- 1Institute of Physical Geography, Goethe University, Frankfurt am Main, Germany
- 2Institute for Social-Ecological Research, Frankfurt am Main, Germany
- 3Senckenberg Leibniz Biodiversity and Climate Research Centre Frankfurt (SBiK-F), Frankfurt am Main, Germany
Groundwater sustains human well-being and ecosystems functioning. Many regions in Europe have experienced declining groundwater levels caused by decreasing groundwater recharge (GWR) or increasing groundwater abstractions (GWAs). These changes can lead to groundwater-related stress, threatening ecosystems and water supplies. Existing groundwater stress indicators estimate stress during a given period but do not address how stress changes or show the uncertainty of future stress. We propose a novel indicator of future groundwater stress (GWSI) due to changes in GWR and GWA and, thus, the alteration of long-term mean annual groundwater discharge (GWD). Groundwater stress is defined as any alteration in GWD since ecosystems are adapted to an equilibrium state. Focusing on decreasing GWD, which is generally more harmful than increasing GWD, we quantified the future GWSI in Europe by integrating scenarios of GWR and GWA in 2070–2099. GWR was evaluated using an ISIMIP2b multi-model ensemble of eight global hydrological models driven by the output of four global climate models under two greenhouse gas emission scenarios. GWA scenarios for irrigation, domestic and manufacturing sectors were combined with the GWR projections to generate an ensemble of GWSIs, simplified into three groundwater stress scenarios (high, intermediate, low). Projected GWSIs vary significantly among the scenarios. For the high-stress scenario, 58% of Europe’s land area is projected to experience a GWD decrease of at least 25% under RCP8.5 compared to 38% under RCP2.6, while the respective values are 26 and 1% for the intermediate-stress scenario. Groundwater demand management alone might not prevent GWD declines under the high-stress and intermediate scenarios, particularly under RCP8.5. Therefore, climate change mitigation might imperative for reducing the decline of GWD, especially in Eastern and Southeastern Europe, where changes in GWR are projected to be the primary cause of declining GWD (in the high abstraction scenario under RCP8.5). Under RCP2.6, reductions in GWAs by 25–75% might balance a GWD decline in parts of Spain and Italy where GWAs are high, even in the high-stress scenario. In line with the precautionary principle, we recommend adapting to the high-stress scenario to minimize harm to the beneficiaries of groundwater.
1 Introduction
Worldwide, the well-being of societies and economies depends on high-quality freshwater resources. Groundwater serves as a major source for drinking water supply, agricultural irrigation and manufacturing (Müller Schmied et al., 2021). In Europe, 24.5% of total freshwater abstractions occurred from groundwater in 2017 (European Environment Agency, 2021) and constitutes the source of 25 and 65% of the water abstractions for irrigation and public water supply, respectively (European Environment Agency, 2022). Several European countries, such as Germany, strongly rely on groundwater for their public water supply, accounting for two-thirds of the total abstracted water (Federal Statistical Office of Germany, 2024), while others, e.g., South and Southeastern European countries, use groundwater mainly for irrigation (Gelati et al., 2020). Concerns about groundwater resources in Europe have arisen in recent years not only in regions well-known for groundwater over-extraction (mainly for irrigation), such as Southern Spain and Greece (Gelati et al., 2020), but also from observed declining groundwater levels in Germany (Donheiser et al., 2022), the Netherlands, Belgium, Luxembourg, Denmark, and Poland (Verkaik et al., 2024) and dominant groundwater storage decline in Central and Eastern Europe as observed by combining satellite observations and re-analysis (Xanke and Liesch, 2022). Simultaneously, increasing climate variability and drought frequency due to climate change (Hänsel et al., 2019; Ionita et al., 2020) is expected to lead to more reliance on groundwater resources since groundwater serves as a natural interseasonal and interannual storage of freshwater (Scanlon et al., 2023; Stigter et al., 2023).
An assessment of present and future groundwater resources in Europe requires, in the first place, a quantification of renewable groundwater resources and human water use. Renewable groundwater resources can be approximated by using the long-term average diffuse groundwater recharge (GWR), i.e., the addition of water to the water-saturated zone below the groundwater table from the overlying unsaturated zone (Döll, 2009). However, differing from total renewable water resources, which can be determined based on streamflow observations, renewable groundwater resources cannot be estimated from GWR measurements (Smerdon and Drewes, 2017). Direct recharge measurements are difficult to conduct and can only provide very small-scale local estimates and, as such, modeling approaches remain indispensable (Wan et al., 2024). European-wide gridded simulations of GWR, mostly at a spatial resolution of 0.5°, are available, although with high uncertainty, from European-scale or global hydrological models (GHMs) (Reinecke et al., 2021), while a high-resolution static estimate of renewable groundwater resources across Europe have been derived from national estimates for seven countries (Martinsen et al., 2022). Regarding the impact of human water use, groundwater resources are affected by groundwater abstractions (GWAs) and return flows on areas that are irrigated by water that was either abstracted from groundwater or surface water. In addition, only a few GHMs provide the gridded estimates of sectoral GWAs and return flows to groundwater (Telteu et al., 2021); these are mostly based on the irrigated area and climate in the case of irrigation water use, and country statistics and population distribution in the case of domestic and manufacturing water use.
Thus, while quantification of the recent historical situation of groundwater resources is already uncertain (Herbert and Döll, 2019), potential future changes will introduce even greater levels of uncertainty (Earman and Dettinger, 2011). It is state-of-the-art to assess future climate change impacts and their uncertainty by multi-model ensembles (MME), where several hydrological models are driven by the bias-adjusted output of some global climate models (GCMs) (Döll et al., 2015). For instance, projected changes in global GWR differ strongly, even for specific future greenhouse gas emissions (Reinecke et al., 2021; Gnann et al., 2023), and are mainly due to uncertain precipitation patterns (Fallah et al., 2020) and evapotranspiration responses from vegetation to increasing atmospheric carbon dioxide levels (active vegetation) (van Roosmalen et al., 2009; Milly and Dunne, 2016). In Germany, for example, one study indicates a decline in groundwater levels under climate change (Wunsch et al., 2022), while others suggest that GWR will either increase or remain constant (Marx et al., 2022; Riedel et al., 2023). In England and Wales, while research indicates stable annual recharge rates (Hughes et al., 2021), climate change is projected to shorten the recharge season (Mansour and Hughes, 2017), intensifying the risk of groundwater droughts due to the concentration of recharge within a narrower time frame (Hughes et al., 2021). Uncertainties in GWR projections are also evident for France, with a projected average increase of +15% by the end of the century and a variance across five climate projections, with GWR alterations ranging from −3 to +41% (Vergnes et al., 2023). Furthermore, Piniewski et al. (2022) reviewed discrepancies between projected future low stream flows (which can be seen as a proxy for long-term GWR) and historical observations across Central Europe. Altough most historical observations show a downward trend in low flows and increasing drought risk, projections suggest a smaller decrease or even an upward trend for future low flows compared to historical observations.
Societal water demands, and thus GWAs and return flows, are changing due to climate change and socio-demographic factors such as population dynamics, increasing wealth, technological advancements and evolving individual behaviors (Thissen et al., 2017; Lüdtke et al., 2021). As a result, this adds pressure on groundwater resources and introduces further uncertainty in the assessments of future groundwater resources. Thus, as temperatures rise and potential evapotranspiration increases, a greater need for agricultural irrigation may occur unless precipitation rises (Taylor et al., 2013; Riedel and Weber, 2020). This heightened demand for irrigation is already evident in Germany where GWAs for this purpose have steadily increased since 2010, peaking in the dry years 2018 and 2019 (Federal Statistical Office of Germany, 2023). Domestic water abstractions per capita (from both surface water and groundwater), however, decreased in Europe between 2000 and 2008, thus, if this trend continues, then this may possibly lead to a decrease in domestic water abstractions by 2030 (Vandecasteele et al., 2014). The GWA patterns within the manufacturing industry exhibit significant heterogeneity across various subsectors, posing challenges in forecasting future trends (Luetkemeier et al., 2022; Mannix et al., 2022).
