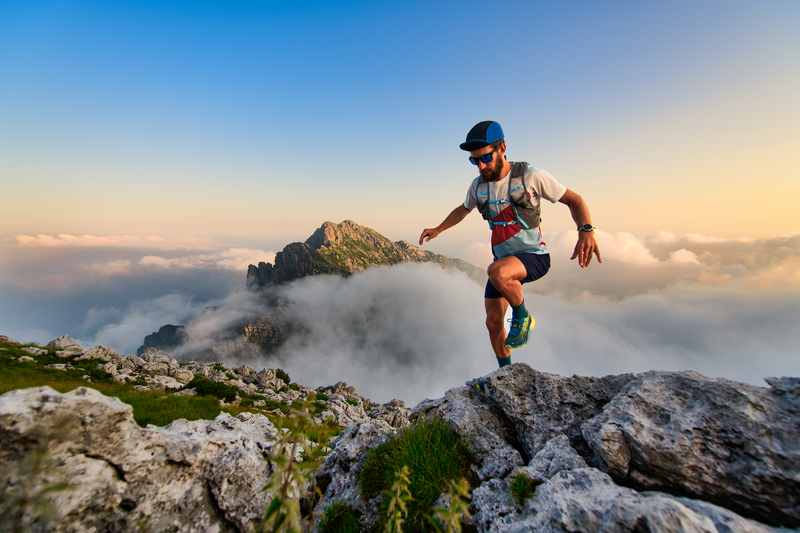
95% of researchers rate our articles as excellent or good
Learn more about the work of our research integrity team to safeguard the quality of each article we publish.
Find out more
MINI REVIEW article
Front. Water , 31 March 2025
Sec. Water and Critical Zone
Volume 7 - 2025 | https://doi.org/10.3389/frwa.2025.1496199
This article is part of the Research Topic Investigating Connectivity to Advance the Predictive Understanding of Watershed Processes and the Earth’s Critical Zone View all 8 articles
This review synthesizes methods for measuring, modeling, and managing hydrologic connectivity, offering pathways to improve practices and address environmental challenges (e.g., climate change) and sustainability. As a key driver of water movement and nutrient cycling, hydrologic connectivity influences flood mitigation, water quality regulation, and biodiversity conservation. However, traditional field-based methods (e.g., dye tracing), indirect measurements (e.g., runoff analysis), and remote sensing techniques (e.g., InSAR) often struggle to capture the complexity of catchment-scale interactions. Similarly, modeling approaches—including process-based and percolation theory-based models, graph theory, and entropy-based metrics—face limitations in fully representing these interconnected processes. Both modeling and measurement techniques are constrained by inadequate spatial and temporal coverage, high data demands, computational complexity, and difficulties in representing subsurface connectivity. Subsequently, we critique current management practices that prioritize isolated variables (e.g., streamflow, sediment transport) over system-wide strategies and emphasize the need for adaptive, connectivity-based approaches in water resource planning and restoration. Moving forward, we highlight the importance of interdisciplinary collaboration, technological innovations (e.g., AI-driven modeling, real-time monitoring), and integrated frameworks to improve connectivity measurement, modeling, and adaptive management to restore fragmented hydrologic networks. This integrated approach sets the stage for transformative water resource management, fostering proactive policy development and stakeholder engagement.
Hydrologic connectivity is defined as the water-mediated transfer of matter, energy, and organisms within or between elements of the hydrologic cycle (Pringle, 2001). It is a cornerstone of catchment science, governing the movement of water, nutrients, and organisms across subsurface areas, surface layers, river streams, and wetlands (Bracken et al., 2013; Zhang et al., 2021). As an emergent property shaped by complex ecological interactions—including hydrological, biogeochemical, and geomorphological processes—connectivity facilitates critical exchanges within and between ecosystems (Lehmann et al., 2007; Wohl et al., 2018; Harvey and Gooseff, 2015). Understanding hydrologic connectivity is essential for predicting water movement in response to climate variability, land-use changes, and increasing anthropogenic pressures on water resources. It plays an indispensable role in water resource management, directly influencing flood and drought mitigation (Maxwell et al., 2021), regulating water quality (Harvey et al., 2019; Fox et al., 2022), and maintaining vital biological habitats (Pringle, 2001). Hydrologic connectivity enhances system resilience by regulating water flow, reducing erosion, and supporting habitat restoration (Fortuna et al., 2006; Rains et al., 2016). It also sustains natural purification processes by enabling wetlands to filter pollutants through sediment trapping and microbial breakdown, mitigating the impacts of urbanization and deforestation (Haarstad et al., 2012; Lane et al., 2018; Bertassello et al., 2022; Fang et al., 2024).
To better understand hydrologic connectivity and its role in catchment dynamics, it is categorized in two ways: by spatial domains (system perspective) and by connectivity dimensions (connectivity types). The system perspective categorizes hydrologic connectivity based on spatial domains—surface, surface-subsurface, and subsurface—highlighting where exchanges occur. Surface connectivity governs the movement of water, sediments, and nutrients across landscapes via rivers, streams, and overland flow. Surface-subsurface connectivity represents interactions between surface water and groundwater, such as infiltration, percolation, and hyporheic exchange, which regulate groundwater recharge and solute transport. Subsurface connectivity describes water and solute flow within soil and groundwater, influencing aquifer recharge and groundwater-surface exchanges (Covino, 2017). These categories are not mutually exclusive, as interactions between surface and sub-surface processes regulate catchment responses across spatial scales.
In contrast, hydrologic connectivity types are classified based on the connectivity dimensions that characterize water movement within and between systems—including longitudinal, lateral, and vertical connectivity—each of which uniquely shapes catchment dynamics. These connectivity types influence functional processes; for example, longitudinal connectivity facilitates the downstream transport of water, organisms, sediments, and nutrients, ensuring both hydrological and ecological continuity along river networks (Buddendorf et al., 2017; Lee S. et al., 2023). Lateral connectivity links rivers to adjacent floodplains, wetlands, and groundwater zones, facilitating nutrient cycling, habitat exchange, and floodplain dynamics (Leibowitz et al., 2018). Vertical connectivity governs surface–sub-surface water and solute exchanges, regulating groundwater recharge and biogeochemical processes.
Within surface and subsurface systems (i.e., the system perspective), hydrologic connectivity is shaped by structural and functional drivers (Turnbull et al., 2008). Structural drivers, such as topography and vegetation patterns, remain relatively static over short timescales, while functional drivers involve transient hydrologic processes, such as overland flow dynamics or storm-driven flow path formation. These structural and functional drivers regulate hydrologic exchanges across spatial domains, influencing the movement of water, solutes, and sediments throughout the catchment.
To capture the influence of transient hydrologic processes, some studies also consider temporal connectivity, which describes fluctuations in water movement driven by seasonal variations, precipitation events, and disturbances. These changes influence surface, subsurface, and vertical exchanges, shaping river discharge, sediment transport, and wetland hydrodynamics (Pringle, 2001; Lane et al., 2018; Lee E. et al., 2023; Wu et al., 2023). Recognizing the role of time in shaping hydrological connectivity, Ward (1989) introduced the four-dimensional perspective of lotic ecosystems, which conceptualizes their dynamic and hierarchical nature through longitudinal (upstream-downstream), lateral (channel-floodplain), and vertical (channel-groundwater) interactions, with time as the fourth dimension providing the temporal scale that governs ecosystem responses to disturbances.
Seminal works have shaped the understanding of various aspects of hydrologic connectivity (for a detailed list, see Pöppl et al., 2024). Key contributions by Schumm (1965), Taylor et al. (1993), Fryirs et al. (2007), Bracken and Croke (2007), Poeppl et al. (2017), and Wohl et al. (2019) have established connectivity as a driver of energy and material transfer, influencing sediment transport, landscape evolution, and hydrological regulation. For instance, Poeppl et al. (2017) proposed a framework integrating human impacts on fluvial systems, highlighting feedback loops between social and geomorphic systems. Similarly, Taylor et al. (1993) pioneered the concept of landscape connectivity, demonstrating its role in species dispersal and source-sink dynamics and urging planners to consider animal movement in conservation strategies. Despite these advancements, significant gaps remain in understanding and managing hydrologic connectivity, particularly in foundational knowledge, methodological development, and management applications.
Effective resource management and predictive modeling depend on a robust understanding of hydrologic connectivity, which requires bridging knowledge gaps in process interactions. Studies highlight the challenges posed by spatial and temporal scale variations, as hydrologic systems exhibit nonlinear behaviors and complex flow pathways (Lehmann et al., 2007; Wohl et al., 2018). Moreover, hydrologic connectivity is deeply intertwined with ecological systems, yet many studies fail to consider these linkages, emphasizing the need for interdisciplinary approaches integrating hydrology, ecology, geomorphology, and biogeochemistry (Bracken et al., 2013; Covino, 2017; Yu et al., 2023).
Accurate assessments of hydrologic connectivity require standardized methodologies to ensure consistent evaluations across spatial and temporal scales (Turnbull et al., 2018). Traditional techniques often fail to capture subsurface and dynamic flow complexities, while graph theory and entropy-based metrics offer novel solutions (Zuecco et al., 2019; Tejedor et al., 2015). Existing models frequently over-rely on static metrics, neglecting the real-time dynamic behaviors essential for managing subsurface flow and transport (Renard and Allard, 2013). Furthermore, the absence of standardized metrics has hindered collaboration and integration across research disciplines (Zhang et al., 2021; Bracken et al., 2013).
