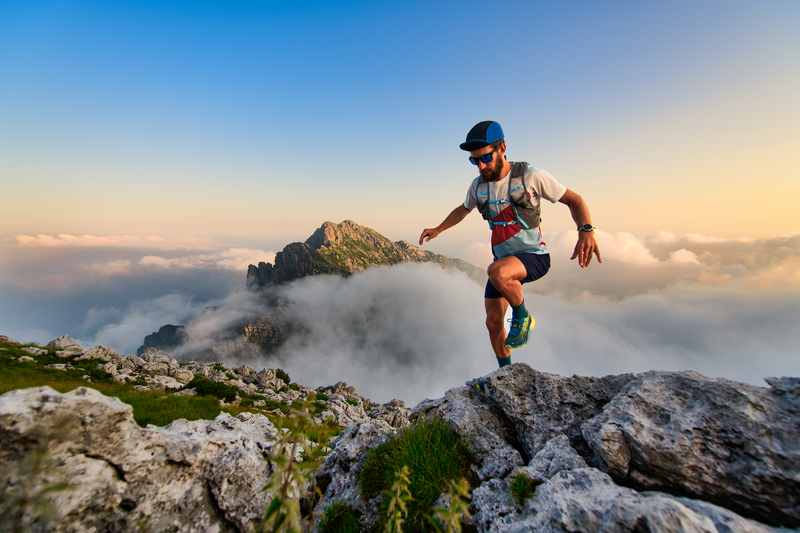
94% of researchers rate our articles as excellent or good
Learn more about the work of our research integrity team to safeguard the quality of each article we publish.
Find out more
ORIGINAL RESEARCH article
Front. Chem. , 02 October 2019
Sec. Chemical Biology
Volume 7 - 2019 | https://doi.org/10.3389/fchem.2019.00666
This article is part of the Research Topic Addressing Roles for Glycans in Immunology using Chemical Biology View all 15 articles
Multivalent structures displaying different instead of similar sugar units, namely heteroglycoclusters (hGCs), are stimulating the efforts of glycochemists for developing compounds with new biological properties. Here we report a four-step strategy to synthesize hexadecavalent hGCs displaying eight copies of αFuc and βGal. These compounds were tested for the binding to lectins LecA and LecB from Pseudomonas aeruginosa. While parent fucosylated (19) and galactosylated (20) homoclusters present nanomolar affinity with LecB and LecA, respectively, we observed that hGCs combining these sugars (11 and 13) maintain their binding potency with both lectins despite the presence of an unspecific sugar. The added multivalency is therefore not a barrier for efficient recognition by bacterial receptors and it opens the route for adding different sugars that can be selected for their immunomodulatory properties.
Glycoclusters and glycodendrimers remain a growing source of interest in glycosciences (Renaudet and Roy, 2013). For a long time, synthetic chemists focused their efforts on structures displaying multiple copies of a single sugar unit to both clarify and modulate biological and pathological processes involving multivalent interactions with proteins (Bernardi et al., 2013; Cecioni et al., 2015; Arsiwala et al., 2019). However, these compounds only partially reflect the heterogeneous expression of glycans at the cell surface (i.e., glycocalyx) and completely underestimate that other sugar units may participate or promote additional biological events (Jiménez Blanco et al., 2013; Müller et al., 2016). For this reason, the development of structures displaying different rather than unique sugars, namely heteroglycoclusters (hGCs), as mimics of the glycocalyx is renewing the enthusiasm in this field.
So far, several heterovalent structures have been reported for studying the interaction process with a large class of carbohydrate-specific proteins (i.e., glycosidases, glycosyltransferases, lectins, antibodies) (Deguise et al., 2007; Ortega-Muñoz et al., 2009; Gómez-García et al., 2010; Lindhorst et al., 2010; Karskela et al., 2012; Abellán Flos et al., 2016; Vincent et al., 2016; Ortiz Mellet et al., 2017; Gade et al., 2018; Ogura et al., 2018) or for triggering multifaceted immune response against tumor cells (Ragupathi et al., 2002; Zhu et al., 2009; Pett et al., 2017). These studies highlight the complexity of heterocluster effects and reinforce the need of new structures to go one step further toward the understanding of naturally occurring recognition events and the development of more active compounds. Among recent reports, J. M. Garcia Fernandez and coworkers have demonstrated the impact of heterovalent display in both glycosidase inhibition and lectin binding using a competitive enzyme-lectin binding assay (Abellán Flos et al., 2016; García-Moreno et al., 2017). In addition, the Wong group have shown by glycan array that ligand density and neighboring sugars significantly affect the binding with antibodies (Liang et al., 2011). In another study, Reymond and co-workers focused their interest on the lectins LecA and LecB from Pseudomonas aeruginosa (Michaud et al., 2016). These two lectins play central roles in both the adhesion of the bacteria to host cells and the biofilm formation, causing severe damages in particular for immunocompromised patients (Mitchell et al., 2002; Imberty et al., 2004). Instead of using homovalent structures specific for either LecA or LecB, the authors postulated that the utilization of compounds combining both α-fucose (αFuc) and β-galactose (βGal) could bind simultaneously LecA and LecB, thus improving inhibitory effect to fight this antibiotic resistant bacterium. To this aim, glycodendrimers decorated with βGal and αFuc have been synthesized and promising activity in comparison with their previous homoclusters has been observed. This pioneering study prompted us to design new heterovalent glycodendrimers and to study their ability to bind these two lectins with high affinity and without loss of efficiency.
