapacitors: technologies and materials. Renew. Sust. Energy Rev. 58, 1189–1206. doi: 10.1016/j.rser.2015.12.249
Han, Y., Ge, Y., Chao, Y., Wang, C., and Wallace, G. G. (2018). Recent progress in 2D materials for flexible supercapacitors. J. Energy Chem. 27, 57–72. doi: 10.1016/j.jechem.2017.10.033
He, X., Li, R., Liu, J., Liu, Q., Chen, R., Song, D., et al. (2018). Hierarchical FeCo2O4@NiCo layered double hydroxide core/shell nanowires for high performance flexible all-solid-state asymmetric supercapacitors. Chem. Eng. J. 334, 1573–1583. doi: 10.1016/j.cej.2017.11.089
Hu, W., Wei, H., She, Y., Tang, X., Zhou, M., Zang, Z., et al. (2017). Flower-like nickel-zinc-cobalt mixed metal oxide nanowire arrays for electrochemical capacitor applications. J. Alloys Comp. 708, 146–153. doi: 10.1016/j.jallcom.2017.02.301
Huang, M., Zhang, Y., Li, F., Zhang, L., Wen, Z., and Liu, Q. (2014). Facile synthesis of hierarchical Co3O4@MnO2 core–shell arrays on Ni foam for asymmetric supercapacitors. J. Power Sources 252, 98–106. doi: 10.1016/j.jpowsour.2013.12.030
Jian, X., Wang, H., Rao, G., Jiang, L., Wang, H., Subramaniyam, C. M., et al. (2019). Self-tunable ultrathin carbon nanocups as the electrode material of sodium-ion batteries with unprecedented capacity and stability. Chem. Eng. J. 364, 578–588. doi: 10.1016/j.cej.2019.02.003
Kim, B. K., Sy, S., Yu, A., and Zhang, J. (2015). “Electrochemical supercapacitors for energy storage and conversion,” in Handbook of Clean Energy Systems, ed J. Yan (Chichester: John Wiley & Sons, Ltd), 1–25. doi: 10.1002/9781118991978.hces112
Lin, H., Huang, Q., Wang, J., Jiang, J., Liu, F., Chen, Y., et al. (2016). Self-assembled graphene/polyaniline/Co3O4 ternary hybrid aerogels for supercapacitors. Electrochim. Acta 191, 444–451. doi: 10.1016/j.electacta.2015.12.143
Ma, H., He, J., Xiong, D.-B., Wu, J., Li, Q., Dravid, V., et al. (2016). Nickel cobalt hydroxide @reduced graphene oxide hybrid nanolayers for high performance asymmetric supercapacitors with remarkable cycling stability. ACS Appl. Mater. Interfaces 8, 1992–2000. doi: 10.1021/acsami.5b10280
Mahmood, A., Li, S., Ali, Z., Tabassum, H., Zhu, B., Liang, Z., et al. (2019). Ultrafast sodium/potassium-Ion intercalation into hierarchically porous thin carbon shells. Adv. Mater. Weinheim. 31:1805430. doi: 10.1002/adma.201805430
Mahmood, A., Zou, R., Wang, Q., Xia, W., Tabassum, H., Qiu, B., et al. (2016). Nanostructured electrode materials derived from metal–organic framework xerogels for high-energy-density asymmetric supercapacitor. ACS Appl. Mater. Interfaces 8, 2148–2157. doi: 10.1021/acsami.5b10725
Mahmood, N., Tahir, M., Mahmood, A., Zhu, J., Cao, C., and Hou, Y. (2015). Chlorine-doped carbonated cobalt hydroxide for supercapacitors with enormously high pseudocapacitive performance and energy density. Nano Energy 11, 267–276. doi: 10.1016/j.nanoen.2014.11.015
Maitra, A., Das, A. K., Bera, R., Karan, S. K., Paria, S., Si, S. K., et al. (2017). An approach to fabricate PDMS encapsulated all-solid-state advanced asymmetric supercapacitor device with vertically aligned hierarchical Zn–Fe–Co ternary oxide nanowire and nitrogen doped graphene nanosheet for high power device applications. ACS Appl. Mater. Interfaces 9, 5947–5958. doi: 10.1021/acsami.6b13259
