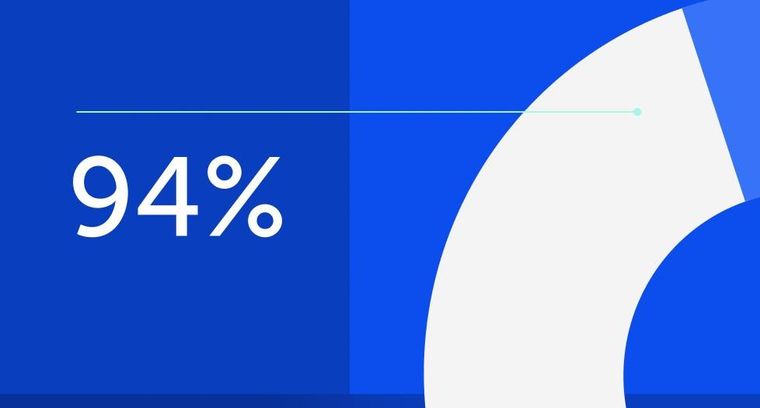
94% of researchers rate our articles as excellent or good
Learn more about the work of our research integrity team to safeguard the quality of each article we publish.
Find out more
ORIGINAL RESEARCH article
Front. Water, 09 April 2025
Sec. Water Resource Management
Volume 7 - 2025 | https://doi.org/10.3389/frwa.2025.1564842
This article is part of the Research TopicAdvancements and Challenges in Sustainable Water Desalination TechnologiesView all 3 articles
This study examines the feasibility and economic performance of Forward Osmosis (FO) desalination systems powered by Concentrated Solar Power (CSP) technologies, specifically Parabolic Trough (PT) and Linear Fresnel Reflector (LFR) systems. The study evaluates two operational scenarios: batch-mode operation without Thermal Energy Storage (TES) and continuous operation with TES. Seawater serves as the Feed Solution (FS), while Dimethyl Ether (DME) is used as the Draw Solution (DS), recovered through a thermal separation process powered by CSP. Key performance metrics analysed include the Capacity Factor (CF), Levelized Cost of Thermal Energy (LCoT), and Levelized Cost of Water (LCoW). Results indicate that in the non-TES scenario, where the FO system operates for 8 h per day, the PT system achieves the lowest LCoW, ranging between $0.74/m3 and $1.91/m3, while the LFR system exhibits slightly higher costs at $0.89/m3 to $2.38/m3. However, this batch-mode operation limits system efficiency and results in excess unused solar energy due to defocusing. In contrast, the TES scenario enables 24-h continuous operation, significantly increasing CF to 99.73% for LFR but also raising costs. The LCoW for LFR in this configuration ranges from $1.69/m3 to $3.48/m3, with the high cost of TES driving up LCoT between 7.72 and 11.60 c$/kWhth. The findings highlight a fundamental trade-off: while TES enhances operational stability, it introduces prohibitive storage costs, making batch-mode CSP-FO configurations more economically viable. FO module costs significantly impact LCoW, with thermal energy OPEX dominating cost composition in TES cases, while FO CAPEX becomes more influential at higher module prices. Additionally, lower solar field output temperatures reduce LCoW in non-TES scenarios, whereas in TES cases, storage costs remain the primary determinant of economic performance. This study contributes to the growing body of research on sustainable desalination by critically assessing CSP-FO integration under real-world constraints. Compared to conventional RO or MED systems, CSP-FO presents a viable alternative in regions where direct solar-driven desalination is preferred. Future research should prioritize reducing TES costs through alternative storage materials, exploring hybrid CSP-PV-FO configurations, and enhancing FO membrane efficiency to improve overall system feasibility and scalability in arid regions.
Forward osmosis (FO) has gained attention as an alternative desalination technology due to its lower energy requirements and higher water recovery efficiency compared to conventional methods like reverse osmosis (RO) (Cath et al., 2006). Unlike RO, which relies on hydraulic pressure, FO operates based on osmotic pressure differences across a semi-permeable membrane, reducing the reliance on high-pressure pumps and minimizing energy consumption (McGinnis and Elimelech, 2007). This makes FO particularly attractive for regions facing energy constraints and water scarcity, such as the Middle East.
A key determinant of FO system efficiency is the selection of an appropriate draw solution. Various draw solutions have been investigated, each with distinct advantages and limitations. Ammonium bicarbonate has been widely studied due to its high osmotic pressure and ease of regeneration (McGinnis and Elimelech, 2007). However, its volatility and potential environmental impact restrict large-scale implementation. Consequently, researchers have explored alternative draw solutions, including organic compounds and ionic liquids (Zhang et al., 2014; Xie et al., 2015). Dimethyl ether (DME) has emerged as a promising candidate due to its high osmotic pressure, low molecular weight, and efficient thermal separation from product water (Sato et al., 2014). Unlike other draw solutions, DME can be efficiently regenerated using low-grade thermal energy (Foo et al., 2024), making it particularly well-suited for integration with concentrated solar power (CSP). However, further research is needed to evaluate its scalability and economic feasibility in large-scale FO desalination systems. A comparison of the osmotic efficiency, stability and energy requirements for regeneration are shown in Table 1.