In the course of developing multivalent glycoconjugates (Galan et al., 2013; Daskhan et al., 2015), we recently focused on the synthesis of a variety of cyclopeptide-based hGCs using orthogonal chemoselective conjugation methods such as the oxime ligation (OL), the Cu(I)-catalyzed azide-alkyne cycloaddition (CuAAC), and the thiol-ene, thiol-chloroactetyl and diethyl squarate couplings (TEC, TCC, DSC). We thus synthesized diverse 4-, 8-, and 16-valent structures displaying from 2 to 4 different sugars in well-defined shuffled proportion and disposition (Thomas et al., 2012; Daskhan et al., 2016; Pifferi et al., 2017). In addition, sequential one-pot multi-click and iterative divergent strategies gave access to glycoconjugates with unprecedented structural complexity in excellent yields and purity (Thomas et al., 2015a). In the present study, we capitalized on these methodologies to synthesize glycodendrimers bearing βGal and αFuc with different chemical linkage and evaluated their binding to LecA and LecB. Because αMan is ligand of LecB, though with a lower affinity than αFuc, we also synthesized different combinations of hGCs containing this residue to evaluate its potential influence in the binding to these two lectins.
In a previous report, we described a series of homovalent glycodendrimers with nanomolar affinity for LecB (Berthet et al., 2013). These compounds have been prepared by a convergent oxime conjugation of cyclopeptides and/or polylysine dendrons then aminooxylated sugar units have been grafted at the periphery. However, the same strategy is not compatible to introduce two different sugars in a regioselective and controlled manner (Bossu et al., 2011). Instead, we used here OL and CuAAC to secure the molecular assembly and the final purification of complex structures (Thomas et al., 2015b). To do so, the construction of the dendrimer core was first carried out from a central cyclopeptide A (Figure 1) containing two aminooxy groups and two serine as oxo-aldehyde precursors as previously described (Pifferi et al., 2017). This scaffold A was successively functionalized with two cyclopeptides: the first one B (right arm) contains one aldehyde and four azido groups, whereas the second one C (left arm) displays one aminooxy and four serines. Treatment with sodium periodate of the resulting hexadecavalent dendrimer afforded 1 which was functionalized with aminooxylated αFuc (2), βGal (3), and αMan (4) in aqueous solution containing 0.1% TFA at 37°C for 30 min. These three octavalent conjugates displaying eight copies of αFuc (5), βGal (6), and αMan (7) were subsequently conjugated without further purification with propargylated αFuc (8), βGal (9), and/or αMan (10) in PBS (pH 7.4, 10 mM) with CuSO4, tris(3-hydroxypropyltriazolylmethylamine) (THPTA) and sodium ascorbate (Figure 2). After RP-HPLC purification, the resulting hGCs 11–14 were obtained in 76–84% yield.
Figure 1. Strategy for the construction of the hexadecavalent scaffold 1. Reagents and conditions (Pifferi et al., 2017): (1) 0.1% TFA in H2O/CH3CN (1:1), 37°C, 30 min; (2) i: NaIO4, H2O, r.t., 40 min; ii: 0.1% TFA in H2O/CH3CN (1:1), 37°C, 30 min; (3) NaIO4, H2O, r.t., 40 min.
Figure 2. Synthesis of hGCs 11–14 by a mixed OL and CuAAC strategy. Reagents and conditions: (1) 2, 3, or 4, 0.1% TFA in H2O/CH3CN, 37°C, 30 min; (2) 8, 9, or 10, CuSO4, Na ascorbate, THPTA, PBS (pH 7.4, 10 mM), r.t., 90 min, 81% for 11, 84% for 12, 77% for 13, and 76% for 14.