The increasing reliance on groundwater resources due to climate change, as well as the uncertainties of future climate and societal developments, underscores the need for groundwater management that sustains groundwater for both human and ecosystem needs (Saccò et al., 2024). Groundwater-related ecosystems are adapted to long-term natural conditions, thus, any alteration of hydrological fluxes, such as GWR and GWAs, disrupt the dynamic equilibrium of the system (Poff et al., 1997) making it necessary for ecosystems and humans to adapt to the ensuing hydrological changes. Therefore, the alteration of groundwater discharge (GWD) due to GWR changes caused by climatic changes and/or GWAs may increase the risk for groundwater-dependent ecosystems and other beneficiaries of groundwater (Herbert and Döll, 2019). Therefore, keeping groundwater levels and, thus, storage at its current level (or increasing it to its natural level in case of groundwater depletion) becomes crucial to sustain ecosystem functioning and, hence, the important ecosystem services for humans. Decreasing groundwater levels lead to decreasing GWD to surface water bodies, threatening environmental flows in rivers and streams and other groundwater-dependent ecosystems such as wetlands, springs and marine ecosystems (Kløve et al., 2011; Griebler and Avramov, 2015). In addition, they may negatively affect the water supply to vegetation such as forests (Skiadaresis et al., 2021). Decreasing groundwater levels also impact the groundwater ecosystem itself, a system that provides a unique habitat for groundwater fauna (stygofauna) (Griebler et al., 2014; Hahn et al., 2018; Becher et al., 2022; Pop et al., 2023) as well as important ecosystem services to maintain high-quality groundwater crucial for human water supply (e.g., purification) (Griebler and Avramov, 2015). While decreasing groundwater levels are generally more hazardous for ecosystems and the water supply, increasing GWD and thus groundwater levels, may also have negative impacts, such as basement flooding or wet soils, which complicates agricultural use.
To assess the extent to which natural groundwater resources have been altered and put under stress by human water use, groundwater stress indicators can be quantified. Groundwater stress indicators have already been computed on global (Gleeson et al., 2012; Richey et al., 2015; Herbert and Döll, 2019) and regional scales (Gleeson and Wada, 2013; Ashraf et al., 2017; Tabarmayeh et al., 2022), mostly for the current conditions. The most widely employed indicator is the ratio of GWAs and renewable groundwater resources, with variations on the definitions of the two components (Akbar et al., 2022). Gleeson et al. (2012), for example, added return flows from irrigation to the renewable groundwater resources from diffuse GWR and subtracted an environmental flow requirement. The environmental flow requirement was estimated as the monthly streamflow exceeded 90% of the time; groundwater stress was defined to occur if this ratio exceeded 1. Only Herbert and Döll (2019) determined the potential future changes in groundwater stress as a function of uncertain future climate change and GWAs. They quantified groundwater stress as the ratio of net GWAs (GWAs minus return flows) to GWR, in units %. However, a future change in this stress indicator is difficult to interpret because the change needs to be expressed in percentage points (as it is the difference between two percentages). In addition, ratio-based indicators have been criticized for falsely assuming that GWAs are balanced by GWR, while, in reality, they are balanced by an increase in groundwater capture (Barlow and Leake, 2012), particularly a decrease in GWD to surface water bodies (Herbert and Döll, 2019), thus an improved indicator for future groundwater stress is required. In addition, Herbert and Döll (2019) did not identify ways to present the uncertainty of future GWR and, hence, groundwater stress that is necessary to support climate change adaptation (Crosbie et al., 2013). They only showed ensemble mean values of change and used the worst-case scenario for the identification of groundwater stress hot spots. As a result, suitable approaches for representing the full range of uncertainty due to the translation of greenhouse gas emissions into GWR and irrigation requirements, and how to combine these uncertainties with groundwater use scenarios, are needed.
This paper aims to support resilient groundwater management under uncertain climate and socio-economic changes in Europe. It integrates GWR and GWA scenarios to assess future groundwater stress at the pan-European scale by a novel groundwater stress indicator that quantifies stress in terms of future changes in GWD to groundwater-dependent ecosystems. Differing from previously developed indicators, stress is assumed to occur in the case of any alteration in GWD and, thus, groundwater levels and storage because ecosystems are adapted to long-term natural conditions. The following research questions will be addressed in the article:
1. How can groundwater stress be assessed in the future considering evolving climate change and socio-economic conditions (Section 2.3)?
2. What is the range of present and future GWR and GWA projections under different climate change and socio-economic scenarios (Section 3.1 and Section 3.2)?
3. What is the range of future alterations of GWD projections caused by changes in GWR and GWAs (Section 3.3)?
4. What potential mitigation scenarios (climate change mitigation policies or abstraction regulations) might prevent groundwater stress in the future (Section 3.5)?
5. What is the advantage of the newly developed groundwater stress indicator in comparison to existing ones (Section 4.1)?
2 Methods and data
The quantitative analysis in this study was computed using R, version 4.3.1 (R Core Team, 2023). The scripts for the data analysis and the visualizations, including the used packages, are publicly available (see Section Data availability Statement). All figures were produced color blindness friendly with the “scico” package (Pedersen and Crameri, 2023), following the recommendations of Crameri et al. (2020).
2.1 Multi-model ensemble of groundwater recharge under climate change
This study uses the output of an MME of GHMs driven by the bias-adjusted output of GCMs to assess future GWR alterations. The output is available at a spatial resolution of 0.5° (approximately 50 km). The MME used in this study, which has been previously evaluated regarding changes of GWR at the global scale by Reinecke et al. (2021), consists of four bias-adjusted GCMs (MIROC5, GFDL-ESM2M, HadGEM2-ES, IPSL-CM5A-LR) simulating the present and future climate conditions (RCP2.6 and RCP8.5) and eight GHMs (CLM4.5, CWatM, H08, Jules-W1, LPJmL, MATSIRO, PCR-GLOBWB, WaterGAP2.2c) that simulated diffuse GWR. GHM model simulations were performed according to the Inter-Sectoral Impact Model Intercomparison Project (ISIMIP) 2b protocol (Frieler et al., 2017; Gosling et al., 2023). Half of the GHMs used in the MME consider active vegetation, in particular the effect of increasing carbon dioxide concentrations on vegetation transpiration (Supplementary Table 1), a complex and highly uncertain process that is, therefore, neglected in some GHMs and hydrological models with a local or regional geographic scope (Schwingshackl et al., 2019). Not considering active vegetation in GHMs may result in underestimating future total runoffs (Milly and Dunne, 2016; Peiris and Döll, 2023) and, thus, GWR. However, for a few regions, it may lead to an overestimation (Milly and Dunne, 2016; Reinecke et al., 2021).
We used monthly diffuse GWR data (variable “qr,” 2005soc runs in ISIMIP2b simulation round) for the period 2006–2099. In the ISIMIP2b protocol, the climate change projections under alternative greenhouse gas emission scenarios (RCPs) start with the year 2006. In the GHM runs, the levels of population, economic development, land use and management were fixed at their levels from 2005 in the 2005soc runs in order to simluate only the effect of future climate change on GWR (Frieler et al., 2017) (Table 1). We evaluated two greenhouse gas emission scenarios: the low emissions scenario RCP2.6 and the high emissions scenario RCP8.5. The mean annual GWR from a monthly time series of GWR from the ISIMIP2b data repository were computed for each GCM-GHM model combination, RCP and 0.5° grid cell for two 30-year time periods, to quantify renewable groundwater resources under the “present” and “future” climate conditions. With the ISIMIP2b simulation setup, it is best to select as a reference period for climate change impact assessments, the 30-year “present” time period from 2006 to 2035 since all water use components remain constant at the year 2005. For the future, the far-future period 2070–2099 was chosen. Negative monthly GWR values, which represent capillary rise and are computed by MATSIRO and CWatM, were set to 0, in both the present and the future periods. The ensemble for the RCP2.6 scenario comprised 32 ensemble members. Since not all GHMs compute the RCP8.5 scenario, the ensemble for this RCP included only 28 ensemble members.