This comparison highlights why DME is an optimal choice for FO desalination, particularly when paired with CSP for sustainable thermal energy recovery.
CSP has demonstrated significant potential as a renewable energy source for desalination, particularly in regions with high solar irradiance (Mahmoud et al., 2023). Unlike photovoltaic (PV) systems, which suffer from efficiency losses at elevated temperatures, CSP technologies—including parabolic troughs (PT), solar towers (ST), and linear Fresnel reflectors (LFR)—maintain efficiency at higher temperatures and offer thermal energy storage (TES) capabilities (Lovegrove and Stein, 2012; Abdelrazik et al., 2023). This enables stable and continuous desalination operations without reliance on conventional energy storage methods. Recent research has explored various hybrid desalination configurations, including CSP-RO, CSP-MED (Multi-Effect Distillation), and CSP-FO systems (Alghassab, 2024). However, comprehensive assessments of CSP-FO integration remain limited, particularly regarding economic trade-offs and long-term operational viability (Moharram et al., 2021).
Despite significant advancements in desalination technologies, integrating FO with CSP remains an underexplored area, requiring a thorough evaluation of technical feasibility, economic viability, and long-term sustainability. Studies on CSP-driven desalination have primarily focused on RO and MED, with limited consideration for FO (Abdelrazik et al., 2024), despite its potential advantages in reducing energy consumption and operational costs (Mahmoud et al., 2023). Moreover, the lack of large-scale pilot projects and real-world case studies on CSP-FO integration has hindered its adoption (McMillan et al., 2023). Addressing these gaps requires a contextualized assessment of CSP-FO systems within the broader desalination landscape, particularly in arid regions where water scarcity and high solar potential make this integration highly relevant.
The Gulf Cooperation Council (GCC) countries, including Saudi Arabia, face critical water scarcity issues due to their arid climates and limited natural freshwater resources. These nations currently rely heavily on energy-intensive desalination processes, predominantly RO, which are susceptible to efficiency losses under high-temperature conditions (Bartels et al., 2008). The adoption of a CSP-FO system offers a potential alternative, aligning with the strategic goals of diversifying energy portfolios and reducing environmental impact across the region (Atanasovska, 2016). Moreover, this aligns with broader sustainability objectives outlined in regional development frameworks, such as Saudi Arabia’s Vision 2030 (2016). Given the high solar irradiance levels and increasing interest in renewable energy solutions in the GCC, assessing CSP-FO integration under these conditions provides valuable insights into the practicality and scalability of such systems due to its arid climate and limited natural freshwater resources.
The integration of Concentrated Solar Power (CSP) with Forward Osmosis (FO) desalination presents a promising alternative for sustainable water production, particularly in regions with high solar energy potential. While CSP-driven desalination has been widely studied in configurations such as CSP-MED (Multi-Effect Distillation) and CSP-RO (Reverse Osmosis), there remains a notable research gap in the comprehensive assessment of CSP-FO systems, particularly regarding economic trade-offs, membrane performance at high temperatures, and large-scale scalability (Tiwari et al., 2022).
The feasibility of CSP-FO depends on several critical factors, including the stability of FO membranes under thermal stress, energy recovery efficiency, and the overall cost-effectiveness compared to other solar desalination methods (Elwardany et al., 2024). Some studies have highlighted the potential of hybrid CSP-FO systems in leveraging solar thermal energy for water treatment, yet there is a lack of systematic analysis on operational sustainability and capital investment requirements (Alghassab, 2024). The work of Elwardany et al. (2024). provides valuable insights into solar-driven desalination, particularly in hybrid membrane-based configurations, emphasizing the need for further exploration of energy optimization in these systems.
Given the strategic significance of diversifying energy portfolios and reducing environmental impact, it is imperative to conduct detailed techno-economic evaluations that examine long-term viability, cost-effectiveness, and integration challenges associated with CSP-FO desalination. This research addresses the interplay between solar energy availability, membrane durability, and the economic feasibility of large-scale implementation, ensuring that CSP-FO systems can be a viable and sustainable solution for addressing water scarcity challenges.
The techno-economic feasibility of a CSP-FO system using DME as a draw solution, focusing on operational parameters such as CSP technology selection, capacity factor (CF), TES, solar field output temperature, and overall system costs was evaluated in this study. The analysis compares batch-mode FO operation without TES and continuous operation with TES to assess trade-offs between cost and efficiency. By addressing these aspects, the study provides critical insights into the suitability of FO-CSP desalination for high-temperature environments and contributes to the advancement of sustainable water treatment solutions.