The resulting hGCs were tested in solution with lectins by Isothermal Titration Calorimetry (Table 1) and their binding properties were compared with homoclusters 15–20 (Berthet et al., 2013; Thomas et al., 2015b) displaying either 4 and 16 βGal or αFuc units attached with an oxime or a triazole linker (Figure 3). Methyl α-L-fucoside (αFuc-OMe) and methyl β-D-galactoside (βGal-OMe) were used as monovalent references for LecB and LecA to calculate the relative potency (α) and relative potency per sugar (β) of each multivalent compound. Experiments were performed in direct injection mode (i.e., ligand in syringe and protein in cell) to minimize aggregation problems.
For LecB, we observed modest and similar Kd (0.3 μM) for tetravalent compounds 15 and 16, with negligible improvement factor α compared to αFuc-OMe indicating the absence of cluster effect (Table 1, Figure 4A, and Supplementary Information). Instead strong aggregation is observed as shown on the thermogram in Figure 4A. The analysis of the thermodynamic contributions indicates a strong gain in enthalpy but that is completely counterbalanced by the entropy cost. This again indicates that the tetravalent compound is bridging between different lectin tetramers instead of clustering neighboring binding sites.
Figure 4. ITC data. Thermograms obtained by injections of (A) glycocluster 16 at 140 μM in a solution of LecB (70 μM) and (B) glycocluster 18 at 80 μM in a solution of LecA (35 μM) with corresponding integrated titration curves. Molar ratio is indicated as number of glycocluster molecules per lectin monomer.
By contrast, for LecA, a very strong improvement of binding was observed for both tetravalent compounds 17 and 18 (Kd = 22 nM) compared to βGal-OMe (Table 1). This suggests a favorable presentation of sugar for the binding to LecA (Cecioni et al., 2012) with geometry allowing for a real clustering effect, also reflected in the huge enthalpy gain. Compound 18 that contains a triazole linkage instead of oxime (17) is the most efficient with a factor α of 7,000 and factor β of 1,700 when reported to the number of sugar (Table 1, Figure 4B, and Supplementary Information). This result is in good agreement with the well-known preference of LecA for β-galactoside containing an aromatic moiety near the anomeric position (Cecioni et al., 2012).
Interestingly, multimerisation of the tetravalent structures 15 and 18 as the hexadecavalent dendrimers 19 and 20 allowed 10-fold binding improvement for fucosylated compounds with LecB (Kd = 44 nM for 19). Only minor difference in Kd was observed between 18 and 20 and LecA (Kd = 22 and 14 nM, respectively), despite a more favorable binding enthalpy for 20 (–ΔH = 378 kJ/mol) and a higher improvement factor α (10,000) compared to βGal-OMe. While both 18 and 20 are excellent ligands for LecA, compound 20 shows a 2.5-lower β factor than 18 which suggests that increasing valency over four sugars units has low effect for enhancing affinity with LecA. Similar observations have been already reported in other studies (Cecioni et al., 2012; Michaud et al., 2016).
We next evaluated the binding potency of hGCs for LecA and LecB by ITC (Table 1, Figure 5, and Supplementary Information). Two compounds 11 and 13 display eight copies of αFuc and βGal with either oxime or triazole linkers and two other hGCs combine αMan with αFuc (12) or βGal (14). As observed with tetravalent clusters 15–16 with LecB, a higher affinity (Kd = 85–92 nM) was measured when the fucose unit is linked by an oxime linkage. These compounds 11 and 12 showed a more favorable binding enthalpy (–ΔH = 212 kJ/mol), suggesting a more favorable geometry and presentation of fucose. Moreover, no significant difference of affinity was observed between 11 and 12 with binding improvement in the same range of magnitude than homovalent compounds (i.e., β factor lower than 1), indicating the absence of influence of the partner sugar (Man or Gal).
Figure 5. ITC data. Thermograms obtained by injections of (A) glycodendrimer 11 at 50 μM in a solution of LecB (32 μM), (B) glycodendrimer 11 (50 μM) in a solution of LecA (32 μM), and (C) glycodendrimer 14 (40 μM) in a solution of LecA (29 μM) with corresponding integrated titration curves. Molar ratio is indicated as number of glycocluster molecules per lectin monomer.
As for LecA, as mentioned with homovalent structures 17–20, hGCs displaying βGal 11, 13, and 14 lead to similar Kd (21–35 nM), showing strong binding entropy contribution (–ΔS = 173–199 kJ/mol) counterbalanced by favorable binding enthalpy (–ΔH = 216–243 kJ/mol).