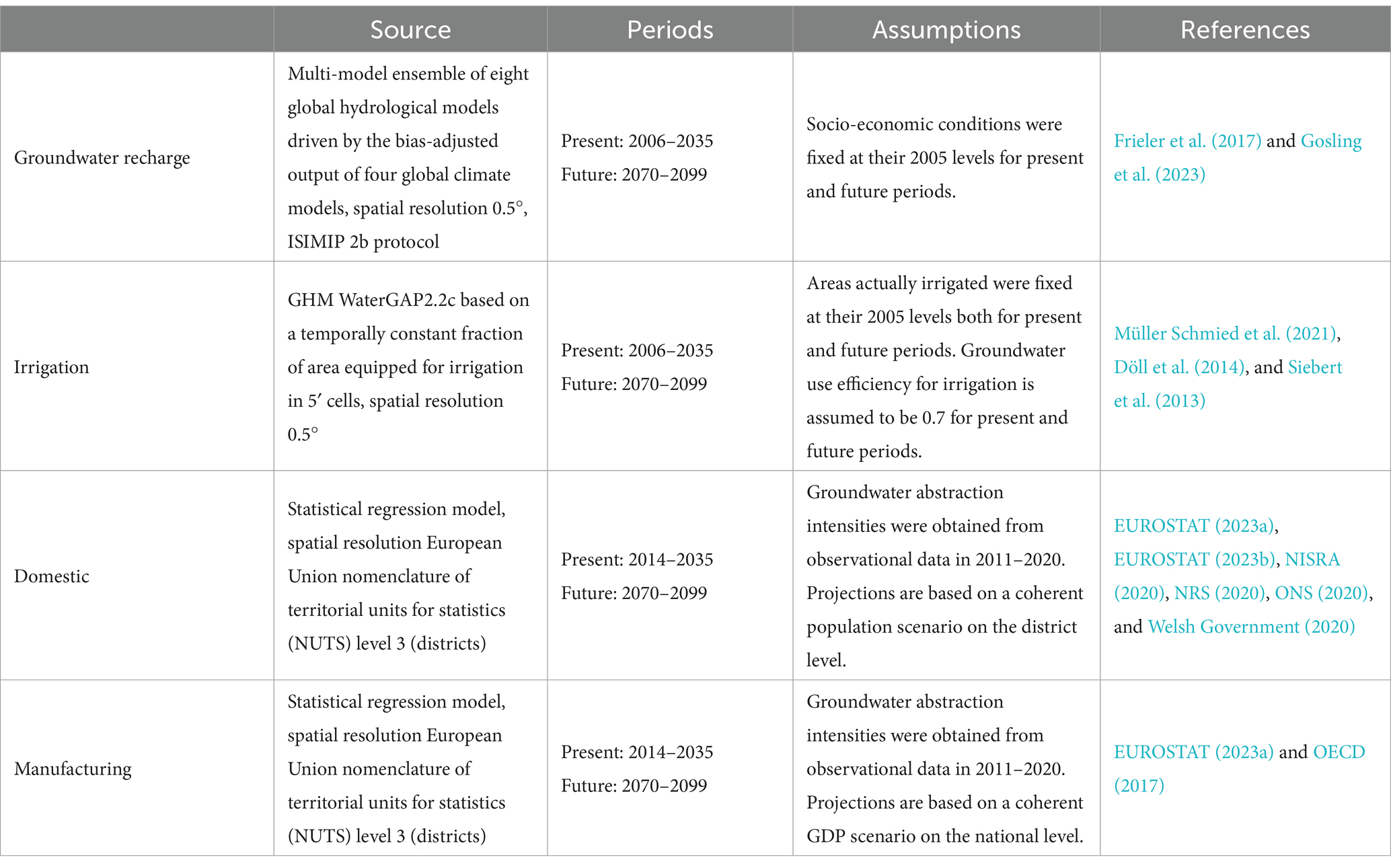
Table 1. Sources, periods and assumptions used in the simulation of sectoral groundwater abstraction and groundwater recharge.
To quantify GWR for the period 2006–2035, the average of the long-term mean annual GWR values for RCP2.6 and RCP8.5 per GCM-GHM model combination were computed, while for the future period, the climate change scenarios were considered separately in order to distinguish the effect of the different emission scenarios by the end of the century. From the long-term mean annual GWR values of the MME, the P10 (10% of the recharge values lie below and 90% above this value), P50 (50% of the recharge values lie below and 50% above this value) and P90 (90% of the recharge values lie below and 10% above this value) values were computed to represent the uncertainty range of GWR for the present period. Relative GWR changes of future GWR projections per ensemble member, i, were then determined by comparing the long-term mean annual averages of GWR (in mm yr.−1) for each GCM-GHM combination between the future ( ) and the present period ( ). Relative GWR changes per GCM-GHM combination were set to N/A if GWR in the period 2006–2035 was <1 mm yr.−1. The three percentiles (P10, P50, P90) of the long-term changes of future GWR projections were then calculated per grid cell to account for the uncertainties among the future changes of GWR. The range of possible GWR projections (changes) was considered in the visualization by consistently presenting the three percentiles among the MME per cell, effectively illustrating the uncertainty range and facilitating the comparison of potential future GWR simulations.
2.2 Groundwater abstractions
The water demands and resulting abstraction patterns of the irrigation, public water supply (domestic) and manufacturing sectors build upon certain sector-specific logics, thus making it necessary to assess each sector’s GWAs separately for the present conditions and future scenarios (Müller Schmied et al., 2022). Observational data on total and sectoral GWAs are rare and are often only available at the national level (e.g., FAO Aquastat, EUROSTAT). Therefore, sectoral GWAs in the present and future periods are computed by using different approaches, with different spatial resolutions and temporal extents (Table 1). For the irrigation sector, the output of a GHM was used to estimate the present and future GWAs in 0.5° grid cells, based on the irrigated area and climate since GWA data from actual measurements are scarcely available (Condon et al., 2021) (Section 2.2.1). For domestic and manufacturing GWAs, national statistics were used to estimate groundwater use intensities; these were applied to simulate the GWAs at the district level using the historic and projected populations and gross domestic product (GDP) data at this level. The results were then scaled to the grid cell resolution (0.5° × 0.5°) to ensure spatial resolution consistency between the irrigation GWAs and GWR data. Relative changes in future GWA projections compared to the present period were determined using long-term mean annual averages (30-year periods).
2.2.1 Irrigation sector
WaterGAP’s Global Irrigation Model (GIM) computes crop-specific irrigation water consumption for each 0.5° grid cell (only distinguishes between rice and non-rice crops), based on irrigated areas and climate (Müller Schmied et al., 2021). The irrigated area per grid cell in the GIM is based on areas equipped for irrigation (AEI) with a spatial resolution of 5′ (arc-minute) grid cells (Siebert et al., 2015). Country-specific ratios of area actually irrigated (AAI) to AEI are utilized to estimate the AAI in each grid cell, considering that not all of the AEI is used for irrigation every year. The AAI/AEI ratios were derived from the Global Map of Irrigation Area for 2005 (Siebert et al., 2013). The crop-specific irrigation consumptive water use per irrigated area is computed during the growing season (based on long-term average temperature, precipitation and potential evapotranspiration) as the difference between crop-specific optimal evapotranspiration and effective precipitation (Müller Schmied et al., 2021). The specific groundwater use fractions for irrigation can be applied to estimate consumptive water use from groundwater and, based on irrigation water use efficiencies, the GWAs were simulated (Siebert et al., 2010). The groundwater use efficiency for irrigation globally is assumed to be 0.7 (Döll et al., 2014), which means that 70% of the abstracted groundwater is consumptively used for irrigation purposes and the remaining part returns to the groundwater. Therefore, in this study, net abstractions of groundwater (abstraction minus return flows of applied irrigation water to the groundwater) for irrigation (GWNAirrig) is computed as 70% of the total groundwater abstractions for irrigation. For the present and future periods of GWNAirrig, the AAI were held temporally constant at the year 2005 (Müller Schmied et al., 2021).
The uncertainty in estimating GWNAirrig caused by climate change can be considered by using the bias-adjusted output of the same GCMs (MIROC5, GFDL-ESM2M, HadGEM2-ES, IPSL-CM5A-LR) used for computing GWR to force WaterGAP, representing present and future GWNAirrig under different climate conditions (RCP2.6 and RCP8.5). GWNAirrig were computed per GCM (four model members) and RCP scenario based on the monthly GWA volumes from WaterGAP 2.2c. Similar to GWR, in the present period, the mean between the RCP2.6 and RCP8.5 GWNAirrig were computed per GCM, while for the future period, the climate change scenarios were considered separately in order to distinguish the effect of the different emission scenarios by the end of the century (Table 2). From GWNAirrig per cell, the minimum, median and maximum abstraction values among the four GCMs were obtained to represent the uncertainty range for the present period. The minimum, median and maximum values of the relative changes between the future and the present abstraction volumes per cell among the four GCMs were obtained to present the uncertainty range of GWNAirrig in the future (Table 2). The median of the four GCM values, both for the present and future periods, was computed using the “quantile” function from R (R Core Team, 2023).