This study evaluates the integration of Forward Osmosis (FO) desalination with Concentrated Solar Power (CSP) systems in addressing water scarcity in arid regions, particularly in the Gulf Cooperation Council (GCC) countries. The methodology focuses on utilizing Dimethyl Ether (DME) as the Draw Solution (DS) in the FO process, which operates at low pressure to transfer water from pre-treated seawater to the DS. CSP technologies provide the thermal energy required to regenerate the DS, ensuring efficient and sustainable water desalination.
Two CSP technologies were analysed:
• Parabolic Trough (PT): Uses steam as the Heat Transfer Fluid (HTF) for thermal energy delivery.
• Linear Fresnel Reflector (LFR): Utilizes thermal oil as the HTF, enabling efficient heat storage and transfer.
These CSP systems were evaluated under two operational scenarios:
1. Without Thermal Energy Storage (TES): The FO system operates as a batch process for 8 h daily, resulting in a Capacity Factor (CF) of approximately 33%. The solar field is sized to supply the required thermal energy directly during peak sunlight hours.
2. With TES: A TES system using thermal oil enables continuous 24-h operation, increasing CF to over 99%. This configuration allows heat storage for night time operation, enhancing operational reliability and water production.
The FO system was designed using hollow fibre membranes, which operate at low pressure (~2–3 bar) and do not require expensive pressure vessels, making it cost-effective.
In practical applications, membrane fouling—caused by biofouling, scaling, and organic deposition—can significantly reduce water flux over time. The system performance depends on how long the membrane maintains a specific flux before cleaning or replacement is required.
• Initial Flux Performance: FO membranes generally exhibit high water flux in the first 24–48 h, depending on feedwater composition and pre-treatment (Cath et al., 2006).
• Flux Decline Over Multiple Cycles: Studies indicate that flux decreases by 10–30% within the first 100–200 h of continuous operation due to organic fouling and scaling (Ghaffour et al., 2013; Linares et al., 2016).
• Mitigation Strategies: Implementing periodic membrane cleaning, and optimizing draw solution concentration can extend membrane lifespan (McGinnis and Elimelech, 2007).
The specific number of cycles or operational hours before reaching a critical flux threshold depends on membrane material, feed water conditions, and system maintenance. Future work should evaluate long-term flux stability under varying operating conditions to optimize system performance.
The desalination system was modelled to produce 1,000 m3/day of fresh water. However, in the non-TES scenario, the FO system’s capacity was increased to 3,000 m3/day to maintain the same daily water output during limited operational hours. Simulations were performed to evaluate system performance at different solar field output temperatures, ranging from 90°C to 160°C.
The study assessed key performance indicators:
• Capacity Factor (CF): Measures system utilization across different configurations.
• Levelized Cost of Thermal Energy (LCoT): Determines the economic feasibility of CSP-driven FO desalination.
• Levelized Cost of Water (LCoW): Evaluates the overall cost-effectiveness of water production.
CF significantly influences both LCoT and LCoW, as a higher CF in the TES scenario reduces the cost impact of capital expenditures over time. Conversely, the lower CF in the non-TES scenario results in higher costs due to underutilization of system capacity.
Jubail in Saudi Arabia, located on the Arabian Gulf coast in the Eastern Province, was selected as the study site due to its high solar irradiance (1662.8 kWh/m2/year, Meteonorm v.7) and existing desalination infrastructure. This ensures realistic assessment conditions for integrating CSP-FO technology within the broader desalination sector in GCC countries.
The desalination process consists of:
• Feed Solution (FS): Pre-treated seawater with a salinity of 45,000 ppm (matching Arabian Gulf conditions), yielding an osmotic pressure of 34.37 bar.
• Draw Solution (DS): DME, with an osmotic pressure of ~120 bar, providing a strong osmotic gradient for water extraction.
• Hollow Fibre FO Membranes: Facilitate water transfer without requiring high hydraulic pressure.
• Regeneration Unit: A stripping tower condenses and recycles evaporated DME for reuse.
Two CSP systems from different manufacturers were used to provide heat for DS regeneration:
• PT System: Uses steam as HTF for high-temperature thermal energy delivery.
• LFR System: Uses thermal oil, ensuring stable and efficient heat transfer over extended periods.
Thermal energy sources were analysed in terms of efficiency, heat loss, and compatibility with FO operation, ensuring optimal performance under varying solar conditions. The properties of the CSP systems are listed in Table 2.
Despite significant advancements in desalination technologies, limited research exists on CSP-FO hybrid systems. Most previous studies have focused on CSP-RO and CSP-MED [(e.g., Mahmoud et al., 2023; Al-Ghamdi et al., 2022)], with few comprehensive evaluations of CSP-FO integration. Given the lower energy footprint of FO compared to RO and its potential for coupling with CSP, this study contributes to bridging this knowledge gap by systematically evaluating cost, scalability, and operational challenges.
The study relies on:
• Solar irradiance data from Meteonorm v.7 to simulate real-world solar field performance.