When the binding is compared to parent galactosyl-homoclusters, the β factor was ~500 for both βGal-αFuc hGCs 11 and 13 and 900 for 14 which displays βGal-αMan combination. The stoichiometry data for these three compounds indicated that approximately six βGal can bind to a monomer of LecA, which indicates a good accessibility despite the presence of non-specific sugars close to βGal. This results supports the fact that high affinity could be due to an aggregative binding mode. In the case of LecB, the stoichiometry measured for 11–13 (n = 0.1) lead to a similar hypothesis. Altogether, our data suggest that hGCs combining both βGal and αFuc derivatives not only bind to both lectins with nanomolar affinity, but also that the presence of the other sugar does not affect the binding potency.
We have developed a synthetic strategy to prepare a series of heteroglycoclusters displaying eight copies of αFuc and βGal. Binding assays with both LecA and LecB lectins from Pseudomonas aeruginosa have revealed that the combination of αFuc and βGal with unspecific sugars maintain the binding potency of the parent homoclusters with both lectins. The review by Jiménez Blanco et al. (2013) opened the questions of the effect of functional promiscuity of glycoligands on different heteroglycocompounds, since such complex architecture are efficient mimics of cell surfaces. The authors raised concerns that the complexity may create “messiness or noise in the processes they participate in.” We could demonstrate here that the crowding with an alternate sugar does not lower the efficiency of the glycocluster to bind to the bacteria lectins. In the future, this could be of importance for designing active compounds that will have the capacity of both binding to the bacterial surface and activating the immune system by different clusters of sugars.
All chemical reagents were purchased from Aldrich (Saint Quentin Fallavier, France) or Acros (Noisy-Le-Grand, France). All protected amino acids and Fmoc-Gly-Sasrin® resin was obtained from Advanced ChemTech Europe (Brussels, Belgium). For peptides and glycopeptides, analytical RP-HPLC was performed on Waters system equipped with a Waters 2695 separations module and a Waters 2487 Dual Absorbance UV/Visible Detector. Analysis was carried out at 1.0 mL/min (EC 125/3 nucleosil 300-5 C18) with UV monitoring at 214 nm and 250 nm using a linear A–B gradient (buffer A: 0.09% CF3CO2H in water; buffer B: 0.09% CF3CO2H in 90% acetonitrile). Purifications were carried out at 22.0 mL/min (VP 250/21 nucleosil 100-7 C18) with UV monitoring at 214 nm and 250 nm using a linear A–B gradient. HRMS and ESI-MS and HRMS spectra of peptides and glycopeptides were measured on an Esquire 3000 spectrometer from Bruker. Lectins LecA and LecB were produced in recombinant form in Escherichia coli BL21(DE3) as described previously (Mitchell et al., 2005; Blanchard et al., 2008).
Cyclopeptide 1 (4.5 mg, 0.72 μmol) and aminooxy Fuc 2 (1.6 mg, 8.7 μmol) were dissolved in 0.1% TFA in H2O (10 mM). After stirring for 30 min at room temperature, the propargyl Gal 9 (5.1 mg, 23.2 μmol) dissolved in DMF (1 mL) and a solution of CuSO4 (1,4 mg, 5.8 μmol) in PBS buffer (500 μL, 100 mM) were added. Then was added a solution of THPTA (10.1 mg, 23.2 μmol) and sodium ascorbate (5.1 mg, 40.1 μmol) in PBS buffer (500 μL, 100 mM). All solutions were previously degassed under argon. The reaction was stirred at room temperature under argon and after 1 h analytical HPLC indicated complete reaction coupling. Then Chelex resin was added to remove excess of copper and the reaction mixture was directly purified by RP-HPLC affording pure compound as a white powder. Yield: 81% (5.4 mg, 0.59 μmol); RP-HPLC: Rt = 4.28 min (C18, 214 nm 5–100% B in 15 min); MS (ESI+) m/z calcd. for C387H619N103O158 [M+5H]5+: 1847.3, found 1847.4.
Compound 12 was obtained from compound 1, aminooxy Fuc 2, and propargyl Man 10 following the procedure described for 11. Yield: 84% (5.6 mg, 0.61 μmol); RP-HPLC: Rt = 4.33 min (C18, 214 nm 5–100% B in 15 min); MS (ESI+) m/z calcd. for C387H618N103O158 [M+4H]4+: 2309.1, found 2308.7
Compound 13 was obtained from compound 1, aminooxy Gal 3 and propargyl Fuc 8 following the procedure described for 11. Yield: 77% (3.5 mg, 0.38 μmol); RP-HPLC: Rt = 4.34 min (C18, 214 nm 5–100% B in 15 min); MS (ESI+) m/z calcd. for C387H619N103O158 [M+5H]5+: 1847.3, found 1847.3.