Many management applications need to adopt adaptive, connectivity-based approaches, resulting in significant gaps in managing hydrological systems and ensuring ecosystem resilience amid climate variability and human-induced changes (Poeppl et al., 2017; Keesstra et al., 2018; Poeppl et al., 2020; Aho et al., 2020; Roy, 2023; Herzog et al., 2024). Although the importance of such strategies is well-recognized, Lexartza-Artza and Wainwright (2009, 2011) emphasize the need for adaptive methods to manage sediment and water interactions whereas Wainwright et al. (2011) highlight the complex interplay of environmental regimes across spatial and temporal scales. Incorporating hydrologic connectivity is vital for global water resource management and climate adaptation. The absence of such approaches weakens flood mitigation, groundwater recharge management, and ecosystem conservation efforts, leading to increased risks of habitat loss and water quality degradation (Good et al., 2015; Tejedor et al., 2015; Maxwell et al., 2021; Chen et al., 2021; Li et al., 2024).
Given hydrologic connectivity's complexities and interdisciplinary nature, this mini-review synthesizes current knowledge on its spatial and temporal dynamics, methodological advancements, and implications for catchment dynamics, connectivity restoration, and water resource management amid climate change and human activities. While analyzing connectivity by system, type, or temporal scale provides a structured approach, its full impact on catchment dynamics emerges only when considered holistically. Therefore, this review examines how an integrated approach can enhance measurement, modeling, and management strategies (Figure 1).
Figure 1. Hydrologic connectivity: advancing measurement techniques, interdisciplinary integration, and management strategies to deepen understanding and optimize water-mediated transfers in the hydrologic cycle, which are vital for river ecosystem health and critical for ensuring the sustainability and resilience of water resources in the face of environmental change.
This section explores measurement techniques and modeling approaches, evaluates their strengths and limitations, and highlights the need for further advancements. We organize the following section around system perspective because it provides a tangible framework for measuring connectivity by integrating spatial and temporal scales and capturing multiple types (e.g., longitudinal, lateral, and vertical) across surface and subsurface systems. Below, we explore measurement techniques tailored to different systems, evaluating their strengths and limitations.
Hydrologic connectivity measurement techniques fall into field-based methods, proxies, and remote sensing techniques (Figure 1). While field-based methods and proxies can characterize connectivity in surface and subsurface systems, remote sensing techniques primarily assess surface connectivity, though some applications can indirectly infer subsurface changes.
Among these approaches, field-based methods provide direct, high-resolution measurements of water movement, making them essential for understanding localized connectivity dynamics (e.g., Zimmer et al., 2020). For instance, dye tracing involves introducing a tracer dye into a water source to track flow pathways and residence times. This approach provides detailed insights into water movement and surface-subsurface interactions, particularly in wetlands and rivers (Zhang et al., 2022). Although highly precise, these methods are labor-intensive, temporally limited, and constrained in spatial coverage. These limitations reduce their effectiveness for large-scale assessments.
Proxies offer valuable tools for inferring hydrological connectivity by measuring environmental variables (Zhang et al., 2022). In surface systems, runoff patterns provide critical insights into hydrologic connectivity (Bracken and Croke, 2007). In sub-surface systems, soil moisture and plant distribution are key factors in assessing subsurface hydrologic connectivity (Yang et al., 2023). Beyond subsurface flow, they also regulate surface runoff, modifying surface connectivity (Liu et al., 2019). This interdependence reinforces that surface and sub-surface systems are not mutually exclusive but dynamically linked.
Sediment connectivity provides a more integrated perspective by linking sediment transport and retention across surface and sub-surface systems (Drummond et al., 2014; Olliver et al., 2020). Surface connectivity is assessed by measuring sediment transport through runoff, river channels, and overland flow (Julien and Simons, 1985; Prosser et al., 1995). For example, Bracken and Croke (2007) highlight hydrological connectivity in runoff-dominated systems, showing its influence on erosion and sedimentation. Building on this, Borselli et al. (2008) demonstrate using Geographic Information System (GIS) and field assessments to explore sediment and flow connectivity. GIS-based approaches, particularly those utilizing digital elevation models (DEMs), calculate connectivity indices that quantify flow pathways and identify areas of high and low connectivity based on topographic features (Borselli et al., 2008; Heckmann et al., 2018). Subsequently, Bracken et al. (2015) propose a sediment connectivity framework to understand sediment transfer across scales, directly influencing landscape stability. In contrast, subsurface connectivity is evaluated by examining sediment movement within soils, hyporheic zones, and groundwater pathways, emphasizing the complex interactions between surface and subsurface processes (Harvey et al., 2012; Lewandowski et al., 2015; Somers et al., 2016).
Complementing these approaches, remote sensing techniques, such as InSAR, provide large-scale, high-resolution monitoring of surface water connectivity, making them particularly useful for assessing fragmented landscapes (Liu D. et al., 2020). InSAR relies on satellite-based radar to detect surface elevation changes, enabling broad-scale monitoring of water movement and identifying fragmented or connected areas in river networks. While remote sensing methods primarily capture surface connectivity, some techniques (e.g., gravity-based satellite data like GRACE) can infer subsurface storage changes (Tapley et al., 2004). Optical imagery and LiDAR complement these approaches by detecting land cover changes, vegetation dynamics, and erosion patterns, indirectly influencing connectivity. However, remote sensing methods typically provide surface-level snapshots and often lack the resolution needed to represent subsurface dynamics accurately (Ameli and Creed, 2017).
Modeling approaches for hydrologic connectivity can be categorized into process-based models (for either surface or subsurface systems), network and graph-based models, and integrated models (Figure 1). While all these approaches can characterize surface and sub-surface connectivity, integrated models uniquely link surface and sub-surface processes, explicitly capturing feedback mechanisms at their interface.
Process-based models, such as hydrodynamic models (Liu Y. et al., 2020; Yang et al., 2021) and percolation theory (Lehmann et al., 2007), simulate physical flow processes and water quality across different spatial and temporal scales. Hydrodynamic models provide detailed simulations of water movement, including flow velocities, flood dynamics, and water quality, making them valuable for localized analyses of both surface and subsurface systems (e.g., Baram et al., 2013; Siqueira et al., 2018). However, they are highly data-intensive, sensitive to parameter errors, and often limited in scalability to larger systems (e.g., Rimon et al., 2011; Siqueira et al., 2018).
Percolation models focus on flow pathways and connectivity thresholds in porous media, primarily addressing subsurface systems (e.g., Lehmann et al., 2007; Rimon et al., 2011). They can be applied probabilistically to analyze runoff thresholds or identify key flow characteristics statistically. While effective for modeling nonlinear flow behaviors in hillslope hydrology and identifying significant runoff thresholds, percolation theory often oversimplifies hydrological systems by neglecting biogeochemical interactions critical for ecosystem management (Lehmann et al., 2007; Dahan et al., 2009).
Network and graph-based models, including graph-theoretic models (Tejedor et al., 2015) and entropy-based metrics (Tejedor et al., 2015), represent hydrologic systems as networks, where nodes and edges depict connected water bodies. This enables clear visualization and quantification of connectivity across river basins and delta channels (Passalacqua, 2017; Garbin et al., 2019). These models effectively assess structural connectivity (Bertassello et al., 2020; Xingyuan et al., 2023; Huang et al., 2024) but require extensive input data and primarily focus on static representations (Durighetto et al., 2023), often overlooking dynamic hydrological behaviors, particularly during extreme events (Beven, 2012).
Entropy-based metrics integrate both topological and dynamic complexity, making them valuable for evaluating system vulnerabilities and resilience. They quantify system disorder and assess dynamic connectivity under varying hydrological conditions, such as flood pulses or droughts, and have been widely applied in hydrologic connectivity and environmental sciences (e.g., Dwivedi and Mohanty, 2016; Arora et al., 2019; Bennett et al., 2019). While these methods can characterize subsurface connectivity, they are more suited for surface connectivity due to the natural network structure of river streams and the greater availability of surface data. However, subsurface connectivity can be analyzed using fracture networks or model outputs with sufficient data. Nonetheless, their computational complexity and lack of standardized metrics limit their broader applicability across diverse landscapes.
Integrated models couple sub-surface and surface hydrology while incorporating land surface processes (e.g., energy fluxes, soil moisture, runoff) and ecohydrology (e.g., plant-water interactions, ecological responses) (e.g., Coon and Shuai, 2022; Xu et al., 2022; Shuai et al., 2022; Ackerer et al., 2023). By simulating hydrological, climatic, and ecological interactions, these models provide a comprehensive understanding of water dynamics, energy exchange, and biogeochemical cycles across scales. These models capture hydrologic connectivity across surface, subsurface, and interface domains, distinguishing longitudinal, vertical, and lateral connectivity (Mikkelson et al., 2013; Camporese et al., 2019). Their ability to simulate biogeochemical processes aids in understanding catchment dynamics and tracing solute sources, differentiating biogenic and geogenic contributions in river systems (Dwivedi et al., 2018b; Arora et al., 2016; Godsey et al., 2019). They are also valuable for managing flood flows and enhancing ecosystem resilience, especially in sensitive regions like drylands (Şensoy et al., 2018).