• Economic data (capital and operational costs) from published CSP and FO desalination cost assessments.
• Sensitivity Analysis: Evaluates the impact of variations in FO membrane costs, CSP efficiency, and TES performance on LCoT and LCoW.
This structured approach ensures a transparent, data-driven evaluation of CSP-FO systems, contributing to the broader field of sustainable desalination research in arid regions.
Heat is exchanged between the FO system and the CSP system through a heat exchanger. Figure 1 shows the interface between the solar field without TES and the FO system, wherein the heat exchanger (HX-1) is used to supply thermal energy to the DS regeneration unit of the FO system. In the CSP with TES case, hot HTF exchanges heat with the HTF in the TES tanks, which in turn would supply heat to the DS regeneration system through a heat exchanger.
The DS regeneration system initially operated at 160°C, with thermal energy requirements ranging from 25 to 35 kWhth/m3 and electrical energy demands of 0.3–0.5 kWh/m3 (based on manufacturer specifications and industry reports). Additional cases were analysed at 140°C and 120°C, allowing a comparative assessment of energy efficiency. The 90°C and 100°C cases were excluded due to reduced thermal regeneration efficiency, which could compromise system performance.
PT systems in this study were assumed to operate without TES. However, TES is commonly used in PT systems in commercial CSP plants, the inclusion of TES in PT systems, while technically feasible, presents significant economic challenges due to the high cost of molten salt or alternative storage media, increased capital investment, and additional operational complexities. In the LFR system, thermal oil was used as HTF, enabling TES to achieve a CF of over 99%. The TES capacity was optimized based on a heat balance model, ensuring sufficient energy storage for overnight FO operation. In non-TES cases, the solar field size was adjusted to maintain a CF between 33.5 and 34.5%.
The FO system was designed to produce 1,000 m3/day of freshwater when operated continuously with TES. In non-TES scenarios, the system operates only 8 h/day, requiring a higher throughput of 3,000 m3/day during operational hours to maintain the same daily water output.
Thermal power output requirements were calculated as follows:
• TES Case: 3,000 m3/day × 25 kWhth/m3 = 3,125 kWth required from CSP.
• Non-TES Case: 1,000 m3/day × 25 kWhth/m3 = 1,042 kWth required from CSP.
These calculations do not consider CSP efficiency losses due to dust accumulation, optical degradation, or seasonal variations, which should be included in future refinements.
The selection of TES and non-TES scenarios was driven by the need to balance capital expenditure (CAPEX) and operational reliability. TES systems provide higher CF but require additional investment, while non-TES systems are cheaper but have limited operational hours. This trade-off is critical in GCC countries, where electricity subsidies, land availability, and solar infrastructure development influence economic feasibility.
By systematically examining these factors, this study contributes to the advancement of sustainable desalination strategies in arid climates while addressing gaps in CSP-FO integration research.
Techno-economic analysis of the CSP-FO system was performed by evaluating the Levelized Cost of Thermal Energy (LCoT) and the Levelized Cost of Water (LCoW). LCoT is calculated as a function of the CAPEX, OPEX and the total thermal energy generated in a year by the CSP system (Equation 1). CAPEX is dependent on the solar field area, the specific solar field cost, the HTF system cost and the TES system cost. OPEX is a function of the operating cost of the solar field and the operating cost of the storage. Solar field costs of small-scale systems are high (see Table 3) and those of large-scale systems get reduced significantly (~150 $/m2).
where the Capital Recovery Factor (CRF) is calculated as Equation 2:
and i is the discount rate (%/year), n is the amortization period in years and k is the yearly insurance rate (%). i was considered as 5%, n as 25 years and k as 0%.
Similarly, the LCoW is calculated as Equation 3:
FO CAPEX includes the FO system (membranes, DS regeneration unit) and the FO OPEX includes chemicals, electricity, thermal power, fixed O & M, and FO membrane replacement. As the thermal power is provided by the CSP system, the LCoT was taken as the cost of thermal energy being supplied to the FO system.
Following Linares et al. (Linares et al., 2016), it was assumed that the FO membranes constitute about 29.4% of the FO CAPEX cost and the cost of membranes was assumed as 450 $/module (equal to commercial RO module cost), 504.5 US$,1 600 US$, 1,000 US$, and 1,500 US$. Furthermore, the chemical costs and fixed O & M costs were considered similar to SWRO systems. The chemical costs were also varied as 0.025 $/m3 (Linares et al., 2016), 0.05 $/m3, 0.075 $/m3, and 0.111 $/m3 to consider variations that could be seen across different locations and conditions. Table 4 lists the costs associated with the FO system.