Compound 14 was obtained from compound 1, aminooxy Man 4 and propargyl Gal 9 following the procedure described for 11. Yield: 76% (3.1 mg, 0.33 μmol); RP-HPLC: Rt = 10.62 min (C18, 214 nm 5–100% B in 20 min); MS (ESI+) m/z calcd. for C387H619N103O166 [M+5H]5+: 1873.7, found: 1874.1.
Aldehyde-containing cyclopeptide (Singh et al., 2005) (10.0 mg, 8.04 μmol) and aminooxy Fuc 2 (8.6 mg, 48.23 μmol) were dissolved in 0.1% TFA in H2O (10 mM). After stirring for 30 min at room temperature, the crude mixture was purified by RP-HPLC. Fractions containing the product were combined and lyophilized to afford the title compound as a white fluffy powder (12.8 mg, 6.75 μmol, 84%). RP-HPLC: Rt = 4.77 min (C18, 214 nm 5–60% B in 15 min); HRMS (ESI+) m/z calcd. for C79H130N19O34 [M+H]+: 1888.9027, found: 1888.9058.
The title compound was prepared according to already published protocol. Analytical data were in agreement with the literature (Ribeiro et al., 2018).
The title compound was prepared following the procedure described for 15 using aminooxy Gal 3 (7.5 mg, 38.6 μmol) and was obtained as a white fluffy powder after lyophilisation (13.7 mg, 7.02 μmol, 80%). RP-HPLC: Rt = 3.38 min (C18, 214 nm 5–60% B in 15 min); HRMS (ESI+) m/z calcd. for C79H130N19O38 [M+H]+: 1952.8824, found: 1952.8890.
The title compound was prepared following the procedure described for 16 from propargyl Gal 9 (13 mg, 58.7 μmol) and was obtained as a white fluffy powder after lyophilisation (13.7 mg, 7.02 μmol, 80%). RP-HPLC: Rt = 4.17 min (C18, 214 nm 5–60% B in 15 min); HRMS (ESI+) m/z calcd. for C83H134N22O34 [M+H]+: 1996.9464, found: 1996.9428.
The title compound was prepared according to already published protocol. Analytical date were in agreement with the literature (Berthet et al., 2013).
The title compound was prepared according to the same protocol as compound 18 and was obtained as a white fluffy powder after lyophilisation (4.8 mg, 0.51 μmol, 69%). RP-HPLC: Rt = 3.98 min (C18, 214 nm 5–60% B in 15 min); MS (ESI+) m/z calcd. for C395H628N111O158 [M+7H]7+: 1350.2, found: 1351.8
ITC experiments of compounds 15–18 were perfomed with a PEAQ-ITC titration calorimeter (Microcal). Other compounds were assayed with a VP-ITC isothermal titration calorimeter (Microcal). The experiments were carried out at 25°C. All glycocompounds and lectins LecB and LecA were dissolved in the same buffer composed of 20 mM Tris with 100 mM NaCl and 0.1 mM CaCl2 at pH 7.5. For PEAQ-ITC, the lectins were placed in the microcalorimeter cell (200 μL) at concentrations varying from 35 to 70 μM. A total of 29 injections of 1.3 μL of glycoclusters at concentrations varying from 80 to 140 μM were performed. For VP-ITC, the microcalorimeter cell (1.447 mL) contained the lectins with concentrations between 30 and 100 μM. A total of 30 injections of 10 μL were performed intervals of 5 min while stirring at 310 rpm with glycoclusters concentrations varying from 0.40 to 0.150 μM. The experimental data were fitted to a theoretical titration curve using the Microcal PEAQ-ITC analysis software, with ΔH (enthalpy change), Ka (association constant), and N (number of binding sites per monomer) as adjustable parameters. Dissociation constant (Kd), free energy change (ΔG), and entropy contributions (TΔS) were derived from the previous ones. Two or three independent titrations were performed for each ligand tested.
All datasets generated for this study are included in the manuscript/Supplementary Files.
OR designed the study. DG and BT synthetized the compounds. DG and EG performed the ITC analysis. DG, AI, and OR wrote the manuscript.
This work was supported by CNRS, Université Grenoble Alpes, ICMG FR 2607, the French ANR project Glyco@Alps (ANR-15-IDEX-02), Labex ARCANE, and CBH-EUR-GS (ANR-17-EURE-0003).