Their ability to integrate ecohydrological interactions makes them highly relevant for interdisciplinary applications. They can leverage modular studies, such as ecohydrological frameworks (Maxwell et al., 2021; Van Meerveld et al., 2021) and dimensionless river connectivity metrics (Harvey et al., 2019), to predict outcomes like habitat connectivity and species distribution based on water availability and flow patterns.
These data-intensive models struggle to represent complex ecological-hydrological relationships due to the need for distributed parameters (Chen et al., 2021). Their computational demands limit global applicability and often lack adaptive management integration, reducing flexibility in changing environmental conditions.
A comprehensive understanding of hydrologic connectivity requires integrating field-based methods, relevant state variables (proxies), remote sensing, and advanced modeling approaches. As discussed above, field methods are accurate but labor-intensive, while remote sensing offers broad coverage but lacks subsurface insights. Modeling translates these measurements into predictions: process-based models provide detailed simulations but demand extensive data and computing power; network models capture structure but struggle with dynamics and subsurface flow; integrated models offer the most comprehensive view but are data-intensive and computationally demanding. Advancing hydrologic connectivity assessments in the future requires improving data availability, scalability, dynamic modeling, and interdisciplinary integration.
Catchment systems are shaped by a complex interplay of processes (Figure 1), making interdisciplinary integration—across hydrology, ecology, biogeochemistry, and geomorphology—essential for both understanding hydrologic connectivity and developing effective restoration strategies that enhance ecosystem functioning (e.g., Hubbard et al., 2018). This approach provides deeper insights into how water movement affects key biogeochemical cycles, such as carbon and nitrogen cycling, which are vital for sustaining ecosystem health. For example, integrating geomorphological insights accounts for terrain evolution and sediment dynamics while incorporating biogeochemical processes captures feedback between water flow and nutrient availability (Liu D. et al., 2020). Appling et al. (2014) demonstrate the critical role of integrating geomorphology and hydrology, showing how the geomorphic history of floodplains influences nutrient movement and transformation through landscape structure and flow paths. The interaction between hydrologic flow and geomorphic configuration plays a crucial role in shaping water residence times and flow pathways (Helton et al., 2014). Integrating hydrology and biogeochemistry further enhances our understanding of nutrient cycling in river systems (Liu D. et al., 2020).
In the context of ecology, hydrologic connectivity plays a vital role in shaping biodiversity and ecosystem resilience. Ecological dynamics, such as species migration and habitat access, further refine spatial and temporal scales of connectivity, identifying critical thresholds for habitat connectivity in floodplain systems (Stoffers et al., 2022). Stoffers et al. (2022) underscore how fish populations in floodplain rivers depend on habitat connectivity, facilitating migration and access to critical habitats. Likewise, Uno et al. (2022) show that aquatic communities in floodplains reflect varying degrees of hydrological connectivity, highlighting the importance of integrating ecological and hydrological research to understand species' distributions and community structure. These studies highlight the need for interdisciplinary approaches to accurately model hydrological and biogeochemical interactions, improving calibration and predictions of nutrient transport and biodiversity responses to climate-driven hydrologic connectivity changes.
Successful interdisciplinary collaborations have led to significant breakthroughs in understanding hydrologic connectivity (Turnbull et al., 2018; iConn Network, 2024). For instance, the collaboration between hydrologists and biogeochemists has helped address the “old water paradox” in hydrology (Kirchner, 2003), where streamflow is often found to contain a significant proportion of older water. Using geochemical tracers, as Cartwright and Morgenstern (2012) describe, has enabled researchers to unravel the sources of this older water, which is critical for understanding the dynamics between surface and subsurface hydrological systems. Similarly, collaboration between geomorphologists and hydrologists has advanced deltaic stability and sustainability knowledge (Figure 1). Passalacqua et al. (2021) emphasize that delta sustainability requires understanding the spatial scale of sediment transport and hydrodynamic processes, which are best assessed through interdisciplinary methods. Overall, these interdisciplinary collaborations—resolving the old water paradox and advancing delta sustainability—highlight how integrating expertise improves hydrologic understanding, modeling accuracy, and restoration strategies.
Effective management requires not only modeling hydrologic connectivity but also implementing restoration strategies, such as floodplain reconnection, wetland rehabilitation, and riparian corridor restoration (Stoffers et al., 2022), to enhance water and nutrient flow across landscapes (Figure 1). As demonstrated, current approaches often fail to consider how multiple ecological, hydrological, and geomorphological variables interact within an ecosystem. This is an especially problematic oversight when accounting for thresholds, feedback loops, and interactions vital to ecosystem health. Consequently, management efforts often focus on individual variables (e.g., streamflow regulation, sediment transport, or nutrient cycling) in isolation rather than adopting a holistic framework that accounts for system-wide interactions (Zhang et al., 2021). The underutilization of predictive capabilities hampers effective nutrient management, habitat restoration, and flood mitigation. For example, although engineered structures like logjams and beaver dam analogs are increasingly implemented to enhance nitrate removal in headwater streams (e.g., Krause et al., 2024; Wade et al., 2020; Dewey et al., 2022), no reliable method exists to predict the optimal number or placement of these obstructions for achieving desired outcomes (Covino, 2017).
Moreover, current management approaches often need to pay more attention to the complexity of hydrologic connectivity in areas like habitat restoration and flood control (Poeppl et al., 2017, 2020). Restoration projects that reestablish natural water flow—including levee setbacks, wetland rehydration, and beaver dam analogs—are typically based on incomplete models that need to account for hydrological impacts on species migration or vegetation growth. Similarly, flood mitigation strategies often neglect the significance of river corridors and floodplain connectivity, which are critical for water storage and nutrient filtering during high-water events (Wohl et al., 2018; Harvey et al., 2019).
We evaluated the strengths and limitations of current measurement and modeling techniques, emphasizing the need to integrate hydrologic connectivity restoration efforts into management strategies. We highlighted the importance of predictive modeling, interdisciplinary collaboration, and system-wide approaches to enhance hydrologic resilience and inform adaptive water management. We noted that an integrated and interdisciplinary perspective on hydrologic connectivity is key to effective management, drawing from hydrology, biogeochemistry, geomorphology, and ecology to address system-wide complexities. The discussion in the following subsections underscores the need for future research to develop integrated frameworks that combine hydrological, ecological, and biogeochemical processes to tackle pressing environmental challenges like climate change.
Despite advancements in measuring and modeling hydrologic connectivity, several challenges have remained. For instance, traditional field-based methods (e.g., dye tracing) provide high-resolution data but lack broad spatial coverage (see Section 2.1), while remote sensing offers wide spatial coverage but limited temporal resolution (see Section 2.2). Models are limited by high data requirements (e.g., process-based models), computational complexity (e.g., integrated models), and oversimplified representations (e.g., percolation theory-based models), all of which reduce accuracy. Additionally, they often struggle with inadequate subsurface characterization. Addressing these challenges requires improved spatial and temporal coverage for a more comprehensive understanding of hydrologic connectivity. Researchers increasingly rely on remote sensing and predictive modeling to bridge this gap and complement traditional measurement approaches.
Researchers have increasingly integrated automated sensors and drones into field-based methods to enhance spatial coverage, reduce labor costs, and provide consistent real-time data collection (Hubbard et al., 2018; Chen et al., 2021). Advancements in visual surveys and high-temporal-resolution camera-based approaches offer promising solutions for reconstructing stream network dynamics (Noto et al., 2024; Manfreda et al., 2024). Efforts should focus on expanding visual surveys, developing machine learning (ML) tools for image analysis, and integrating high-resolution data into models to improve hydrological predictions. To improve subsurface characterization, Wireless Underground Sensor Networks (WUSN) capable of providing subsurface properties should be leveraged (e.g., Barnhart et al., 2010; Ajo-Franklin et al., 2018).
Although automated sensors, drones, visual surveys, high-temporal-resolution cameras, and WUSN offer deeper insights into localized hydrological processes, their full potential can only be realized through scaling and broader application. Remote sensing advancements improve temporal resolution, such as increased satellite monitoring frequency and combining multiple data types (optical, thermal, radar). Incorporating subsurface sensing technologies will further enrich our understanding of surface and subsurface dynamics.
Artificial Intelligence (AI) and ML are already being used to enhance hydrodynamic models by optimizing calibration and reducing dependence on large datasets (Shen et al., 2023). To achieve global applicability, these models require ongoing refinement to address scalability. Incorporating biogeochemical fluxes into percolation theory would make these models more ecologically relevant; meanwhile, integrating real-time monitoring into graph-theoretic models would capture adaptive changes in connectivity. Although graph-theoretic models often use a fixed structure to represent connections, they can be adapted dynamically by integrating real-time monitoring data (Durighetto et al., 2022; Bertassello et al., 2022). For instance, real-time measurements of flow conditions, water levels, or seasonal variations can update edge weights or node attributes, allowing the graph to reflect temporal changes in connectivity, such as flow interruptions or restored connectivity after precipitation events (Himmel et al., 2017; Casteigts et al., 2021). Integrating this real-time monitoring would enhance the models' ability to capture adaptive changes in connectivity (Casteigts et al., 2021). Similarly, ongoing efforts to simplify entropy-based metrics and embed them into flexible frameworks are progressing, yet further refinement is urgently needed to make them more accessible across various ecosystems (Bennett et al., 2019). Similarly, adaptive river connectivity metrics that factor in real-time environmental changes will enhance assessment precision, provided they are consistently implemented across diverse settings (Godsey and Kirchner, 2014).