The integrated CSP-FO system is shown in Figure 1. The Heat Transfer Fluid (HTF) loops can consist of either the PT collectors or the LFR collectors. The number of FO membranes needed are obtained by dividing the production capacity by the flux of individual membranes. The number of membranes needed for 1,000 m3/d are 824 and the number of membranes replaced yearly, considering 5% replacement are 42. Three cases were considered, with the first case consisting of a PT system without TES, the second case consisted of an LFR system without TES and the third case being an LFR system with TES. The CF, the LCoT, the LCoW, the effect of solar field output temperature, the effect of TES and the variation of various components of LCoW due to varying solar field cost, the FO module cost and the TES were evaluated and are presented in the next section.
The PT system was simulated using the online tool provided by PT manufacturer which provides the estimates of energy generated. The LFR simulations were performed using the Repository of Solar Simulation Sources for Industrial Processes (RESSSPI), an on-line simulation tool which gives the cost and energy performance estimates of solar energy systems applied in industrial processes.2 However, only the energy performance estimates were used from RESSSPI, with the techno-economic calculations done in-house. RESSSPI uses an evolution of the simulation engine of SHIPcal (Frasquet, 2016), which has been theoretically validated using TRNSYS.
This section presents the performance and cost analysis of the CSP-FO system under two operational scenarios: without Thermal Energy Storage (TES) (batch mode operation) and with TES (continuous operation). The findings include the impact of CSP system capacity factors, energy utilization, Levelized Cost of Thermal Energy (LCoT), Levelized Cost of Water (LCoW), and the influence of FO module costs and solar field output temperatures on overall system efficiency and economics. Results are structured to improve clarity, using figures and tables to highlight key trends.
The capacity factor (CF) of the CSP systems increases with an increase in the solar field aperture area. However, in the absence of TES, excess solar energy generated beyond system demand is lost due to defocusing. Figure 2 illustrates the CF variation for PT and LFR systems, showing that without TES, a CF of 33.6% is required to meet the daily production demand of 1,000 m3/day within an 8-h operation window.
Figure 2. Capacity factor variation with an increase in the solar field aperture area of the PT and LFR system without TES. The black squares show the PT system and the grey circles represent the LFR system.
Solar energy utilization plays a crucial role in system efficiency. Figure 3 presents the solar radiation, utilized energy, and defocused energy for the LFR system at different months (January, July, and December) with a 17,424 m2 aperture area. Excess energy produced during peak radiation periods must be defocused, reducing overall efficiency.
Figure 3. Solar radiation, utilized energy and defocussed energy in the LFR system at 17,424 m2 (110 × 6 collectors) solar aperture area during the months of January, July and December. TES was not considered (FO run in batch mode). Solar radiation is shown on the secondary y-axis.
To further illustrate the effect of system sizing, Figure 4 compares the defocused energy for a smaller solar field aperture area (12,672 m2). This demonstrates that improper sizing leads to substantial energy losses, emphasizing the importance of optimized field design.
Figure 4. Solar radiation, utilized energy and defocussed energy in the LFR CSP system at 12,672 m2 (80 × 6 collectors) solar aperture area during the months of January, July and December. TES was not considered (FO run in batch mode). Solar radiation is shown on the secondary y-axis. This indicates that the aperture area selection with operation hours is well suited with required CF.
Thermal energy costs significantly influence desalination economics. Figure 5 shows the LCoT and LCoW trends for PT and LFR systems at different aperture areas.
• At low aperture areas, LCoT remains low due to minimal energy wastage.
• As aperture area increases, LCoT rises as more energy is defocused.
• The lowest LCoW for LFR is $0.875/m3, corresponding to an LCoT of 1.67 c$/kWhth, but the capacity factor at this point is only 25.09%, making it impractical.
• At 33.6% CF, the LCoT and LCoW for LFR are 2.03 c$/kWhth and $0.93/m3, respectively. For PT, the values are 1.71 c$/kWhth and $0.83/m3, showing PT achieves a lower LCoW than LFR at a lower aperture area.
Figure 5. (a) LCoT and LCoW for the LFR system (specific solar field cost 150 $/m2 and FO module cost 504.5 $, solar field output temperature 160°C). (b): LCoT and LCoW for the PT system (specific solar field cost 150 $/m2 and FO module cost 504.5 $, solar field output temperature 160°C).
In the TES case, the system operates 24/7, significantly improving the CF but adding storage-related costs. Figure 6 illustrates how CF increases with larger TES storage volumes (2,000 m3, 4,000 m3, 4,500 m3), demonstrating diminishing returns beyond a certain TES size.
Figure 6. (a) CF and TES variation for LFR when the TES volume is changed. TES is shown on the secondary vertical axis and is represented by markers and lines in red color. CF is shown using the primary vertical axis and values are represented by markers and lines in black colour. (b) LCoT for LFR is shown using black and grey markers for different TES volumes and aperture areas. CFs are shown in red. (c) LCoW for LFR is shown using black and blue markers for different TES volumes and aperture areas. CFs are shown in red (specific solar field cost 150 $/m2 and FO module cost 504.5 $, solar field output temperature 160°C).