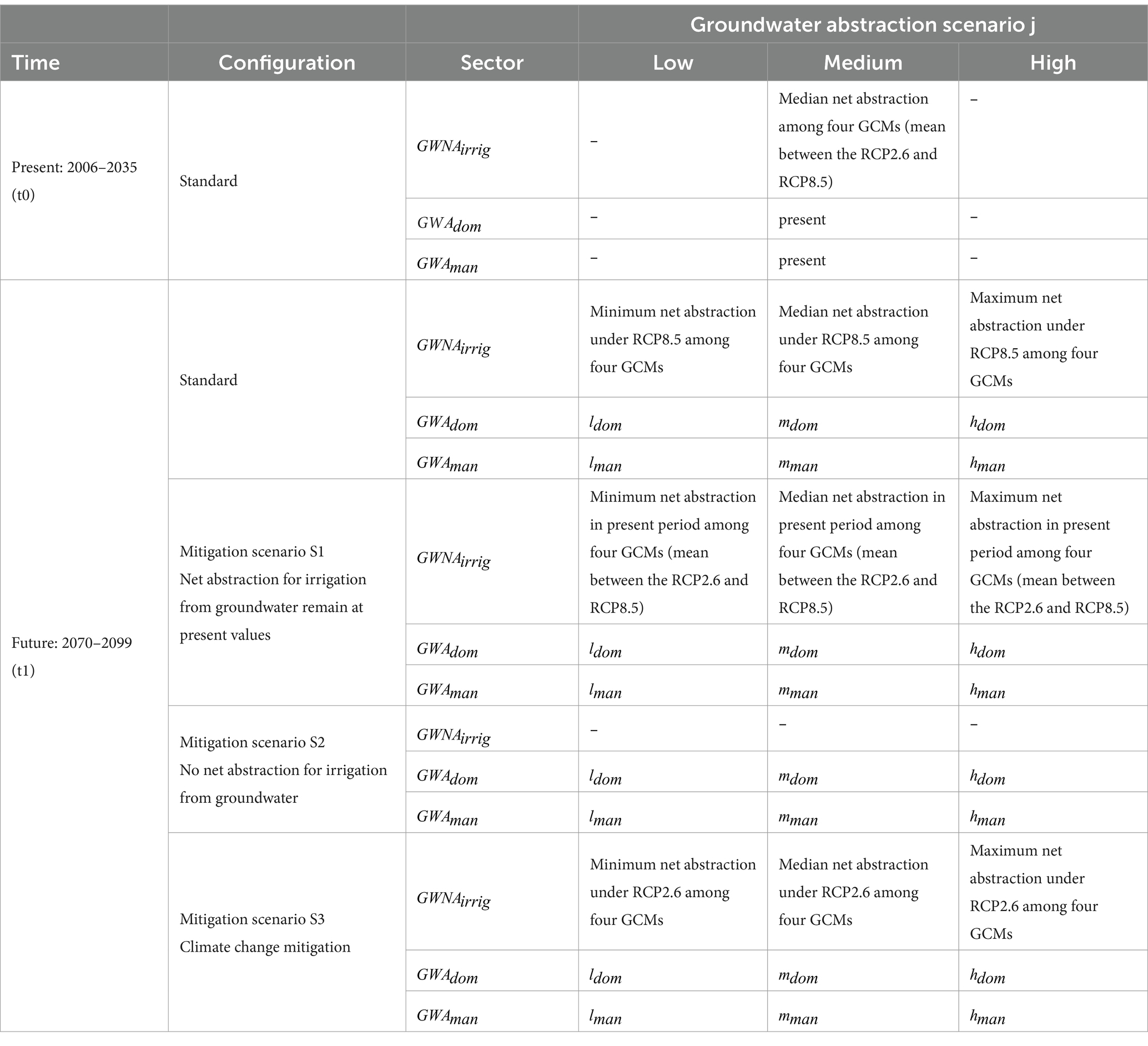
Table 2. Configuration of groundwater abstraction scenarios including the groundwater mitigation scenarios S1, S2 and S3. l, m, and h refer to the groundwater abstraction scenarios of low, medium and high for the domestic and manufacturing sectors, respectively.
2.2.2 Domestic and manufacturing sectors
In the domestic sector, groundwater is abstracted for the water supply of private households and small businesses. GWAs may put significant pressure on groundwater resources in regions of high population densities (Ahmadi et al., 2020). Likewise, the manufacturing sector may put pressure on groundwater resources in regions with high industrial intensity (Zheng et al., 2021). In contrast to the domestic sector, manufacturing GWA patterns are characterized by a strong inherent heterogeneity due to the various subsectors (Luetkemeier et al., 2022; Mannix et al., 2022). Since GHMs, for example, those available on the ISIMIP repository, do not incorporate recent projections for the population and GDP data at the district level (Flörke et al., 2013), a regression model was developed to simulate domestic and manufacturing GWAs at the European district levels (NUTS-3, Table 1) using the latest official data projections. Each sector’s specific GWA intensity ( ) link the abstracted groundwater volumes to explanatory variables. For the domestic sector, gwi is the ratio of GWAs to the population, while for the manufacturing sector, the explanatory variable is GDP. For further processing, country-specific gwi values were calculated from national time series data for the population, GDP and groundwater abstractions (2011–2020) obtained from the EUROSTAT database (EUROSTAT, 2023a). The final gwi values at the country level were calculated as medians from the annual time series. The resulting national GWA intensities were then multiplied with the district level values of the explanatory variables for the population or GDP, respectively, available from 2014 onwards. Subsequently, data for the historic and future populations and the GDP values were compiled, based on the observational data and official institutional projections. The projection for population development is available at the district level for EU members (EUROSTAT, 2023b) and non-EU members (NISRA, 2020; NRS, 2020; ONS, 2020; Welsh Government, 2020), while the projection for GDP development is available at the national level for both EU and non-EU members (OECD, 2017). The application of to the district level followed Equation (1):
Here, is the annual GWA in a certain district, d, at = 2014. The resulting district level GWA data was transformed to the spatial resolution of 0.5° via spatially weighted averages for further computation. GWA scenarios were employed to account for future uncertainty in the GWAs. The country-specific sectoral GWA intensities were tuned by the scenario factor, [low ( , medium ( or high ( abstraction scenarios], to account for technological and behavioral changes in GWA patterns in the future. , the annual GWAs in a certain district, d, at time in the future, was computed with an annual time step following Equation (2):
where the scenario factor s was obtained by extrapolating national trends between 2011 and 2020 up to 2099. For the domestic sector, showed an annual change of 0.04%, leading to an increase of gwi by 5% above today´s average for the high abstraction scenario until the end of the century, if this rate remained constant (Table 3). Based on the slight changes in gwi for the domestic sector in the past, an uncertainty envelope of only −5% ( ) and + 5% ( ) was assumed until 2099, respectively. For the medium scenario, no changes in were assumed to occur ( , 0%). For the manufacturing sector, recent data show a strong decline with annual changes of −2%, leading to the assumption that gwi could be improved by −75% until the end of the century ( ) and may only stay at today’s levels in the high scenario ( , 0%). The medium case for manufacturing consists of a reduction of −50% ( ).
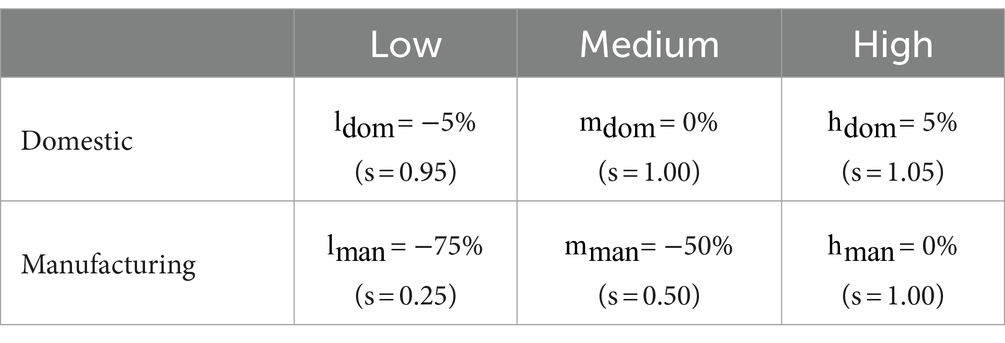
Table 3. Scenario factor s used for the groundwater abstraction scenarios for the domestic and manufacturing sectors up to 2099.
2.3 Groundwater stress as indicated by groundwater discharge alterations
While most groundwater stress indicators relate GWR and GWAs only, groundwater storage (GWS) and, thus, the groundwater level, does not depend solely on GWR and GWAs, but also on GWD from aquifers into streams, wetlands or springs (Figure 1) (Taylor and Alley, 2001; Barlow and Leake, 2012), which can be described with Equation (3):
where t is time.