The authors declare that the research was conducted in the absence of any commercial or financial relationships that could be construed as a potential conflict of interest.
OR acknowledge the European Research Council Consolidator Grant LEGO (647938) for DG.
The Supplementary Material for this article can be found online at: https://www.frontiersin.org/articles/10.3389/fchem.2019.00666/full#supplementary-material
Abellán Flos, M., García Moreno, M. I., Ortiz Mellet, C., García Fernández, J. M., Nierengarten, J. F., and Vincent, S. P. (2016). Potent glycosidase inhibition with heterovalent fullerenes: unveiling the binding modes triggering multivalent inhibition. Chemistry 22, 11450–11460. doi: 10.1002/chem.201601673
Arsiwala, A., Castro, A., Frey, S., Stathos, M., and Kane, R. S. (2019). Designing multivalent ligands to control biological interactions: from vaccines and cellular effectors to targeted drug delivery. Chem. Asian J. 14, 244–255. doi: 10.1002/asia.201801677
Bernardi, A., Jiménez-Barbero, J., Casnati, A., De Castro, C., Darbre, T., Fieschi, F., et al. (2013). Multivalent glycoconjugates as anti-pathogenic agents. Chem. Soc. Rev. 42, 4709–4727. doi: 10.1039/C2CS35408J
Berthet, N., Thomas, B., Bossu, I., Dufour, E., Gillon, E., Garcia, J., et al. (2013). High affinity glycodendrimers for the lectin LecB from Pseudomonas aeruginosa. Bioconjug. Chem. 24, 1598–1611. doi: 10.1021/bc400239m
Blanchard, B., Nurisso, A., Hollville, E., Tétaud, C., Wiels, J., Pokorná, M., et al. (2008). Structural basis of the preferential binding for globo-series glycosphingolipids displayed by Pseudomonas aeruginosa lectin I. J. Mol. Biol. 383, 837–853. doi: 10.1016/j.jmb.2008.08.028
Bossu, I., Šulc, M., Krenek, K., Dufour, E., Garcia, J., Berthet, N., et al. (2011). Dendri-RAFTs: a second generation of cyclopeptide-based glycoclusters. Org. Biomol. Chem. 9, 1948–1959. doi: 10.1039/c0ob00772b
Cecioni, S., Faure, S., Darbost, U., Bonnamour, I., Parrot-Lopez, H., Roy, O., et al. (2011). Selectivity among two lectins: probing the effect of topology, multivalency and flexibility of “clicked” multivalent glycoclusters. Chemistry 17, 2146–2159. doi: 10.1002/chem.201002635
Cecioni, S., Imberty, A., and Vidal, S. (2015). Glycomimetics versus multivalent glycoconjugates for the design of high affinity lectin ligands. Chem. Rev. 115, 525–561. doi: 10.1021/cr500303t
Cecioni, S., Praly, J. P., Matthews, S. E., Wimmerov,á, M., Imberty, A., and Vidal, S. (2012). Rational design and synthesis of optimized glycoclusters for multivalent lectin-carbohydrate interactions: influence of the linker arm. Chemistry 18, 6250–6263. doi: 10.1002/chem.201200010
Daskhan, G. C., Berthet, N., Thomas, B., Fiore, M., and Renaudet, O. (2015). Multivalent glycocyclopeptides: toward nano-sized glycostructures. Carbohydr. Res. 405, 13–22. doi: 10.1016/j.carres.2014.07.017
Daskhan, G. C., Pifferi, C., and Renaudet, O. (2016). Synthesis of a new series of sialylated homo- and heterovalent glycoclusters by using orthogonal ligations. ChemistryOpen 5, 477–484. doi: 10.1002/open.201600062
Deguise, I., Lagnoux, D., and Roy, R. (2007). Synthesis of glycodendrimers containing both fucoside and galactoside residues and their binding properties to Pa-IL and PA-IIL lectins from Pseudomonas aeruginosa. N. J. Chem. 31, 1321–1331. doi: 10.1039/b701237c
Gade, M., Alex, C., Leviatan Ben-Arye, S., Monteiro, J. T., Yehuda, S., Lepenies, B., et al. (2018). Microarray analysis of oligosaccharide-mediated multivalent carbohydrate–protein interactions and their heterogeneity. Chembiochem 19, 1170–1177. doi: 10.1002/cbic.201800037
Galan, M. C., Dumy, P., and Renaudet, O. (2013). Multivalent glyco(cyclo)peptides. Chem. Soc. Rev. 42, 4599–4612. doi: 10.1039/C2CS35413F