Significant strides have been made in developing globally applicable ecohydrological models that account for regional ecological dynamics (e.g., Xu et al., 2022; Shuai et al., 2022), yet further efforts are needed to ensure their consistency and broader applicability. Developing integrated frameworks that combine remote sensing, field methods, and modeling will improve assessments and predictive capabilities and enhance adaptive management of hydrologic connectivity by addressing scale, complexity, and metric standardization gaps. However, achieving these advancements requires not only technical innovations but also interdisciplinary collaboration. Hydrologists, ecologists, and data scientists must work together to integrate diverse methodologies and ensure that emerging tools are effectively implemented across various ecosystems.
Despite the clear benefits of interdisciplinary approaches, several challenges remain (e.g., Hubbard et al., 2020). A major issue is the lack of data standardization across fields (Varadharajan et al., 2019; Faybishenko et al., 2022; Simmonds et al., 2022; Arora et al., 2023), as researchers employ diverse data collection and analysis methods, leading to inconsistencies that hinder integration. For example, hydrologists use high-frequency sensor networks to measure water fluxes at 5-min intervals, whereas geochemists rely on seasonal field sampling to analyze water chemistry (Faybishenko et al., 2022). Similarly, in overland flow connectivity studies, hydrologists focus on processes like infiltration and runoff. At the same time, geomorphologists examine structural factors such as slope and gradient—both essential for understanding hydrologic connectivity (Reaney et al., 2014). Maximizing the impact of interdisciplinary collaboration to advance hydrologic connectivity research requires standardized measurement strategies, particularly in data frequency and spatial distribution, to improve research integration and applicability.
In addition, data availability and accessibility remain challenges, as many datasets are fragmented, discipline-specific, or lack open access. Expanding data-sharing platforms and cross-institutional collaborations can help bridge these gaps. Initiatives like the Department of Energy's Environmental System Science Data Infrastructure for a Virtual Ecosystem (ESS-DIVE), United States Geological Survey (USGS) data repositories, and journal-driven open-access mandates aim to improve data accessibility and support interdisciplinary research (Clark et al., 1993; Varadharajan et al., 2019; Simmonds et al., 2022; Lightsom et al., 2022).
Communication barriers further complicate interdisciplinary research, including hydrologic connectivity studies. Scientists from different fields often use distinct terminologies (e.g., runoff in hydrology vs. overland flow in ecology for the same process) and different measurement frameworks (e.g., infiltration measured using infiltrometers in hydrology vs. soil water recharge assessed in ecology through plant water potential), making collaboration difficult. Addressing these challenges requires fostering a culture of interdisciplinary thinking through education and training. Several studies have advocated for educational frameworks that equip scientists with the skills to work across disciplines, enabling them to understand better complex Earth system interactions (e.g., Arora et al., 2022; Dwivedi et al., 2022; Arora et al., 2024).
Hydrologic connectivity research must develop quantifiable metrics and standardized frameworks through interdisciplinary research and collaboration to enable comparisons across space and time. These tools will enable managers to assess connectivity across diverse regions and time periods, plan accordingly, and simulate future scenarios under changing climate conditions. However, more empirical research is needed to quantify the relationships between hydrologic connectivity and ecological diversity, including habitat heterogeneity and biodiversity (Liu D. et al., 2020; Stoffers et al., 2022).
As discussed in this review, Hydrologic connectivity, encompassing the interplay of hydrological, biogeochemical, ecological, and geomorphological processes, plays a fundamental role in shaping catchment dynamics and resilience. However, both natural processes—such as drought, flooding, and sediment deposition—and human interventions—such as dam removal, fish passage installations, levee setbacks, floodplain reconnection, beaver reintroduction, and wetland rehabilitation—can alter these networks, potentially impacting catchment functionality. Effectively managing these changes requires an integrated approach that combines scientific research with practical applications.
Many field-based studies—such as wetland monitoring (e.g., Ury et al., 2023), river corridor assessments (e.g., Dwivedi et al., 2018a), and beaver dam analog (e.g., Dewey et al., 2022) studies—do not always explicitly assess hydrologic connectivity but still provide valuable insights into its dynamics. These studies can supplement hydrologic connectivity measurements and be used to evaluate restoration interventions by tracking changes in water flow, sediment transport, groundwater levels, and floodplain inundation.
Enhancing or restoring hydrologic connectivity is essential for sustaining water flow regulation, ecosystem resilience, and water quality. Restoring connectivity—reestablishing natural flows within a catchment by removing physical barriers, enhancing natural storage, and improving hydrologic linkages—is a key strategy for effective catchment management and climate adaptation (Jacobson et al., 2022). Key techniques for restoring hydrologic connectivity include longitudinal connectivity restoration, dam removal, fish passage installations, culvert replacement, and sediment transport restoration to improve water, sediment, and organism movement along rivers and streams (Heckmann et al., 2018; Rogosch et al., 2024). Lateral connectivity restoration focuses on reconnecting floodplains through levee setbacks, wetland restoration, and riparian buffer establishment to enhance flood storage and infiltration (Covino, 2017; Ameli and Creed, 2017). Vertical connectivity improvements aim to restore surface-subsurface interactions through soil conservation, permeable urban surfaces, and groundwater flow path restoration to support infiltration and water retention. Ecological connectivity enhances habitat continuity by reintroducing beavers, creating side channels, and managing invasive species to restore natural hydrologic dynamics (Zimmer and McGlynn, 2018; Walker and Hassall, 2021).
While policy measures—such as wetland protection laws, river corridor zoning—and financial incentives for floodplain restoration, and ecological interventions—such as dam removal, riparian buffer restoration, and reintroducing beavers—can help restore hydrologic connectivity, successful implementation depends on a robust understanding of the complex interactions among water, sediment, and ecosystems across landscapes (Magilligan et al., 2016; Kendall, 2023; Brown et al., 2024). Because these interactions are complex and abstract, analogies—such as social networks, ecological corridors, and fluid flow—help visualize connectivity patterns (Masselink et al., 2017; Rinaldo et al., 2018; Gooseff et al., 2017). Similarly, hydrologic connectivity can be likened to an electric circuit, where water flow resembles electrical current, obstacles such as vegetation and rocky outcrops act as resistors, and wetlands function as capacitors, temporarily storing and regulating water (Al-Sayouri et al., 2018; Hasanah et al., 2022). These interdisciplinary frameworks provide valuable tools for research and management, though further refinement is needed for effective real-world application.
This review synthesizes current approaches to measuring, modeling, and managing hydrologic connectivity, focusing on the importance of interdisciplinary collaboration (e.g., between hydrologists and ecologists) and the potential of emerging technologies such as drones and imagery. Traditional measurement and modeling approaches for hydrologic connectivity struggle to capture complex catchment interactions, constrained by spatial, temporal, and computational limitations, as well as the inherent difficulty of integrating subsurface processes with surface hydrology. Other challenges are posed by fragmented datasets, limited open-access data, and communication barriers across disciplines. Without broader implementation and comparability across different regions and scales, the true potential of hydrologic connectivity in water resource management cannot be realized, yet the absence of standardized connectivity metrics and methodologies hampers progress. All of these issues require urgent attention.
A comprehensive approach to hydrologic connectivity should integrate emerging technologies—including camera imagery, drones, AI, and WUSN—to enhance data collection and improve spatial and temporal coverage. Expanding standardization efforts, including open-access data platforms (e.g., ESS-DIVE, USGS repositories) and unified measurement strategies, will facilitate interdisciplinary collaboration and improve data integration. A unified framework is required to facilitate interdisciplinary collaboration and enhance science-based decision-making in water resource management. Conceptual simplifications, such as the electric circuit analogy, can provide such a framework by illustrating hydrologic connectivity holistically within a catchment and fostering a shared perspective, common language, and integrated approach across disciplines. Future research should prioritize high-resolution and long-term monitoring as well as adaptive management to restore hydrologic connectivity in fragmented landscapes. Management strategies must integrate predictive modeling to account for system-wide connectivity, anticipate climate-driven shifts, and enhance ecosystem resilience. Ultimately, this review inspires the catchment science community to transform hydrologic connectivity research into practical management tools that guide policy decisions and promote adaptive, resilient water resource strategies.
DD: Conceptualization, Methodology, Writing – original draft, Writing – review & editing. RP: Conceptualization, Writing – review & editing. EW: Conceptualization, Writing – review & editing.
The author(s) declare that financial support was received for the research and/or publication of this article. DDs work was supported by the Watershed Function Scientific Focus Area at Lawrence Berkeley National Laboratory, funded by the U.S. Department of Energy, Office of Science, Office of Biological and Environmental Research, under Award No. DE-AC02-05CH11231.
Graphics support provided by Susana Roque-Malo from Convey Scientific Graphics and Ella Maru Studio.
The authors declare that the research was conducted in the absence of any commercial or financial relationships that could be construed as a potential conflict of interest.
The author(s) declared that they were an editorial board member of Frontiers, at the time of submission. This had no impact on the peer review process and the final decision.
All claims expressed in this article are solely those of the authors and do not necessarily represent those of their affiliated organizations, or those of the publisher, the editors and the reviewers. Any product that may be evaluated in this article, or claim that may be made by its manufacturer, is not guaranteed or endorsed by the publisher.