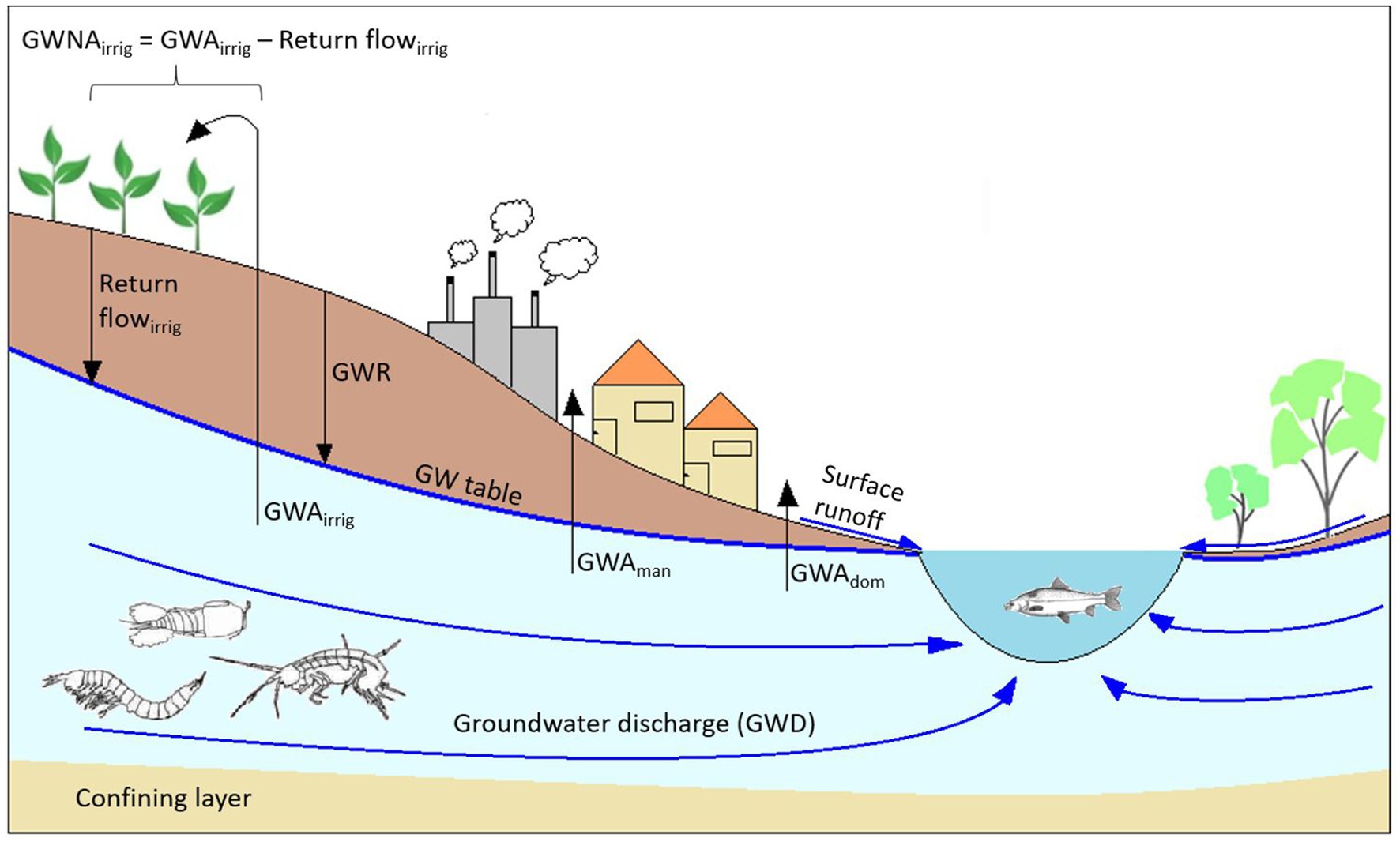
Figure 1. Schematic figure of the hydro(geo)logical processes focused on in the analysis (modified from Taylor and Alley, 2001, p. 4). GW, groundwater; GWR, groundwater recharge; GWA, groundwater abstractions; GWNA, groundwater net abstractions; irrig, irrigation; man, manufacturing; dom, domestic. (Schematic fauna illustrations: Koch et al., 2021 and Research Institute for Agriculture and Fisheries Mecklenburg-Western Pomerania, 2024).
GWD is often assumed to be linearly correlated with GWS (Telteu et al., 2021). Previous studies have shown that GWAs have significantly decreased GWS and GWD to streams worldwide (Herbert and Döll, 2019), leading to environmental stress for riverine ecosystems and that this is likely to increase in the future (de Graaf et al., 2019). Moreover, a decrease in GWD, which can be assumed to indicate a decrease in GWS and, in case of an unconfined aquifer, also a lowering of the groundwater table (Barlow and Leake, 2012; de Graaf et al., 2019), decreases the habitat size for biota and microorganisms living in both confined and unconfined aquifers; this is important since these organisms provide valuable ecosystem services (e.g., purification) (Griebler and Avramov, 2015). Herbert and Döll (2019) introduced a groundwater stress indicator that quantified the alterations of GWD during a specific time period due to human water use. However, their study did not include future changes in GWD. Thus, we propose to consider GWD as the crucial variable for indicating water availability for all beneficiaries of groundwater, including plants, animals and humans (Figure 1), and to identify any future changes of GWD as groundwater stress caused by future changes in both GWR and GWAs by climate, demographic or socio-economic change. In our study, mean annual GWD per model member, i, and GWA scenario, j, both in the present and future periods, is computed using Equation (4):
with GWA computed with Equation (5):
where GWR in the present period indicates the mean annual GWR for each ensemble member, i, in the present period (mean between the RCP2.6 and RCP8.5 long-term annual mean of GWR values per ensemble member, Section 2.1), and the GWAs in the present period are the total net abstraction from groundwater for the three sectors in the present period. GWAs in the present period consist of the median of GWNAirrig among the four GCMs (mean between the RCP2.6 and RCP8.5 GWNAirrig per GCM) for the present period and the present GWAs for the domestic and manufacturing sectors (Table 2). Use of Equation 4 for computing GWD assumes steady-state conditions during the 30-years of the present and future period while groundwater storage can change between the periods. The steady-state assumption is not valid if GWA is larger than GWR during any 30-year period and continuous depletion of GWS balances a GWD decline. In this case, our study only indicates the occurrence of groundwater depletion but does not quantify the GWD decline.
An ensemble of future GWDs under each RCP, with the ensemble members combining an ensemble member, i, for GWR and a GWA scenario, j, was computed using future GWR values for each GWR ensemble member under each RCP and future GWA scenarios, (j = low, medium or high GWA scenario) for the domestic, manufacturing and irrigation sectors (Table 2). An ensemble of groundwater stress (GWSI) was then computed based on the relative changes of GWD, following Equation (6):
The uncertainty due to both the uncertain future GWR and GWAs is, thus, quantified by the ensemble of the change of the GWD, i.e., of . To facilitate communication of the modeling results and their uncertainty, we simplified the GWSI ensemble by defining three groundwater stress scenarios per RCP. The “low stress” scenario was determined, for each grid cell, as the P90 of all the GWSI ensemble members that were computed with GWAs in the case of the low GWA scenario, as described in Table 2. The P90 values represent projections of either comparably high increases or low decreases of GWD. As groundwater stress is mostly affected by the change in GWR, this corresponds, approximately, to combining the high projections of future GWR with low future GWAs. The “high stress” scenario was computed as the P10 of all the GWSI ensemble members that were computed with GWAs in the case of the high GWA scenario. It is the scenarios with low increases or high decreases of GWD that can be regarded as those that should be taken into account under the precautionary principle. The “intermediate stress” scenario was derived from the median GWSI of the ensemble members computed with GWAs in the case of the medium GWA scenario.
2.4 Mitigation scenarios
Any major change in GWD can lead to stress for both humans and ecosystems since they are adapted to an ecological equilibrium, thus sustaining ecological functioning and important ecosystem services. Increases in GWD, which reflect increases in the groundwater level, may cause the flooding of basement or wet soils that are consequently more difficult to use for agriculture. However, local solutions, such as drainage or waterproofing basements, should be considered in these cases instead of increasing GWAs. Decreases in GWD, however, have more potential negative impacts than increases. The most important risks are those for the human water supply, loss of habitat for groundwater biota and loss of habitat for biota in groundwater-dependent wetlands and in rivers. Therefore, three scenarios were developed and simulated to assess the effects of abstraction regulations (S1 and S2) and climate change mitigation policies (S3) on GWD, with the aim to identify potential actions to prevent negative GWD in the future (i.e., GWSI <0) (Table 2).