García-Moreno, M. I., Ortega-Caballero, F., Rísquez-Cuadro, R., Ortiz Mellet, C., and García Fernández, J. M. (2017). The impact of heteromultivalency in lectin recognition and glycosidase inhibition: an integrated mechanistic study. Chemistry 23, 6295–6304. doi: 10.1002/chem.201700470
Gómez-García, M., Benito, J. M., Gutiérrez-Gallego, R., Maestre, A., Mellet, C. O., Fernández, J. M., et al. (2010). Comparative studies on lectin–carbohydrate interactions in low and high density homo- and heteroglycoclusters. Org. Biomol. Chem. 8, 1849–1860. doi: 10.1039/b920048g
Imberty, A., Wimmerová, M., Mitchell, E. P., and Gilboa-Garber, N. (2004). Structures of the lectins from Pseudomonas aeruginosa: insight into the molecular basis for host glycan recognition. Microbes Infect. 6, 221–228. doi: 10.1016/j.micinf.2003.10.016
Jiménez Blanco, J. L., Ortiz Mellet, C., and García Fernández, J. M. (2013). Multivalency in heterogeneous glycoenvironments: hetero-glycoclusters, -glycopolymers and -glycoassemblies. Chem. Soc. Rev. 42, 4518–4531. doi: 10.1039/C2CS35219B
Karskela, M., von Usedom, M., Virta, M., and Lönnberg, P. (2012). Synthesis of biotinylated multipodal glycoclusters on a solid support. Eur. J. Org. Chem. 2012, 6594–6605. doi: 10.1002/ejoc.201200926
Liang, C. H., Wang, S. K., Lin, C. W., Wang, C. C., Wong, C. H., and Wu, C. Y. (2011). Effects of neighboring glycans on antibody-carbohydrate interaction. Angew. Chem. Int. Ed Engl. 50, 1608–1612. doi: 10.1002/anie.201003482
Lindhorst, T. K., Bruegge, K., Fuchs, A., and Sperling, O. (2010). A bivalent glycopeptide to target two putative carbohydrate binding sites on FimH. Beilstein J. Org. Chem. 6, 801–809. doi: 10.3762/bjoc.6.90
Michaud, G., Visini, R., Bergmann, M., Salerno, G., Bosco, R., Gillon, E., et al. (2016). Overcoming antibiotic resistance in Pseudomonas aeruginosa biofilms using glycopeptide dendrimers. Chem. Sci. 7, 166–182. doi: 10.1039/C5SC03635F
Mitchell, E., Houles, C., Sudakevitz, D., Wimmerova, M., Gautier, C., Pérez, S., et al. (2002). Structural basis for oligosaccharide-mediated adhesion of Pseudomonas aeruginosa in the lungs of cystic fibrosis patients. Nat. Struct. Biol. 9, 918–921. doi: 10.1038/nsb865
Mitchell, E. P., Sabin, C., Snajdrová, L., Pokorná, M., Perret, S., Gautier, C., et al. (2005). High affinity fucose binding of Pseudomonas aeruginosa lectin PA-IIL: 1.0 A resolution crystal structure of the complex combined with thermodynamics and computational chemistry approaches. Proteins 58, 735–746. doi: 10.1002/prot.20330
Müller, C., Despras, G., and Lindhorst, T. K. (2016). Organizing multivalency in carbohydrate recognition. Chem. Soc. Rev. 45, 3275–3302. doi: 10.1039/C6CS00165C
Ogura, A., Urano, S., Tahara, T., Nozaki, S., Sibgatullina, R., Vong, K., et al. (2018). A viable strategy for screening the effects of glycan heterogeneity on target organ adhesion and biodistribution in live mice. Chem. Commun. 54, 8693–8696. doi: 10.1039/C8CC01544A
Ortega-Muñoz, M., Perez-Balderas, F., Sanfrutos, J. M., Hernandez-Mateo, F., Isac-García, J., Santoyo-González, F., et al. (2009). Click multivalent heterogeneous neoglycoconjugates – modular synthesis and evaluation of their binding affinities. Eur. J. Org. Chem. 2009, 2454–2473. doi: 10.1002/ejoc.200801169
Ortiz Mellet, C., J.-,Nierengarten, F., and García Fernández, J. M. (2017). Multivalency as an action principle in multimodal lectin recognition and glycosidase inhibition: a paradigm shift driven by carbon-based glyconanomaterials. J. Mater. Chem. B 5, 6428–6436. doi: 10.1039/C7TB00860K
Pett, C., Cai, H., Liu, J., Palitzsch, B., Schorlemer, M., Hartmann, S., et al. (2017). Microarray analysis of antibodies induced with synthetic antitumor vaccines: specificity against diverse mucin core structures. Chemistry 23, 3875–3884. doi: 10.1002/chem.201603921