Ackerer, J., Kuppel, S., Braud, I., Pasquet, S., Fovet, O., Probst, A., et al. (2023). Exploring the critical zone heterogeneity and the hydrological diversity using an integrated ecohydrological model in three contrasted long-term observatories. Water Resour. Res. 59:e2023WR035672. doi: 10.1029/2023WR035672
Aho, K. B., Flotemersch, J. E., Leibowitz, S. G., LaCroix, M. A., and Weber, M. H. (2020). Applying the index of watershed integrity to the matanuska-susitna basin. Arct. Antarct. Alp. Res. 52, 435–449. doi: 10.1080/15230430.2020.1800219
Ajo-Franklin, J., Dou, S., Lindsey, N., Monga, I., Tracy, C., Robertson, M., et al. (2018). Using dark fiber and distributed acoustic sensing for near-surface characterization and broadband seismic event detection. Sci. Rep. 9:1328. doi: 10.31223/OSF.IO/KXQB2
Al-Sayouri, S. A., Koutra, D., Papalexakis, E. E., and Lam, S. S. (2018). Recs: robust graph embedding using connection subgraphs. arXiv preprint arXiv:1805.01509.
Ameli, A. A., and Creed, I. F. (2017). Quantifying hydrologic connectivity of wetlands to surface water systems. Hydrol. Earth Syst. Sci. 21, 1791–1808. doi: 10.5194/hess-21-1791-2017
Appling, A. P., Bernhardt, E. S., and Stanford, J. A. (2014). Floodplain biogeochemical mosaics: a multidimensional view of alluvial soils. J. Geophys. Res. 119, 1538–1553. doi: 10.1002/2013JG002543
Arora, B., Currin, A., Dwivedi, D., Fru, M. I., Kumar, N., McLeod, C. L., et al. (2022). Volcanology, geochemistry, and petrology perspectives on integrated, coordinated, open, networked (ICON) science. Earth Space Sci. 9:e2021EA002120. doi: 10.1029/2021EA002120
Arora, B., Kuppel, S., Wellen, C., Oswald, C., Groh, J., Payandi-Rolland, D., et al. (2023). Building cross-site and cross-network collaborations in critical zone science. J. Hydrol. 618:129248. doi: 10.1016/j.jhydrol.2023.129248
Arora, B., Seybold, E., and Jin, L. (2024). Women in critical zone science. Front. Water 6:1404317. doi: 10.3389/frwa.2024.1404317
Arora, B., Spycher, N. F., Steefel, C. I., Molins, S., Bill, M., Conrad, M. E., et al. (2016). Influence of hydrological, biogeochemical and temperature transients on subsurface carbon fluxes in a flood plain environment. Biogeochemistry 127, 367–396. doi: 10.1007/s10533-016-0186-8
Arora, B., Wainwright, H. M., Dwivedi, D., Vaughn, L. J., Curtis, J. B., Torn, M. S., et al. (2019). Evaluating temporal controls on greenhouse gas (ghg) fluxes in an arctic tundra environment: an entropy-based approach. Sci. Total Environ. 649, 284–299. doi: 10.1016/j.scitotenv.2018.08.251
Baram, S., Ronen, Z., Kurtzman, D., Külls, C., and Dahan, O. (2013). Desiccation-crack-induced salinization in deep clay sediment. Hydrol. Earth Syst. Sci. 17, 1533–1545. doi: 10.5194/hess-17-1533-2013
Barnhart, K., Urteaga, I., Han, Q., Jayasumana, A., and Illangasekare, T. (2010). On integrating groundwater transport models with wireless sensor networks. Groundwater 48, 771–780. doi: 10.1111/j.1745-6584.2010.00684.x
Bennett, A., Nijssen, B., Ou, G., Clark, M., and Nearing, G. (2019). Quantifying process connectivity with transfer entropy in hydrologic models. Water Resour. Res. 55, 4613–4629. doi: 10.1029/2018WR024555
Bertassello, L. E., Durighetto, N., and Botter, G. (2022). Eco-hydrological modelling of channel network dynamics part 2: application to metapopulation dynamics. R. Soc. Open Sci. 9:220945. doi: 10.1098/rsos.220945
Bertassello, L. E., Rao, P. S. C., Jawitz, J. W., Aubeneau, A. F., and Botter, G. (2020). Wetlandscape hydrologic dynamics driven by shallow groundwater and landscape topography. Hydrol. Process. 34, 1460–1474. doi: 10.1002/hyp.13661
Beven, K. J. (2012). Rainfall-Runoff Modelling: the Primer. New York: John Wiley &Sons. doi: 10.1002/9781119951001
Borselli, L., Cassi, P., and Torri, D. (2008). Prolegomena to sediment and flow connectivity in the landscape: a gis and field numerical assessment. Catena 75, 268–277. doi: 10.1016/j.catena.2008.07.006
Bracken, L. J., and Croke, J. (2007). The concept of hydrological connectivity and its contribution to understanding runoff-dominated geomorphic systems. Hydrol. Proc. 21, 1749–1763. doi: 10.1002/hyp.6313
Bracken, L. J., Turnbull, L., Wainwright, J., and Bogaart, P. (2015). Sediment connectivity: a framework for understanding sediment transfer at multiple scales. Earth Surf. Proc. Landf. 40, 177–188. doi: 10.1002/esp.3635
Bracken, L. J., Wainwright, J., Ali, G., Tetzlaff, D., Smith, M., Reaney, S., et al. (2013). Concepts of hydrological connectivity: research approaches, pathways and future agendas. Earth-Sci. Rev. 119, 17–34. doi: 10.1016/j.earscirev.2013.02.001
Brown, R. L., Charles, D., Horwitz, R. J., Pizzuto, J. E., Skalak, K., Velinsky, D. J., et al. (2024). Size-dependent effects of dams on river ecosystems and implications for dam removal outcomes. Ecol. Applic. 34:e3016. doi: 10.1002/eap.3016
Buddendorf, W., Malcolm, I., Geris, J., Fabris, L., Millidine, K., Wilkinson, M., et al. (2017). Spatio-temporal effects of river regulation on habitat quality for atlantic salmon fry. Ecol. Indic. 83, 292–302. doi: 10.1016/j.ecolind.2017.08.006
Camporese, M., Paniconi, C., Putti, M., and McDonnell, J. J. (2019). Fill and spill hillslope runoff representation with a richards equation-based model. Water Resour. Res. 55, 8445–8462. doi: 10.1029/2019WR025726
Cartwright, I., and Morgenstern, U. (2012). Constraining groundwater recharge and the rate of geochemical processes using tritium and major ion geochemistry: ovens catchment, southeast australia. J. Hydrol. 475, 137–149. doi: 10.1016/j.jhydrol.2012.09.037
Casteigts, A., Peters, J. G., and Schoeters, J. (2021). Temporal cliques admit sparse spanners. J. Comput. Syst. Sci. 121, 1–17. doi: 10.1016/j.jcss.2021.04.004
Chen, X., Lee, R. M., Dwivedi, D., Son, K., Fang, Y., Zhang, X., et al. (2021). Integrating field observations and process-based modeling to predict watershed water quality under environmental perturbations. J. Hydrol. 602:125762. doi: 10.1016/j.jhydrol.2020.125762
Clark, R. N., Swayze, G. A., Gallagher, A. J., King, T. V., and Calvin, W. M. (1993). The us geological survey, digital spectral library: version 1 (0.2 to 3.0 um). Technical report, Geological Survey (US). doi: 10.3133/ofr93592
Coon, E. T., and Shuai, P. (2022). Watershed workflow: a toolset for parameterizing data-intensive, integrated hydrologic models. Environ. Model. Softw. 157:105502. doi: 10.1016/j.envsoft.2022.105502
Covino, T. (2017). Hydrologic connectivity as a framework for understanding biogeochemical flux through watersheds and along fluvial networks. Geomorphology 277, 133–144. doi: 10.1016/j.geomorph.2016.09.030
Dahan, O., Talby, R., Yechieli, Y., Adar, E., Lazarovitch, N., and Enzel, Y. (2009). In situ monitoring of water percolation and solute transport using a vadose zone monitoring system. Vadose Zone J. 8, 916–925. doi: 10.2136/vzj2008.0134
Dewey, C., Fox, P. M., Bouskill, N. J., Dwivedi, D., Nico, P., and Fendorf, S. (2022). Beaver dams overshadow climate extremes in controlling riparian hydrology and water quality. Nat. Commun. 13:6509. doi: 10.1038/s41467-022-34022-0
Drummond, J., Aubeneau, A., and Packman, A. (2014). Stochastic modeling of fine particulate organic carbon dynamics in rivers. Water Resour. Res. 50, 4341–4356. doi: 10.1002/2013WR014665