2.4.1 Mitigation scenario 1 (S1): future irrigation groundwater net abstractions remain at present volumes
Given the increasing irrigation demands and decreasing water availability due to climate change (Ruppaner, 2021), one potential future scenario to avoid decreasing GWD involves maintaining the present levels of GWNAirrig while implementing water-saving techniques to enhance irrigation efficiency (Fabbri et al., 2016), rather than allowing them to increase in response to climate change. Hence, GWSI under the RCP8.5 scenario was computed using future long-term mean annual GWR values for each ensemble member and future groundwater (net) abstraction scenario, where the future GWNAirrig remained at the present net abstraction volume (Table 2).
2.4.2 Mitigation scenario 2 (S2): no future groundwater irrigation
An extreme scenario could involve no groundwater irrigation in the future to avoid decreasing GWD. Hence, GWSI under the RCP8.5 scenario was computed using future long-term mean annual GWR values for each ensemble member and future GWA scenario where GWNAirrig values were excluded (Table 2).
2.4.3 Mitigation scenario (S3) 3: climate change mitigation to Paris agreement (RCP2.6)
To quantify the impact of climate change mitigation, GWSI was determined for S3 using GWR and GWNAirrig for RCP2.6 (instead of RCP8.5), in the standard scenario and future GWA scenarios, wherever GWNAirrig under the RCP2.6 scenario was used (Table 2).
2.5 Causes of decreasing groundwater discharge
To evaluate the extent to which groundwater demand management can reduce any decrease in GWD, the contribution ( ) of future GWA changes to GWD changes (if negative) was computed for each ensemble member, I, and GWA scenario, j, following Equation (7):
The required relative reduction of future GWAs, in percent, to avoid decreasing GWD under the three groundwater stress scenarios was computed following Equation (8):
with GWDt0 computed following Equation (9):
consists, in this case, of the difference of the median of long-term annual mean GWR values among the MME in the present period ( ) and GWAs in the present period (Table 2). The threshold in eq. 8 was introduced to disregard high relative reductions of GWAs caused by low abstraction values in the present period.
3 Results
3.1 Groundwater recharge
GWR projections for the present period (2006–2035) exhibit a wide range among the MME (Figure 2). In Southern and Eastern Europe as well as in Norway, the range between the P90 and the P10 values may be at least twice as high as the P50 (Figure 2d), indicating large uncertainties in the GWR estimations within the MME in those areas. The P50 of the MME indicates low recharge values in Southern and Southeastern Europe, while those of Central and Northern Europe range between 100 and 400 mm yr.−1, except for areas such as the Alps, UK and the Norwegian coast which show higher recharge values exceeding 400 mm yr.−1. Despite the uncertainties mentioned, comparing the present GWR values obtained from the MME with those derived from a recent Europe-wide study (utilizing satellite data and national surveys at a spatial scale of 1 × 1 km) illustrates that the P50 of the MME aligns with the spatial distribution of high (in the Alps and the Norwegian coast) and low (in Southern and Southeastern Europe) potential GWR values across most of Europe, as analyzed by Martinsen et al. (2022).
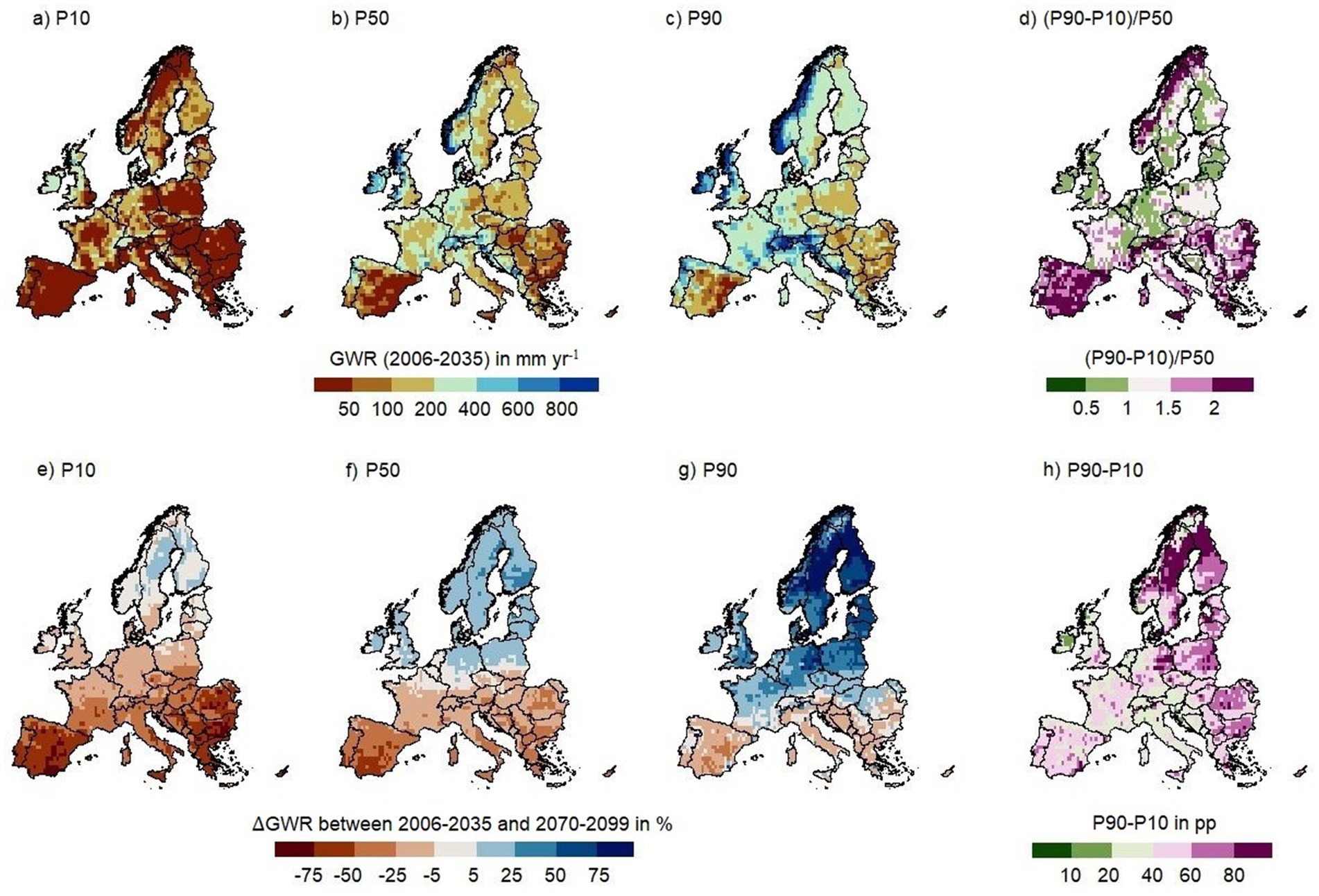
Figure 2. Long-term mean annual groundwater recharge projections computed by the MME. Parts (a) P10, (b) P50, (c) P90 show the long-term means (over eight GHMs as driven by four GCMs and the RCP2.6 and RCP8.5 scenarios) for the present period (2006–2035) in mm yr.−1, (d) shows (P90-P10)/P50, while (e) P10, (f) P50, (g) P90 show the long-term mean annual changes of groundwater recharge values among the MME until the end of the century (2070–2099, RCP8.5) compared to the present period (2006–2035), in %, and (h) shows the percent point changes between the P90 and P10.
The projected relative changes in GWR also show a wide range (Figures 2e-h), however, the models agree on a strong north–south gradient of changes, with Southern Europe tending to get drier and Northern Europe wetter. In the future (2070–2099), under the RCP8.5 scenario, the P50 of the GWR alterations among the MME members indicates a GWR increase northward of approximately 51°N and a decrease south of this (Figure 2f), while the P90 of the MME moves this boundary 5° southward (Figure 2g). However, at least 10% of the model combinations project decreases almost everywhere in Europe, with decreases of more than 90% for the Iberian peninsula and Southeastern Europe (Figure 2e). A decreasing GWR by at least 10, 25, or 50% is simulated for 47, 26 or 3% of Europe’s land area, respectively, until the end of the century (P50, RCP8.5) (Table 4). The P10 projects a decreasing GWR of more than 10, 25 or 50% for 69, 49 or 26% of Europe’s land area, respectively (RCP8.5) (Table 4). Assuming climate change mitigation (RCP2.6), the P50 shows a decrease of GWR by at least 10% on only 5% instead of 47% under RCP8.5 of Europe’s land area. Considering the P10, even with climate mitigation efforts, 69, 23 or 3% of Europe’s land area would still face a decrease in GWR exceeding 10, 25 or 50%, respectively. For the future period, the uncertainty range was computed using the difference between the P90 and P10 of the MME values (since this type of coefficient of variation is only suitable for time series with positive values). Uncertainty was found to increase from RCP2.6 to RCP8.5. For 13% of Europe’s land area, the range between the P90 and P10 among the MME was found to exceed 80 percent points (pp) for the future and RCP8.5 (Supplementary Table 2), especially in Northern Europe (Figure 2h).