For TES storage of 4,500 m3 and a CF of 99.51%, the LCoT is 7.69 c$/kWhth and the corresponding LCoW is $2.19/m3. Although CF can be pushed to 100% by increasing the aperture area, this results in excessive energy losses due to defocusing, leading to increased LCoT.
The FO module cost is a key determinant in LCoW composition. Figure 7 illustrates how variations in FO module pricing affect the cost distribution in the PT CSP-FO system without TES:
• When FO module cost is low ($450/module), thermal energy cost dominates LCoW (68%).
• As FO module cost increases ($1,500/module), thermal cost contribution drops to 29%, making FO CAPEX the largest factor.
Figure 7. PT CSP-FO case with no TES (FO run in batch mode) at 160°C solar field output. (a) % LCoW components at a variable FO element cost and 300 $/m2 solar field cost. (b) % LCoW components at 504.5 $/FO (see Table 4) element and 300 $/m2 solar field cost (c) % LCoW components at 1,500 $/FO element and 150 $/m2 solar field cost.
A similar trend is observed for LFR (Figure 8), but LFR systems exhibit slightly higher O&M thermal costs due to increased system expenses.
Figure 8. LFR CSP-FO case with no TES (FO run in batch mode) at 160°C solar field output. (a) % LCoW components at a variable FO element cost and 355 $/m2 solar field cost. (b) % LCoW components at 504.5 $/FO element and 355 $/m2 solar field cost (c) % LCoW components at 1500 $/FO element and 150 $/m2 solar field cost.
In continuous-mode FO operation (TES scenario), thermal energy remains the largest cost component. Figure 9 illustrates:
• Even with FO module costs of $1,500/module, thermal energy constitutes 83.5% of LCoW, indicating that TES costs are a major economic barrier.
• Reducing TES costs is necessary to improve economic feasibility.
Figure 9. LFR CSP-FO case with TES at 160°C solar field output. (a) % LCoW components at a variable FO element cost and 355 $/m2 solar field cost. (b) % LCoW components at a variable FO element cost and 150 $/m2 solar field cost. (c) % LCoW components at 504.5 $/FO element and 355 $/m2 solar field cost (d) % LCoW components at 1500 $/FO element and 150 $/m2 solar field cost.
The solar field output temperature influences CF, LCoT, and LCoW. Figure 10 presents the effect of varying output temperatures (160°C, 140°C, 120°C) on LCoW:
• For non-TES cases (PT & LFR): LCoW decreases as temperature drops due to improved thermal efficiency.
• For TES cases (LFR only): Temperature variations have minimal impact, suggesting that higher TES storage volumes neutralize efficiency gains from lower operating temperatures.
Figure 10. (a) Influence of solar field cost and the solar field output temperature on the LCoW in the PT non-TES case. (b) Influence of solar field cost and the solar field output temperature on the LCoW in the LFR non-TES case. (c) Influence of solar field cost and the solar field output temperature on the LCoW in the LFR with TES case.
Key findings of this study are summarized in Table 5.
While TES enables continuous operation, it introduces prohibitive storage costs, making non-TES configurations more viable for regions where batch-mode desalination aligns with water demand patterns. The study also highlights that FO module cost plays a significant role in determining LCoW, with thermal energy costs dominating at lower module prices and amortized FO CAPEX becoming the primary cost factor as module prices increase.
Compared to existing literature on CSP-driven desalination, these findings align with studies that emphasize the economic burden of TES in solar thermal applications but diverge in demonstrating that lower solar field temperatures in non-TES scenarios contribute to better cost efficiency. The minimal impact of temperature variations in TES cases supports prior studies indicating that storage costs overshadow efficiency gains in high-CF CSP configurations.
• Cost Reduction Strategies for TES: Future work should explore alternative storage materials and hybrid storage solutions to mitigate the cost penalty of TES.
• Hybridization with PV Systems: Investigating CSP-PV-FO hybrid systems may provide a more cost-effective approach, leveraging PV for electricity generation while maintaining CSP for thermal regeneration.
• Optimization of FO Membranes: Research should focus on enhancing membrane longevity and performance, particularly in terms of reducing energy losses associated with draw solution regeneration.
• Economic Sensitivity Analyses: Further economic modelling incorporating real-world financial parameters (e.g., discount rates, subsidies, operational contingencies) is needed to validate the long-term feasibility of CSP-FO desalination.
Overall, this study provides a comprehensive evaluation of CSP-FO integration, identifying key trade-offs between cost, efficiency, and scalability while outlining actionable research pathways for making CSP-driven desalination a commercially viable solution for arid regions.
The original contributions presented in the study are included in the article/supplementary material, further inquiries can be directed to the corresponding author.
SA: Conceptualization, Data curation, Formal analysis, Investigation, Software, Validation, Visualization, Writing – original draft. AA: Conceptualization, Data curation, Funding acquisition, Investigation, Methodology, Resources, Validation, Writing – original draft. AM: Conceptualization, Investigation, Methodology, Project administration, Writing – review & editing. HA: Validation, Writing – review & editing. JA: Project administration, Resources, Writing – review & editing.