Pan, L. J., Pu, L., Shi, Y., Sun, T., Zhang, R., and Zheng, Y. O. (2006). Hydrothermal synthesis of polyaniline mesostructures. Adv. Funct. Mater. 16, 1279–1288. doi: 10.1002/adfm.200500543
Pomerantseva, E., and Gogotsi, Y. (2017). Two-dimensional heterostructures for energy storage. Nat. Energy 2:17089. doi: 10.1038/nenergy.2017.89
Raza, W., Ali, F., Raza, N., Luo, Y., Kim, K.-H., Yang, J., et al. (2018). Recent advancements in supercapacitor technology. Nano Energy 52, 441–473. doi: 10.1016/j.nanoen.2018.08.013
Sahoo, S., Nguyen, T. T., and Shim, J.-J. (2018). Mesoporous Fe–Ni–Co ternary oxide nanoflake arrays on ni foam for high-performance supercapacitor applications. J. Ind. Eng. Chem. 63, 181–190. doi: 10.1016/j.jiec.2018.02.014
Sahoo, S., and Shim, J.-J. (2017). Facile synthesis of 3D ternary ZnCo2O4/reduced graphene oxide/NiO composite film on nickel foam for next generation supercapacitor electrodes. ACS Sust. Chem. Eng. 5, 241–251. doi: 10.1021/acssuschemeng.6b01367
Sun, H.-Y., Lin, L.-Y., Huang, Y.-Y., and Hong, W.-L. (2018). Nickel precursor-free synthesis of nickel cobalt-based ternary metal oxides for asymmetric supercapacitors. Electrochim. Acta 281, 692–699. doi: 10.1016/j.electacta.2018.06.017
Tabassum, H., Mahmood, A., Wang, Q., Xia, W., Liang, Z., Qiu, B., et al. (2017). Hierarchical cobalt hydroxide and B/N Co-doped graphene nanohybrids derived from metal-organic frameworks for high energy density asymmetric supercapacitors. Sci. Rep. 7:43084. doi: 10.1038/srep43084
Tabassum, H., Qu, C., Cai, K., Aftab, W., Liang, Z., Qiu, T., et al. (2018). Large-scale fabrication of BCN nanotube architecture entangled on a three-dimensional carbon skeleton for energy storage. J. Mater. Chem. A 6, 21225–21230. doi: 10.1039/C8TA08590K
Wang, G., Zhang, L., and Zhang, J. (2012). A review of electrode materials for electrochemical supercapacitors. Chem. Soc. Rev. 41, 797–828. doi: 10.1039/C1CS15060J
Wang, M., Zhao, Y., Zhang, X., Qi, R., Shi, S., Li, Z., et al. (2018). Interface-rich core-shell ammonium nickel cobalt phosphate for high-performance aqueous hybrid energy storage device without a depressed power density. Electrochimica Acta 272, 184–191. doi: 10.1016/j.electacta.2018.04.005
Wu, C., Cai, J., Zhang, Q., Zhou, X., Zhu, Y., Shen, P. K., et al. (2015). Hierarchical mesoporous zinc–nickel–cobalt ternary oxide nanowire arrays on nickel foam as high-performance electrodes for supercapacitors. ACS Appl. Mater. Interfaces 7, 26512–26521. doi: 10.1021/acsami.5b07607
Wu, C., Cai, J., Zhu, Y., and Zhang, K. (2017). Hybrid reduced graphene oxide nanosheet supported Mn–Ni–Co ternary oxides for aqueous asymmetric supercapacitors. ACS Appl. Mater. Interfaces 9, 19114–19123. doi: 10.1021/acsami.7b03709
Wu, C., Chen, L., Lou, X., Ding, M., and Jia, C. (2018). Fabrication of cobalt-nickel-zinc ternary oxide nanosheet and applications for supercapacitor electrode. Front. Chem. 6:597. doi: 10.3389/fchem.2018.00597
Xiong, P., Huang, H., and Wang, X. (2014). Design and synthesis of ternary cobalt ferrite/graphene/polyaniline hierarchical nanocomposites for high-performance supercapacitors. J. Power Sources 245, 937–946. doi: 10.1016/j.jpowsour.2013.07.064