Table 4. Fraction of Europe’s land area affected by a decrease in GWR of at least 10, 25, and 50% until the end of the century, according to the changes as computed by the MME, in %.
3.2 Groundwater abstractions
Currently, the sectoral abstraction patterns differ strongly across Europe, with GWNAirrig dominating in Portugal, Spain, Greece, Denmark and parts of France (Supplementary Figure 1a). In many grid cells in these regions, more than 80% of the total GWAs are used for irrigation. Domestic GWAs dominate elsewhere (Supplementary Figure 1b), while GWAs by manufacturing are relatively low overall and occur mainly in Central Europe (Supplementary Figure 1c). Absolute GWAs for manufacturing purposes are highest in industrial areas such as the Ruhr area in Germany and Northern Italy. Future projections indicate significant increases in GWNAirrig, particularly in Central and Southern parts of Europe, driven by climate change as the only driver for alterations since the irrigated area and the source of irrigation water (groundwater or surface water) were kept constant in our study (Supplementary Figure 2). Under the climate change scenario RCP8.5, the maximum abstraction values of the four GCMs show a future increase in GWNAirrig (or rather the demand for GWAs to fulfill the irrigation requirements of the crops under the changed climate) of more than 50% in France, Northern Italy and in certain regions of Germany. Across all scenarios, domestic GWAs are projected to decrease in the future in Eastern Europe, except for the UK, Switzerland and Western Germany as well as in metropolitan areas (Supplementary Figure 3). The medium scenario for future GWAs for manufacturing shows, until the end of the century, an increase in Germany (up to 25%) and Italy (up to 50%) (Supplementary Figure 4).
Considering total GWAs, especially Central and South Europe show high absolute values of GWA (Figure 3a). The high GWA scenario shows for most parts of Europe a future increase by up to 75% (Figure 3b) with the exception of Southeastern Europe, showing decreases up to 25%. The uncertainty range in the GWA scenarios is minimal for Southern Europe; this is primarily due to the dominance of groundwater irrigation on the total GWAs within those regions that exhibited only small variation among the four GCMs (Figure 3e).
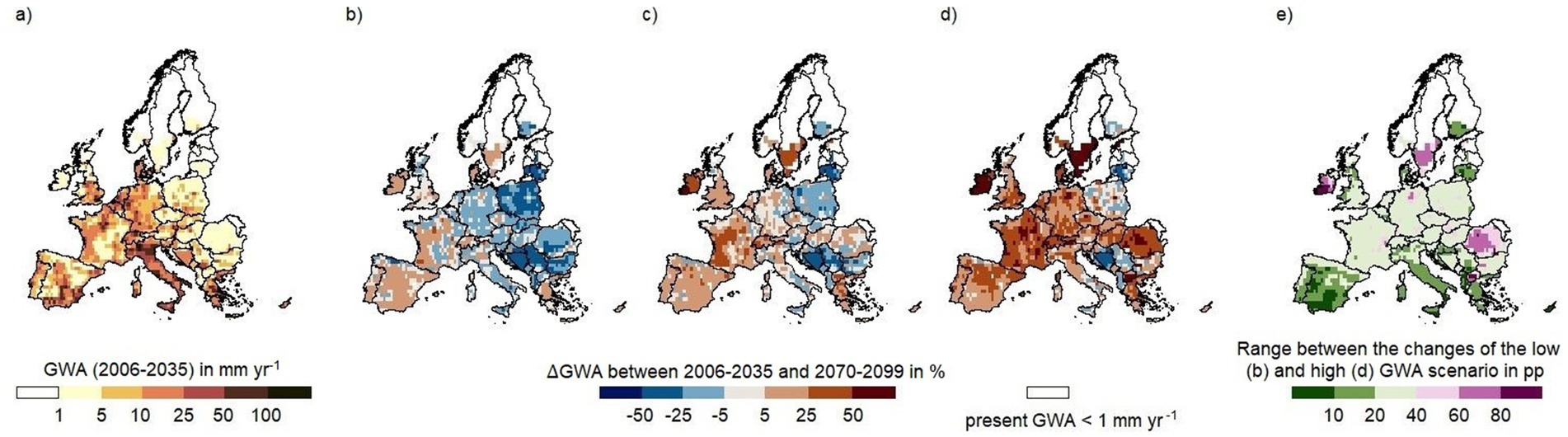
Figure 3. Simulated total groundwater abstractions among the different scenarios. Subgraph (a) shows the medium groundwater abstraction scenario for the present period, in mm yr.−1. The long-term annual relative changes of groundwater abstractions until the end of the century in comparison to the present period are shown under (b) low, (c) medium and (d) high groundwater abstraction scenarios (see Table 2 for scenario configuration), in %; (e) shows the difference between the changes of the low and high groundwater abstraction scenarios, in pp.
3.3 Groundwater discharge
In the present period, GWD is projected to be low, particularly in Southern and Eastern Europe, while regions with higher latitudes (such as the UK and the Norwegian coast) and elevated areas (e.g., the Alps) exhibit high GWD values (Figures 4a–c), similar to the GWR patterns (Figure 2). The uncertainty range of the projected GWD values by the MME follows a similar pattern of high and low GWD values; in areas characterized by low (high) GWD values, the uncertainty range between the P90 and the P10 is narrow (wide) owing to the lower (higher) absolute values of P90 and P10 in those regions (Figure 4d). The P10 of GWD in the present period shows no GWD in Southern and Southeastern Europe and only up to 200 mm yr.−1 in the rest of Europe, with the exception of the coastal regions and the Alps (Figure 4a). In these areas, GWR < GWAs, thus groundwater is depleted and the groundwater levels fall unless it is recharged from surface water bodies. Even the P90 of GWD in the present period shows no GWD in parts of Spain and Greece (Figure 4c).
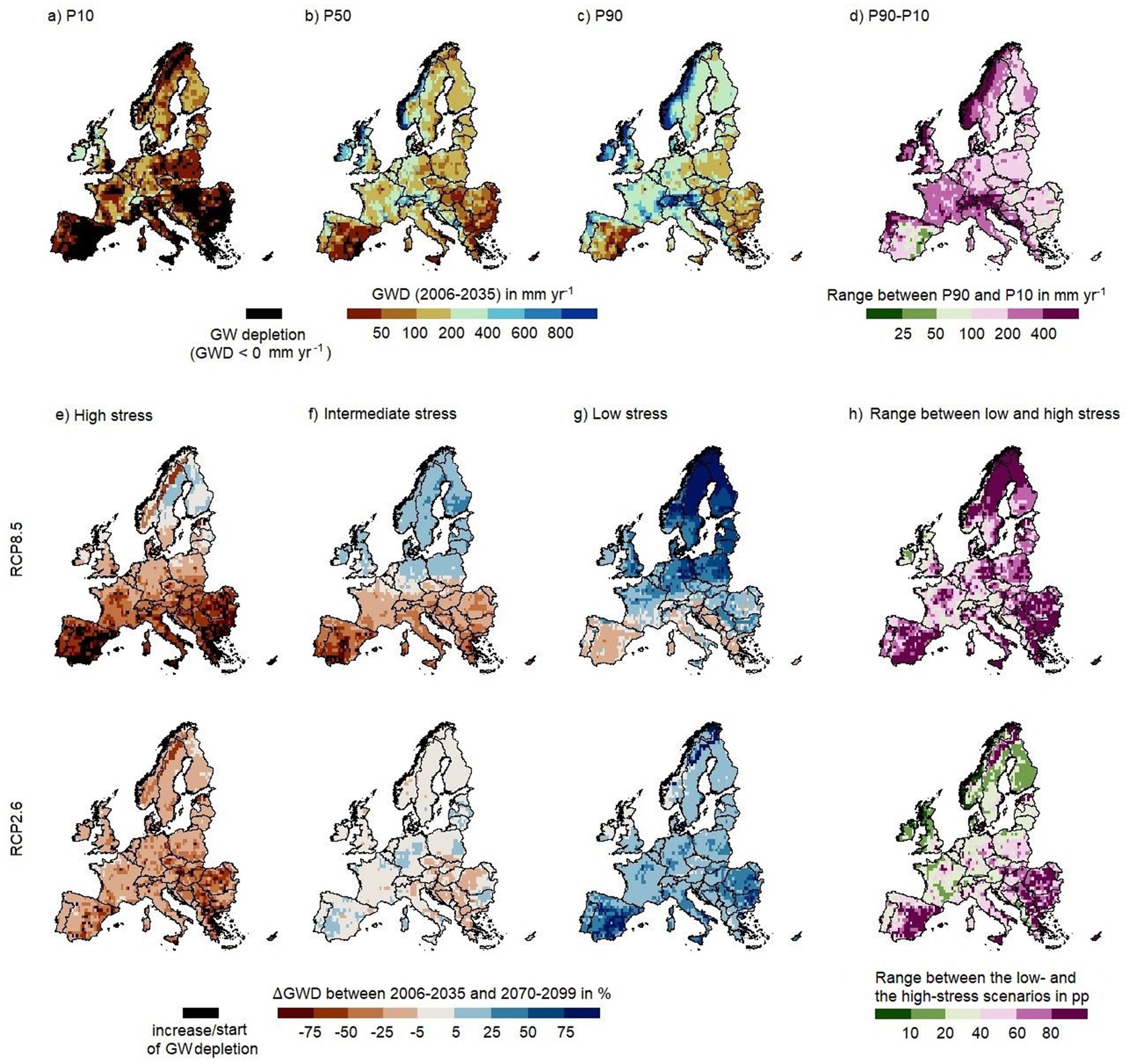
Figure 4. Simulated groundwater discharge for the different scenarios. (a) P10, (b) P50, (c) P90 of groundwater discharge in the present period, and (d) the range between P90 and P10, in mm yr.−1. (e) High-, (f) intermediate- and (g) low-stress scenarios of the mean annual relative changes of groundwater discharge between 2006–2035 and 2070–2099; first row RCP8.5, second row RCP2.6, in %. (h) Range between the low- and the high-stress scenarios, in pp (see Table 2 for scenario configuration).