The author(s) declare that no financial support was received for the research and/or publication of this article.
The authors declare that the research was conducted in the absence of any commercial or financial relationships that could be construed as a potential conflict of interest.
The authors declare that no Gen AI was used in the creation of this manuscript.
All claims expressed in this article are solely those of the authors and do not necessarily represent those of their affiliated organizations, or those of the publisher, the editors and the reviewers. Any product that may be evaluated in this article, or claim that may be made by its manufacturer, is not guaranteed or endorsed by the publisher.
FO, Forward Osmosis; RO, Reverse Osmosis; DS, Draw Solution; FS, Feed Solution; DME, Di-Methyl Ether; PT, Parabolic Trough; LFR, Linear Fresnel Reflector; HTF, Heat Transfer Fluid; CSP, Concentrated Solar Power; c$, cents; LCoT, Levelized Cost of Thermal energy; LCoW, Levelized Cost of Water; kWht, kilo Watt hour thermal.
Abdelrazik, A. S., Osama, A., Allam, A. N., Shboul, B., and Sharafeldin, M. A. (2023). M. Elwardany and A. M. Masoud (2023) ANSYS-fluent numerical modeling of the solar thermal and hybrid photovoltaic-based solar harvesting systems. J. Therm. Anal. Calorim. 148, 11373–11424. doi: 10.1007/s10973-023-12509-2
Abdelrazik, A. S., Sharafeldin, M. A., Elwardany, M., Abdulkawy, N., Shboul, B., Ezzat, S. M., et al. (2024). Effect of design and operation parameters on solar-driven membrane-based desalination systems: an overview. WIREs Energy Environ. 13:e521. doi: 10.1002/wene.521
Al-Ghamdi, A. S., Mahmoud, A. M., and Bamardouf, K. (2022). Prospect of utilization of solar energy in SWCC existing multi-effect desalination satellite plants. Desalin. Water Treat. 263, 9–14. doi: 10.5004/dwt.2022.28195
Alghassab, M. A. (2024). A review of hybrid solar desalination systems: structure and performance. Water Sci. Technol. 89, 1357–1381. doi: 10.2166/wst.2024.042
Atanasovska, A.. (2016) Solar Desalination: A Promising Solution for the Future of Water in the MENA Region. Available online at: https://water.fanack.com/publications/desalination/ (Accessed March 6, 2025).
Bartels, C. R., Wilf, M., Andes, K., and Iong, J. (2008). Design considerations for wastewater treatment by reverse osmosis. Water Sci. Technol. 8, 625–632. doi: 10.2166/ws.2008.110
Cai, Y., and Hu, X. M. (2016). A critical review on draw solutes development for forward osmosis. Desalination 391, 16–29. doi: 10.1016/j.desal.2016.03.021
Cai, Y., Shen, W., Wei, J., Chong, T. H., Wang, R., Krantz, W. B., et al. (2015). Energy-efficient desalination by forward osmosis using responsive ionic liquid draw solutes. Environ. Sci. 1, 341–347. doi: 10.1039/C4EW00073K
Cath, T. Y., Childress, A. E., and Elimelech, M. (2006). Forward osmosis: principles, applications, and recent developments. J. Membr. Sci. 281, 70–87. doi: 10.1016/j.memsci.2006.05.048
Colciaghi, R., Simonetti, R., Molinaroli, L., Binotti, M., and Manzolini, G. (2021). Potentialities of thermal responsive polymer in forward osmosis (FO) process for water desalination, desalination. Desalination 519:115311. doi: 10.1016/j.desal.2021.115311
Elwardany, M., Abdelrazik, A. S., Fathi, H., Omar, A. M. A., and Abdelkawy, N. (2024). Practicality and economic assessment on using the solar organic Rankine cycle as a power source for a specific membrane-based desalination system. Water Conserv. Sci. Eng. 9:46. doi: 10.1007/s41101-024-00273-9
Foo, Z. H., Deshmukh, A., Wilson, A. D., and Lienhard, J. H. (2024). Harnessing dimethyl ether with ultra-low-grade heat for scaling-resistant brine concentration and fractional crystallization. Chem. Eng. J. 489:151159. doi: 10.1016/j.cej.2024.151159
Frasquet, M. (2016). SHIPcal: solar heat for industrial processes online calculator. Energy Procedia 91, 611–619. doi: 10.1016/j.egypro.2016.06.213
Ghaffour, N., Missimer, T. M., and Amy, G. L. (2013). Technical review and evaluation of the economics of water desalination: current and future challenges for better water supply sustainability. Desalination 309, 197–207. doi: 10.1016/j.desal.2012.10.015
Linares, R. V., Li, Z., Yangali-Quintanilla, V., Ghaffour, N., Amy, G., Leiknes, T., et al. (2016). Life cycle cost of a hybrid forward osmosis - low pressure reverse osmosis system for seawater desalination and wastewater recovery. Water Res. 88, 225–234. doi: 10.1016/j.watres.2015.10.017
Lovegrove, K., and Stein, W. (2012). Concentrating solar power technology: Principles, developments and applications. Sawston, Cambridge: Woodhead Publishing.