Pifferi, C., Thomas, B., Goyard, D., Berthet, N., and Renaudet, O. (2017). Heterovalent glycodendrimers as epitope carriers for antitumor synthetic vaccines. Chemistry 23, 16283–16296. doi: 10.1002/chem.201702708
Ragupathi, G., Coltart, D. M., Williams, L. J., Koide, F., Kagan, E., Allen, J., et al. (2002). On the power of chemical synthesis: immunological evaluation of models for multiantigenic carbohydrate-based cancer vaccines. Proc. Natl. Acad. Sci. U.S.A. 99, 13699–13704. doi: 10.1073/pnas.202427599
Renaudet, O., and Roy, R. (2013). Multivalent scaffolds in glycoscience: an overview. Chem. Soc. Rev. 42, 4515–4517. doi: 10.1039/c3cs90029k
Ribeiro, J. P., Villringer, S., Goyard, D., Coche-Guerente, L., Höferlin, M., Renaudet, O., et al. (2018). Tailor-made Janus lectin with dual avidity assembles glycoconjugate multilayers and crosslinks protocells. Chem. Sci. 9, 7634–7641. doi: 10.1039/C8SC02730G
Singh, Y., Renaudet, O., Defrancq, E., and Dumy, P. (2005). Preparation of a multitopic glycopeptide-oligonucleotide conjugate. Org. Lett. 7, 1359–1362. doi: 10.1021/ol050134n
Thomas, B., Fiore, M., Bossu, I., Dumy, P., and Renaudet, O. (2012). Synthesis of heteroglycoclusters by using orthogonal chemoselective ligations. Beilstein J. Org. Chem. 8, 421–427. doi: 10.3762/bjoc.8.47
Thomas, B., Fiore, M., Daskhan, G. C., Spinelli, N., and Renaudet, O. (2015a). A multi-ligation strategy for the synthesis of heterofunctionalized glycosylated scaffolds. Chem. Commun. 51, 5436–5439. doi: 10.1039/C4CC05451B
Thomas, B., Pifferi, C., Daskhan, G. C., Fiore, M., Berthet, N., and Renaudet, O. (2015b). Divergent and convergent synthesis of GalNAc-conjugated dendrimers using dual orthogonal ligations. Org. Biomol. Chem. 13, 11529–11538. doi: 10.1039/C5OB01870F
Vincent, S. P., Buffet, K., Nierengarten, I., Imberty, A., and Nierengarten, J. F. (2016). Biologically active heteroglycoclusters constructed on a pillar[5]arene-containing [2]rotaxane scaffold. Chemistry 22, 88–92. doi: 10.1002/chem.201504110
Keywords: heteroglycocluster, multivalency, orthogonal ligation, lectin, Pseudomonas aeruginosa
Citation: Goyard D, Thomas B, Gillon E, Imberty A and Renaudet O (2019) Heteroglycoclusters With Dual Nanomolar Affinities for the Lectins LecA and LecB From Pseudomonas aeruginosa. Front. Chem. 7:666. doi: 10.3389/fchem.2019.00666
Received: 29 July 2019; Accepted: 18 September 2019;
Published: 02 October 2019.
Edited by:
Karina Valeria Mariño, Institute of Biology and Experimental Medicine (IBYME), ArgentinaReviewed by:
Maria Laura Uhrig, Universidad de Buenos Aires, ArgentinaCopyright © 2019 Goyard, Thomas, Gillon, Imberty and Renaudet. This is an open-access article distributed under the terms of the Creative Commons Attribution License (CC BY). The use, distribution or reproduction in other forums is permitted, provided the original author(s) and the copyright owner(s) are credited and that the original publication in this journal is cited, in accordance with accepted academic practice. No use, distribution or reproduction is permitted which does not comply with these terms.
*Correspondence: Anne Imberty, YW5uZS5pbWJlcnR5QGNlcm1hdi5jbnJzLmZy; Olivier Renaudet, b2xpdmllci5yZW5hdWRldEB1bml2LWdyZW5vYmxlLWFscGVzLmZy
Disclaimer: All claims expressed in this article are solely those of the authors and do not necessarily represent those of their affiliated organizations, or those of the publisher, the editors and the reviewers. Any product that may be evaluated in this article or claim that may be made by its manufacturer is not guaranteed or endorsed by the publisher.
Research integrity at Frontiers
Learn more about the work of our research integrity team to safeguard the quality of each article we publish.