Durighetto, N., Mariotto, V., Zanetti, F., McGuire, K. J., Mendicino, G., Senatore, A., et al. (2022). Probabilistic description of streamflow and active length regimes in rivers. Water Resour. Res. 58:e2021WR031344. doi: 10.1029/2021WR031344
Durighetto, N., Noto, S., Tauro, F., Grimaldi, S., and Botter, G. (2023). Integrating spatially-and temporally-heterogeneous data on river network dynamics using graph theory. Iscience 26:107417. doi: 10.1016/j.isci.2023.107417
Dwivedi, D., Arora, B., Steefel, C. I., Dafflon, B., and Versteeg, R. (2018a). Hot spots and hot moments of nitrogen in a riparian corridor. Water Resour. Res. 54, 205–222. doi: 10.1002/2017WR022346
Dwivedi, D., and Mohanty, B. P. (2016). Hot spots and persistence of nitrate in aquifers across scales. Entropy 18:25. doi: 10.3390/e18010025
Dwivedi, D., Santos, A., Barnard, M. A., Crimmins, T. M., Malhotra, A., Rod, K. A., et al. (2022). Biogeosciences perspectives on integrated, coordinated, open, networked (ICON) science. Earth Space Sci. 9:e2021EA002119. doi: 10.1029/2021EA002119
Dwivedi, D., Steefel, C. I., Arora, B., Newcomer, M., Moulton, J. D., Dafflon, B., et al. (2018b). Geochemical exports to river from the intrameander hyporheic zone under transient hydrologic conditions: east river mountainous watershed, colorado. Water Resour. Res. 54, 8456–8477. doi: 10.1029/2018WR023377
Fang, S., Chen, M., Li, J., Zhang, L., Zhang, Y., Yang, C., et al. (2024). Evaluation and optimization of urban hydrological connectivity based on graph theory: a case study in chengdu, China. Ecol. Inform. 82:102749. doi: 10.1016/j.ecoinf.2024.102749
Faybishenko, B., Versteeg, R., Pastorello, G., Dwivedi, D., Varadharajan, C., and Agarwal, D. (2022). Challenging problems of quality assurance and quality control (QA/QC) of meteorological time series data. Stoch. Environ. Res. Risk Assess. 36, 1049–1062. doi: 10.1007/s00477-021-02106-w
Fortuna, M. A., Gómez-Rodríguez, C., and Bascompte, J. (2006). Spatial network structure and amphibian persistence in stochastic environments. Proc. R. Soc. B Biol. Sci. 273, 1429–1434. doi: 10.1098/rspb.2005.3448
Fox, P. M., Carrero, S., Anderson, C., Dewey, C., Keiluweit, M., Conrad, M., et al. (2022). Sulfur biogeochemical cycling and redox dynamics in a shale-dominated mountainous watershed. J. Geophys. Res. 127:e2021JG006769. doi: 10.1029/2021JG006769
Fryirs, K. A., Brierley, G. J., Preston, N. J., and Kasai, M. (2007). Buffers, barriers and blankets: The (dis) connectivity of catchment-scale sediment cascades. Catena 70, 49–67. doi: 10.1016/j.catena.2006.07.007
Garbin, S., Celegon, E. A., Fanton, P., and Botter, G. (2019). Hydrological controls on river network connectivity. R. Soc. Open Sci. 6:181428. doi: 10.1098/rsos.181428
Godsey, S., and Kirchner, J. W. (2014). Dynamic, discontinuous stream networks: hydrologically driven variations in active drainage density, flowing channels and stream order. Hydrol. Process. 28, 5791–5803. doi: 10.1002/hyp.10310
Godsey, S. E., Hartmann, J., and Kirchner, J. W. (2019). Catchment chemostasis revisited: water quality responds differently to variations in weather and climate. Hydrol. Process. 33, 3056–3069. doi: 10.1002/hyp.13554
Good, S. P., Noone, D., and Bowen, G. (2015). Hydrologic connectivity constrains partitioning of global terrestrial water fluxes. Science 349, 175–177. doi: 10.1126/science.aaa5931
Gooseff, M. N., Wlostowski, A., McKnight, D. M., and Jaros, C. (2017). Hydrologic connectivity and implications for ecosystem processes-lessons from naked watersheds. Geomorphology 277, 63–71. doi: 10.1016/j.geomorph.2016.04.024
Haarstad, K., Bavor, H., and Mæhlum, T. (2012). Organic and metallic pollutants in water treatment and natural wetlands: a review. Water Sci. Technol. 65, 76–99. doi: 10.2166/wst.2011.831
Harvey, J., Gomez-Velez, J., Schmadel, N., Scott, D., Boyer, E., Alexander, R., et al. (2019). How hydrologic connectivity regulates water quality in river corridors. JAWRA J. Am. Water Resour. Assoc. 55, 369–381. doi: 10.1111/1752-1688.12691
Harvey, J., and Gooseff, M. (2015). River corridor science: hydrologic exchange and ecological consequences from bedforms to basins. Water Resour. Res. 51, 6893–6922. doi: 10.1002/2015WR017617
Harvey, J. W., Drummond, J. D., Martin, R. L., McPhillips, L. E., Packman, A. I., Jerolmack, D., et al. (2012). Hydrogeomorphology of the hyporheic zone: stream solute and fine particle interactions with a dynamic streambed. J. Geophys. Res. 117:2043. doi: 10.1029/2012JG002043
Hasanah, A., Sunarya, A., and Viridi, S. (2022). “Charge and discharge of capasitors: revisiting the analogy with water in connected containers, in Journal of Physics: Conference Series (IOP Publishing), 012073. doi: 10.1088/1742-6596/2243/1/012073
Heckmann, T., Cavalli, M., Cerdan, O., Foerster, S., Javaux, M., Lode, E., et al. (2018). Indices of sediment connectivity: opportunities, challenges and limitations. Earth-Sci. Rev. 187, 77–108. doi: 10.1016/j.earscirev.2018.08.004
Helton, A. M., Poole, G. C., Payn, R. A., Izurieta, C., and Stanford, J. A. (2014). Relative influences of the river channel, floodplain surface, and alluvial aquifer on simulated hydrologic residence time in a montane river floodplain. Geomorphology 205, 17–26. doi: 10.1016/j.geomorph.2012.01.004
Herzog, A., Hellwig, J., and Stahl, K. (2024). An investigation of anthropogenic influences on hydrologic connectivity using model stress tests. Hydrol. Earth Syst. Sci. 28, 4065–4083. doi: 10.5194/hess-28-4065-2024
Himmel, A.-S., Molter, H., Niedermeier, R., and Sorge, M. (2017). Adapting the bron-kerbosch algorithm for enumerating maximal cliques in temporal graphs. Soc. Netw. Anal. Mining 7, 1–16. doi: 10.1007/s13278-017-0455-0
Huang, S., Wang, P., Hua, Z., Dong, Y., and Shi, J. (2024). Structural characteristics and spatiotemporal changes of a reticular river network based on complex network theory. J. Hydrol. 638:131577. doi: 10.1016/j.jhydrol.2024.131577
Hubbard, S. S., Varadharajan, C., Wu, Y., Wainwright, H., and Dwivedi, D. (2020). Emerging technologies and radical collaboration to advance predictive understanding of watershed hydrobiogeochemistry. Hydrol. Process. 34, 3175–3182. doi: 10.1002/hyp.13807
Hubbard, S. S., Williams, K. H., Agarwal, D., Banfield, J., Beller, H., Bouskill, N., et al. (2018). The east river, colorado, watershed: a mountainous community testbed for improving predictive understanding of multiscale hydrological-biogeochemical dynamics. Vadose Zone J. 17, 1–25. doi: 10.2136/vzj2018.03.0061
iConn Network (2024). Useful papers. Available online at: https://iconn.network/network-resources/useful-papers/ (accessed December 20, 2024).
Jacobson, R. B., Bouska, K. L., Bulliner, E. A., Lindner, G. A., and Paukert, C. P. (2022). Geomorphic controls on floodplain connectivity, ecosystem services, and sensitivity to climate change: an example from the lower missouri river. Water Resour. Res. 58:e2021WR031204. doi: 10.1029/2021WR031204
Julien, P., and Simons, D. (1985). Sediment transport capacity of overland flow. Trans. ASAE 28, 755–0762. doi: 10.13031/2013.32333
Keesstra, S., Nunes, J. P., Saco, P., Parsons, T., Poeppl, R., Masselink, R., et al. (2018). The way forward: can connectivity be useful to design better measuring and modelling schemes for water and sediment dynamics? Sci. Total Environ. 644, 1557–1572. doi: 10.1016/j.scitotenv.2018.06.342
Kendall, M. (2023). From Wastelands to Wetlands: The Story of Coastal Wetlands in the United States. PhD thesis, Duke University.