Mahmoud, A. M., Al-Ghamdi, A. S., and Ahmed, S. (2023). Enhancing the efficiency of a CSP assisted MED-TVC desalination system. Desalin. Water Treat. 309, 119–125. doi: 10.5004/dwt.2023.29889
McCutcheon, J. R., McGinnis, R. L., and Elimelech, M. (2005). A novel ammonia–carbon dioxide forward (direct) osmosis desalination process. Desalination 174, 1–11. doi: 10.1016/j.desal.2004.11.002
McGinnis, R. L., and Elimelech, M. (2007). Energy requirements of ammonia-carbon dioxide forward osmosis desalination. Desalination 207, 370–382. doi: 10.1016/j.desal.2006.08.012
McMillan, C. A., Ruth, M. F., and Brancaccio, D. (2023). Renewable thermal energy systems: modeling developments and systemic challenges. Golden, CO: National Renewable Energy Laboratory (NREL).
Moharram, N. A., Bayoumi, S., Hanafy, A. A., and El-Maghlany, W. M. (2021). Techno-economic analysis of a combined concentrated solar power and water desalination plant. Energy Convers. Manag. 228:113629. doi: 10.1016/j.enconman.2020.113629
Monjezi, A. A., Mahood, H. B., and Campbell, A. N. (2017). Regeneration of dimethyl ether as a draw solute in forward osmosis by utilising thermal energy from a solar pond. Desalination 415, 104–114. doi: 10.1016/j.desal.2017.03.034
Pocock, J., Muzhingi, A., Mercer, E., Velkushnova, K., Septien, S., and Buckley, C. A. (2022). Water and nutrient recovery from stored urine by forward osmosis with an ammonium bicarbonate draw solution. Front. Environ. Sci. 10:937456. doi: 10.3389/fenvs.2022.937456
Sato, N., Sato, Y., and Yanase, S. (2014). Forward osmosis using dimethyl ether as a draw solute. Desalination 349, 102–105. doi: 10.1016/j.desal.2014.06.028
Tiwari, A., Rathod, M. K., and Kumar, A. (2022). A comprehensive review of solar-driven desalination systems and its advancements. Environ. Dev. Sustain. 25, 1052–1083. doi: 10.1007/s10668-021-02040-5
Vision 2030. (2016). National Transformation Program. Available online at: https://www.vision2030.gov.sa/en/explore/programs/national-transformation-program (Accessed March 5, 2025).
Xie, M., Lee, J., Nghiem, L. D., Price, W. E., and Elimelech, M. (2015). Effects of feed and draw solution temperature and transmembrane temperature difference on the rejection of trace organic contaminants by forward osmosis. J. Membr. Sci. 453, 292–301. doi: 10.1016/j.memsci.2013.10.070
Zeng, L.-M., Du, M.-Y., and Wang, X.-L. (2017). A Thermodynamical approach for evaluating energy consumption of the forward osmosis process using various draw solutes. Water 9:189. doi: 10.3390/w9030189
Keywords: forward osmosis, concentrated solar power, thermal energy storage, parabolic trough, linear Fresnel
Citation: Ahmed S, Alghamdi AS, Mahmoud AM, Albutuwaybh HH and Almadeh JA (2025) Concentrating solar power (CSP) assisted FO hybrid systems for desalination of seawater: a preliminary study. Front. Water. 7:1564842. doi: 10.3389/frwa.2025.1564842
Received: 22 January 2025; Accepted: 14 March 2025;
Published: 09 April 2025.
Edited by:
Shailendra Kumar Shukla, Banaras Hindu University, IndiaReviewed by:
Vikash Kumar Chauhan, Indian Institute of Technology Bombay, IndiaCopyright © 2025 Ahmed, Alghamdi, Mahmoud, Albutuwaybh and Almadeh. This is an open-access article distributed under the terms of the Creative Commons Attribution License (CC BY). The use, distribution or reproduction in other forums is permitted, provided the original author(s) and the copyright owner(s) are credited and that the original publication in this journal is cited, in accordance with accepted academic practice. No use, distribution or reproduction is permitted which does not comply with these terms.
*Correspondence: Sultan Ahmed, c2FobWVkN0Bzd2NjLmdvdi5zYQ==
Disclaimer: All claims expressed in this article are solely those of the authors and do not necessarily represent those of their affiliated organizations, or those of the publisher, the editors and the reviewers. Any product that may be evaluated in this article or claim that may be made by its manufacturer is not guaranteed or endorsed by the publisher.
Research integrity at Frontiers
Learn more about the work of our research integrity team to safeguard the quality of each article we publish.