Xu, J., Gao, L., Cao, J., Wang, W., and Chen, Z. (2010). Preparation and electrochemical capacitance of cobalt oxide (Co3O4) nanotubes as supercapacitor material. Electrochim. Acta 56, 732–736. doi: 10.1016/j.electacta.2010.09.092
Xu, K., Li, W., Liu, Q., Li, B., Liu, X., An, L., et al. (2014). Hierarchical mesoporous NiCo2O4@MnO2 core–shell nanowire arrays on nickel foam for aqueous asymmetric supercapacitors. J. Mater. Chem. A 2:4795. doi: 10.1039/c3ta14647b
Yan, K.-L., Shang, X., Li, Z., Dong, B., Li, X., Gao, W.-K., et al. (2017). Ternary mixed metal fe-doped NiCo2O4 nanowires as efficient electrocatalysts for oxygen evolution reaction. Appl. Surf. Sci. 416, 371–378. doi: 10.1016/j.apsusc.2017.04.204
Yuan, Y. F., Xia, X. H., Wu, J. B., Huang, X. H., Pei, Y. B., Yang, J. L., et al. (2011). Hierarchically porous Co3O4 film with mesoporous walls prepared via liquid crystalline template for supercapacitor application. Electrochem. Commun. 13, 1123–1126. doi: 10.1016/j.elecom.2011.07.012
Zhao, J., Li, C., Zhang, Q., Zhang, J., Wang, X., Sun, J., et al. (2018). Hierarchical ferric-cobalt-nickel ternary oxide nanowire arrays supported on graphene fibers as high-performance electrodes for flexible asymmetric supercapacitors. Nano Res. 11, 1775–1786. doi: 10.1007/s12274-017-1795-9
Zhao, Y., Ma, H., Huang, S., Zhang, X., Xia, M., Tang, Y., et al. (2016). Monolayer nickel cobalt hydroxyl carbonate for high performance all-solid-state asymmetric supercapacitors. ACS Appl. Mater. Interfaces 8, 22997–23005. doi: 10.1021/acsami.6b05496
Zhao, Y., Zhang, X., He, J., Zhang, L., Xia, M., and Gao, F. (2015). Morphology controlled synthesis of nickel cobalt oxide for supercapacitor application with enhanced cycling stability. Electrochimica Acta 174, 51–56. doi: 10.1016/j.electacta.2015.05.162
Keywords: zinc-nickel-cobalt oxide, hierarchically porous nanostructures, SCs, aqueous asymmetric supercapacitors, high energy density
Citation: Ahsan MT, Usman M, Ali Z, Javed S, Ali R, Farooq MU, Akram MA and Mahmood A (2020) 3D Hierarchically Mesoporous Zinc-Nickel-Cobalt Ternary Oxide (Zn0.6Ni0.8Co1.6O4) Nanowires for High-Performance Asymmetric Supercapacitors. Front. Chem. 8:487. doi: 10.3389/fchem.2020.00487
Received: 28 September 2019; Accepted: 11 May 2020;
Published: 15 June 2020.
Edited by:
Elizabeth J. Podlaha, Clarkson University, United StatesReviewed by:
Akihiro Kushima, University of Central Florida, United StatesYanping Cui, China University of Geosciences Wuhan, China
Copyright © 2020 Ahsan, Usman, Ali, Javed, Ali, Farooq, Akram and Mahmood. This is an open-access article distributed under the terms of the Creative Commons Attribution License (CC BY). The use, distribution or reproduction in other forums is permitted, provided the original author(s) and the copyright owner(s) are credited and that the original publication in this journal is cited, in accordance with accepted academic practice. No use, distribution or reproduction is permitted which does not comply with these terms.
*Correspondence: Muhammad Aftab Akram, YWZ0YWJha3JhbSYjeDAwMDQwO3NjbWUubnVzdC5lZHUucGs=; Asif Mahmood, YXNpZi5tYWhtb29kJiN4MDAwNDA7c3lkbmV5LmVkdS5hdQ==