Kirchner, J. W. (2003). A double paradox in catchment hydrology and geochemistry. Hydrol. Process. 17, 871–874. doi: 10.1002/hyp.5108
Krause, S., Lewandowski, J., Grimm, N. B., Hannah, D. M., Pinay, G., McDonald, K., et al. (2024). Ecohydrological interfaces as hotspots of ecosystem processes. Ecohydrol. Interf. 56, 1–28. doi: 10.1002/9781119489702.ch1
Lane, C. R., Leibowitz, S. G., Autrey, B. C., LeDuc, S. D., and Alexander, L. C. (2018). Hydrological, physical, and chemical functions and connectivity of non-floodplain wetlands to downstream waters: a review. JAWRA 54, 346–371. doi: 10.1111/1752-1688.12633
Lee, E., Epstein, J. M., and Cohen, M. J. (2023). Patterns of wetland hydrologic connectivity across coastal-plain wetlandscapes. Water Resour. Res. 59:e2023WR034553. doi: 10.1029/2023WR034553
Lee, S., Lee, B., Lee, J., Song, J., and McCarty, G. W. (2023). Detecting causal relationship of non-floodplain wetland hydrologic connectivity using convergent cross mapping. Sci. Rep. 13:17220. doi: 10.1038/s41598-023-44071-0
Lehmann, P., Hinz, C., McGrath, G., Tromp-van Meerveld, H., and McDonnell, J. J. (2007). Rainfall threshold for hillslope outflow: an emergent property of flow pathway connectivity. Hydrol. Earth Syst. Sci. 11, 1047–1063. doi: 10.5194/hess-11-1047-2007
Leibowitz, S. G., Wigington Jr, P. J., Schofield, K. A., Alexander, L. C., Vanderhoof, M. K., and Golden, H. E. (2018). Connectivity of streams and wetlands to downstream waters: an integrated systems framework. JAWRA 54, 298–322. doi: 10.1111/1752-1688.12631
Lewandowski, J., Meinikmann, K., Nützmann, G., and Rosenberry, D. O. (2015). Groundwater-the disregarded component in lake water and nutrient budgets. part 2: effects of groundwater on nutrients. Hydrol. Proc. 29, 2922–2955. doi: 10.1002/hyp.10384
Lexartza-Artza, I., and Wainwright, J. (2009). Hydrological connectivity: Linking concepts with practical implications. Catena 79, 146–152. doi: 10.1016/j.catena.2009.07.001
Lexartza-Artza, I., and Wainwright, J. (2011). Making connections: changing sediment sources and sinks in an upland catchment. Earth Surf. Proc. Landforms 36, 1090–1104. doi: 10.1002/esp.2134
Li, L., Knapp, J. L., Lintern, A., Ng, G.-H. C., Perdrial, J., Sullivan, P. L., et al. (2024). River water quality shaped by land-river connectivity in a changing climate. Nat. Clim. Chang. 14, 225–237. doi: 10.1038/s41558-023-01923-x
Lightsom, F. L., Hutchison, V. B., Bishop, B., Debrewer, L. M., Govoni, D. L., Latysh, N., et al. (2022). Opportunities to improve alignment with the fair principles for us geological survey data. Technical report, US Geological Survey. doi: 10.3133/ofr20221043
Liu, D., Wang, X., Aminjafari, S., Yang, W., Cui, B., Yan, S., et al. (2020). Using insar to identify hydrological connectivity and barriers in a highly fragmented wetland. Hydrol. Process. 34, 4417–4430. doi: 10.1002/hyp.13899
Liu, J., Engel, B. A., Wang, Y., Wu, Y., Zhang, Z., and Zhang, M. (2019). Runoff response to soil moisture and micro-topographic structure on the plot scale. Sci. Rep. 9:2532. doi: 10.1038/s41598-019-39409-6
Liu, Y., Wallace, C. D., Zhou, Y., Ershadnia, R., Behzadi, F., Dwivedi, D., et al. (2020). Influence of streambed heterogeneity on hyporheic flow and sorptive solute transport. Water 12:1547. doi: 10.3390/w12061547
Magilligan, F. J., Graber, B. E., Nislow, K. H., Chipman, J. W., Sneddon, C. S., and Fox, C. A. (2016). River restoration by dam removal: enhancing connectivity at watershed scales. Elementa 4:000108. doi: 10.12952/journal.elementa.000108
Manfreda, S., Miglino, D., Saddi, K. C., Jomaa, S., Eltner, A., Perks, M., et al. (2024). Advancing river monitoring using image-based techniques: challenges and opportunities. Hydrol. Sci. J. 69, 657–677. doi: 10.1080/02626667.2024.2333846
Masselink, R. J., Heckmann, T., Temme, A. J., Anders, N. S., Gooren, H. P., and Keesstra, S. D. (2017). A network theory approach for a better understanding of overland flow connectivity. Hydrol. Process. 31, 207–220. doi: 10.1002/hyp.10993
Maxwell, C. M., Fernald, A. G., Cadol, D., Faist, A. M., and King, J. P. (2021). Managing flood flow connectivity to landscapes to build buffering capacity to disturbances: an ecohydrologic modeling framework for drylands. J. Environ. Manage. 278:111486. doi: 10.1016/j.jenvman.2020.111486
Mikkelson, K., Maxwell, R., Ferguson, I., Stednick, J., McCray, J., and Sharp, J. (2013). Mountain pine beetle infestation impacts: modeling water and energy budgets at the hill-slope scale. Ecohydrology 6, 64–72. doi: 10.1002/eco.278
Noto, S., Durighetto, N., Tauro, F., Grimaldi, S., and Botter, G. (2024). Characterizing space-time channel network dynamics in a mediterranean intermittent catchment of central Italy combining visual surveys and cameras. Water Resour. Res. 60:e2023WR034682. doi: 10.1029/2023WR034682
Olliver, E. A., Edmonds, D., and Shaw, J. B. (2020). Influence of floods, tides, and vegetation on sediment retention in wax Lake Delta, Louisiana, USA. J. Geophys. Res. 125:e2019JF005316. doi: 10.1029/2019JF005316
Passalacqua, P. (2017). The delta connectome: a network-based framework for studying connectivity in river deltas. Geomorphology 277, 50–62. doi: 10.1016/j.geomorph.2016.04.001
Passalacqua, P., Giosan, L., Goodbred, S., and Overeem, I. (2021). Stable≠ sustainable: delta dynamics versus the human need for stability. Earth's Future 9:e2021EF002121. doi: 10.1029/2021EF002121
Poeppl, R. E., Fryirs, K. A., Tunnicliffe, J., and Brierley, G. J. (2020). Managing sediment (dis) connectivity in fluvial systems. Sci. Total Environ. 736:139627. doi: 10.1016/j.scitotenv.2020.139627
Poeppl, R. E., Keesstra, S. D., and Maroulis, J. (2017). A conceptual connectivity framework for understanding geomorphic change in human-impacted fluvial systems. Geomorphology 277, 237–250. doi: 10.1016/j.geomorph.2016.07.033
Pöppl, R. E., Parsons, A. J., and Keesstra, S. D. (2024). “Connectivity in geomorphology, in Connectivity in Geomorphology, eds. R. E. Pöppl, A. J. Parsons, and S. D. Keesstra (Cambridge: Cambridge University Press).
Pringle, C. M. (2001). Hydrologic connectivity and the management of biological reserves: a global perspective. Ecol. Applic. 11, 981–998. doi: 10.1890/1051-0761(2001)0110981:HCATMO2.0.CO;2
Prosser, I. P., Dietrich, W. E., and Stevenson, J. (1995). Flow resistance and sediment transport by concentrated overland flow in a grassland valley. Geomorphology 13, 71–86. doi: 10.1016/0169-555X(95)00020-6
Rains, M., Leibowitz, S., Cohen, M., Creed, I., Golden, H., Jawitz, J., et al. (2016). Geographically isolated wetlands are part of the hydrological landscape. Hydrol. Process. 30, 153–160. doi: 10.1002/hyp.10610
Reaney, S., Bracken, L. J., and Kirkby, M. (2014). The importance of surface controls on overland flow connectivity in semi-arid environments: results from a numerical experimental approach. Hydrol. Process. 28, 2116–2128. doi: 10.1002/hyp.9769
Renard, P., and Allard, D. (2013). Connectivity metrics for subsurface flow and transport. Adv. Water Resour. 51, 168–196. doi: 10.1016/j.advwatres.2011.12.001
Rimon, Y., Nativ, R., and Dahan, O. (2011). Physical and chemical evidence for pore-scale dual-domain flow in the vadose zone. Vadose Zone J. 10, 322–331. doi: 10.2136/vzj2009.0113
Rinaldo, A., Gatto, M., and Rodriguez-Iturbe, I. (2018). River networks as ecological corridors: a coherent ecohydrological perspective. Adv. Water Resour. 112, 27–58. doi: 10.1016/j.advwatres.2017.10.005
Rogosch, J. S., Boehm, H. I., Tingley III, R. W., Wright, K. D., Webb, E. B., and Paukert, C. P. (2024). Evaluating effectiveness of restoration to address current stressors to riverine fish. Freshw. Biol. 69, 607–622. doi: 10.1111/fwb.14232
Roy, S. (2023). “Identification of potential anthropogenic barriers to fluvial connectivity in the lower gangetic basin of India”, in Environmental Management and Sustainability in India: Case Studies from West Bengal (Springer), 35–55. doi: 10.1007/978-3-031-31399-8_3
Schumm, S. A. (1965). Quaternary Paleohydrology. The Quaternary of the U.S. Princeton: Princeton University Press, 783–794. doi: 10.1515/9781400876525-050
Şensoy, A., Uysal, G., and Şorman, A. (2018). Developing a decision support framework for real-time flood management using integrated models. J. Flood Risk Manag. 11, S866–S883. doi: 10.1111/jfr3.12280
Shen, C., Appling, A. P., Gentine, P., Bandai, T., Gupta, H., Tartakovsky, A., et al. (2023). Differentiable modelling to unify machine learning and physical models for geosciences. Nat. Rev. Earth Environ. 4, 552–567. doi: 10.1038/s43017-023-00450-9
Shuai, P., Chen, X., Mital, U., Coon, E. T., and Dwivedi, D. (2022). The effects of spatial and temporal resolution of gridded meteorological forcing on watershed hydrological responses. Hydrol. Earth Syst. Sci. 26, 2245–2276. doi: 10.5194/hess-26-2245-2022
Simmonds, M. B., Riley, W. J., Agarwal, D. A., Chen, X., Cholia, S., Crystal-Ornelas, R., et al. (2022). Guidelines for publicly archiving terrestrial model data to enhance usability, intercomparison, and synthesis. Data Sci. J. 21:3. doi: 10.5334/dsj-2022-003
Siqueira, V. A., Paiva, R. C., Fleischmann, A. S., Fan, F. M., Ruhoff, A. L., Pontes, P. R., et al. (2018). Toward continental hydrologic-hydrodynamic modeling in South America. Hydrol. Earth Syst. Sci. 22, 4815–4842. doi: 10.5194/hess-22-4815-2018
Somers, L. D., Gordon, R. P., McKenzie, J. M., Lautz, L. K., Wigmore, O., Glose, A., et al. (2016). Quantifying groundwater-surface water interactions in a proglacial Valley, Cordillera Blanca, Peru. Hydrol. Process. 30, 2915–2929. doi: 10.1002/hyp.10912
Stoffers, T., Buijse, A., Geerling, G., Jans, L., Schoor, M., Poos, J., et al. (2022). Freshwater fish biodiversity restoration in floodplain rivers requires connectivity and habitat heterogeneity at multiple spatial scales. Sci. Total Environ. 838:156509. doi: 10.1016/j.scitotenv.2022.156509
Tapley, B. D., Bettadpur, S., Ries, J. C., Thompson, P. F., and Watkins, M. M. (2004). Grace measurements of mass variability in the earth system. Science 305, 503–505. doi: 10.1126/science.1099192
Taylor, P. D., Fahrig, L., Henein, K., and Merriam, G. (1993). Connectivity is a vital element of landscape structure. Oikos 68, 571–573. doi: 10.2307/3544927
Tejedor, A., Longjas, A., Zaliapin, I., and Foufoula-Georgiou, E. (2015). Delta channel networks: 2. metrics of topologic and dynamic complexity for delta comparison, physical inference, and vulnerability assessment Water Resour. Res. 51, 4019–4045. doi: 10.1002/2014WR016604
Turnbull, L., Hütt, M.-T., Ioannides, A. A., Kininmonth, S., Poeppl, R., Tockner, K., et al. (2018). Connectivity and complex systems: learning from a multi-disciplinary perspective. Appl. Netw. Sci. 3, 1–49. doi: 10.1007/s41109-018-0067-2
Turnbull, L., Wainwright, J., and Brazier, R. E. (2008). A conceptual framework for understanding semi-arid land degradation: ecohydrological interactions across multiple-space and time scales. Ecohydrology 1, 23–34. doi: 10.1002/eco.4
Uno, H., Yokoi, M., Fukushima, K., Kanno, Y., Kishida, O., Mamiya, W., et al. (2022). Spatially variable hydrological and biological processes shape diverse post-flood aquatic communities. Freshw. Biol. 67, 549–563. doi: 10.1111/fwb.13862
Ury, E. A., Arrumugam, P., Herbert, E. R., Badiou, P., Page, B., and Basu, N. B. (2023). Source or sink? Meta-analysis reveals diverging controls of phosphorus retention and release in restored and constructed wetlands. Environ. Res. Lett. 18:083002. doi: 10.1088/1748-9326/ace6bf
Van Meerveld, H., Jones, J. P., Ghimire, C. P., Zwartendijk, B. W., Lahitiana, J., Ravelona, M., et al. (2021). Forest regeneration can positively contribute to local hydrological ecosystem services: implications for forest landscape restoration. J. Appl. Ecol. 58, 755–765. doi: 10.1111/1365-2664.13836
Varadharajan, C., Agarwal, D. A., Brown, W., Burrus, M., Carroll, R. W., Christianson, D. S., et al. (2019). Challenges in building an end-to-end system for acquisition, management, and integration of diverse data from sensor networks in watersheds: lessons from a mountainous community observatory in east river, colorado. IEEE Access 7, 182796–182813. doi: 10.1109/ACCESS.2019.2957793
Wade, J., Lautz, L., Kelleher, C., Vidon, P., Davis, J., Beltran, J., et al. (2020). Beaver dam analogues drive heterogeneous groundwater-surface water interactions. Hydrol. Process. 34, 5340–5353. doi: 10.1002/hyp.13947
Wainwright, J., Turnbull, L., Ibrahim, T. G., Lexartza-Artza, I., Thornton, S. F., and Brazier, R. E. (2011). Linking environmental regimes, space and time: interpretations of structural and functional connectivity. Geomorphology 126, 387–404. doi: 10.1016/j.geomorph.2010.07.027
Walker, J. R., and Hassall, C. (2021). The effects of water chemistry and lock-mediated connectivity on macroinvertebrate diversity and community structure in a canal in northern england. Urban Ecosyst. 24, 491–500. doi: 10.1007/s11252-020-01053-8
Ward, J. (1989). The four-dimensional nature of lotic ecosystems. J. North Am. Benthol. Soc. 8, 2–8. doi: 10.2307/1467397
Wohl, E., Brierley, G., Cadol, D., Coulthard, T. J., Covino, T., Fryirs, K. A., et al. (2019). Connectivity as an emergent property of geomorphic systems. Earth Surf. Proc. Landforms 44, 4–26. doi: 10.1002/esp.4434
Wohl, E., Lininger, K. B., and Scott, D. N. (2018). River beads as a conceptual framework for building carbon storage and resilience to extreme climate events into river management. Biogeochemistry 141, 365–383. doi: 10.1007/s10533-017-0397-7
Wu, L., Zhang, M., Xie, X., Lv, J., Zhou, X., and Shen, N. (2023). Effect of wetland hydrological connectivity on runoff based on a basin comparison. J. Hydrol. 619:129348. doi: 10.1016/j.jhydrol.2023.129348
Xingyuan, Z., Fawen, L., and Yong, Z. (2023). Impact of changes in river network structure on hydrological connectivity of watersheds. Ecol. Indic. 146:109848. doi: 10.1016/j.ecolind.2022.109848
Xu, Z., Molins, S., Özgen-Xian, I., Dwivedi, D., Svyatsky, D., Moulton, J. D., et al. (2022). Understanding the hydrogeochemical response of a mountainous watershed using integrated surface-subsurface flow and reactive transport modeling. Water Resour. Res. 58:e2022WR032075. doi: 10.1029/2022WR032075
Yang, Q., Hu, P., Wang, J., Zeng, Q., Yang, Z., Liu, H., et al. (2021). The stereoscopic spatial connectivity of wetland ecosystems: evaluation method and regulation measures. Hydrol. Process. 35:e14074. doi: 10.1002/hyp.14074
Yang, Q., Zhang, C., Liu, X., Yao, S., and Huang, R. (2023). Assessment of hydrological connectivity characteristics of riparian zones and their correlation with root-soil composites at different bank heights of a first-class river in China. Front. Ecol. Evol. 11:1205697. doi: 10.3389/fevo.2023.1205697
Yu, Y., Feng, J., Liu, H., Wu, C., Zhang, J., Wang, Z., et al. (2023). Linking hydrological connectivity to sustainable watershed management in the loess plateau of China. Curr. Opin. Environ. Sci. Health 35:100493. doi: 10.1016/j.coesh.2023.100493
Zhang, Y., Huang, C., Zhang, W., Chen, J., and Wang, L. (2021). The concept, approach, and future research of hydrological connectivity and its assessment at multiscales. Environ. Sci. Pollut. Res. 28, 52724–52743. doi: 10.1007/s11356-021-16148-8
Zhang, Y., Jiang, J., Zhang, J., Zhang, Z., and Zhang, M. (2022). Effects of roots systems on hydrological connectivity below the soil surface in the yellow river delta Wetland. Ecohydrology 15:e2393. doi: 10.1002/eco.2393
Zimmer, M. A., Kaiser, K. E., Blaszczak, J. R., Zipper, S. C., Hammond, J. C., Fritz, K. M., et al. (2020). Zero or not? Causes and consequences of zero-flow stream gage readings. Water 7:e1436. doi: 10.1002/wat2.1436
Zimmer, M. A., and McGlynn, B. L. (2018). Lateral, vertical, and longitudinal source area connectivity drive runoff and carbon export across watershed scales. Water Resour. Res. 54, 1576–1598. doi: 10.1002/2017WR021718
Keywords: hydrologic connectivity, interdisciplinary collaboration, catchment dynamics, climate change adaptation, geomorphological processes, ecohydrological models, environmental sustainability, ecosystem resilience
Citation: Dwivedi D, Poeppl RE and Wohl E (2025) Hydrological connectivity: a review and emerging strategies for integrating measurement, modeling, and management. Front. Water 7:1496199. doi: 10.3389/frwa.2025.1496199
Received: 13 September 2024; Accepted: 04 March 2025;
Published: 31 March 2025.
Edited by:
Timothy O. Randhir, University of Massachusetts Amherst, United StatesReviewed by:
Tianfang Xu, Arizona State University, United StatesCopyright © 2025 Dwivedi, Poeppl and Wohl. This is an open-access article distributed under the terms of the Creative Commons Attribution License (CC BY). The use, distribution or reproduction in other forums is permitted, provided the original author(s) and the copyright owner(s) are credited and that the original publication in this journal is cited, in accordance with accepted academic practice. No use, distribution or reproduction is permitted which does not comply with these terms.
*Correspondence: Dipankar Dwivedi, RER3aXZlZGlAbGJsLmdvdg==
Disclaimer: All claims expressed in this article are solely those of the authors and do not necessarily represent those of their affiliated organizations, or those of the publisher, the editors and the reviewers. Any product that may be evaluated in this article or claim that may be made by its manufacturer is not guaranteed or endorsed by the publisher.
Research integrity at Frontiers
Learn more about the work of our research integrity team to safeguard the quality of each article we publish.