Groundwater stress is defined to occur whenever GWD changes in the future. However, decreasing GWD implies greater risks to the functioning of ecosystems functioning and human water supply, i.e., the change in GWD as shown in Figures 4e–g is negative. Please note that even if the P10 of the present GWD in a cell is negative (black), it does not mean that all model combinations in this cell are negative. For the positive GCM-GHM combinations, changes in GWD can be calculated for the future; this can result in the percentiles of these changes being negative, even though the same cell is already represented as negative under P10 in the present period. Nevertheless, while the percentiles hold validity at the grid cell level, the spatial patterns, such as the increase in GWD across Europe under the low-stress and RCP2.6 scenarios (Figure 4g), do not suggest a 90% likelihood of occurrence.
In the high-stress scenario (Table 2) under RCP8.5, GWD is projected to decrease by at least 10, 25, or 50% on 75, 58, or 37% of Europe’s land area, respectively, especially in Southern, Southeastern and Central Europe (Figure 4e and Table 5). Mitigation scenario 2, which involves no net abstraction for irrigation from groundwater in the future, slightly reduces the decreasing GWD under both climate change scenarios. The intermediate-stress scenario reveals a distinct north–south gradient, indicating projected declines in GWD in Southern Europe and increases in northern regions (Figure 4f). This pattern is reflected in the affected land areas, with at least a 10, 25, or 50% decrease in GWD projected across approximately 47, 26, or 9% of Europe’s land area, respectively, under RCP8.5 (Table 5). In the intermediate scenario, significant improvements can be achieved by mitigating global warming in alignment with the Paris Agreement. Under RCP2.6, only up to 5% of Europe’s land area is projected to experience a decrease in GWD by at least 10%, depending on additional reductions in GWAs.
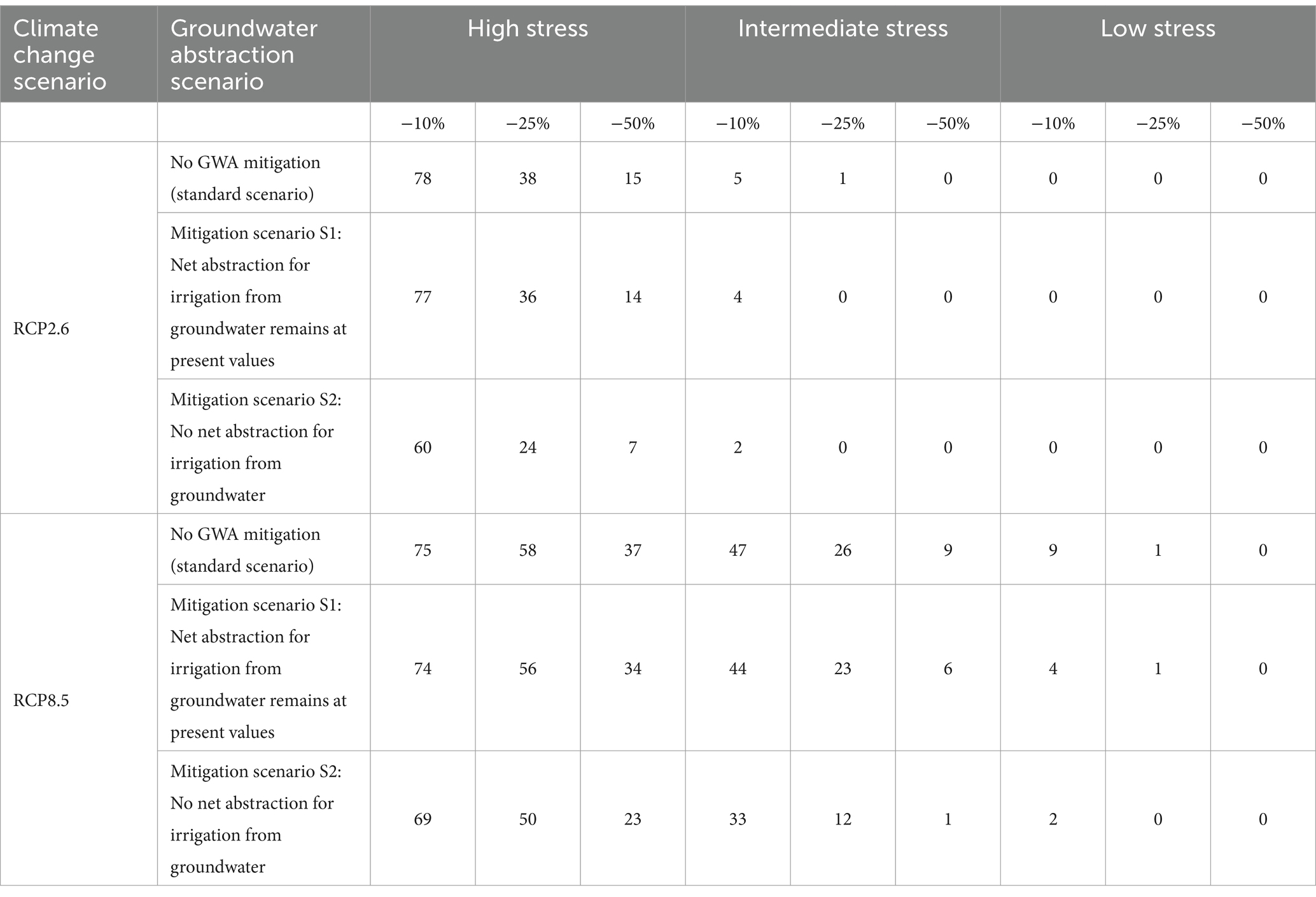
Table 5. Europe’s land areas affected by a decrease in groundwater discharge by at least 10, 25, and 50% until the end of the century (2070–2099) in the high-, intermediate- and low-stress scenarios under different GWA mitigation scenarios, in % (see Table 2 for scenario configuration).
3.4 Contribution of groundwater abstractions to decreasing groundwater discharges
The impact of GWAs on decreasing GWDs varies significantly across regions and GWA scenarios, ranging from localized effects to continent-wide implications. When focusing on the high GWA scenario, the dominance of GWAs on decreasing GWDs varies from a few regions (P10) to nearly the entire continent (P90), depending on the GWR and its changes computed by each model combination (Figure 5). In Eastern and Southeastern Europe, decreasing GWR remains the primary driver of decreasing GWD (red-colored grid cells), where decreasing GWAs fail to offset the decline in GWR caused by climate change. Considering the P90, GWAs emerge as the predominant factor driving decreasing GWD in Spain, Northern Italy, Eastern Romania and Greece. In parts of France, the UK, Germany, Poland, Austria and Slovakia (magenta-colored grid cells), even positive changes in GWR cannot counterbalance the rise in GWAs, thus resulting in declining GWDs within those areas.
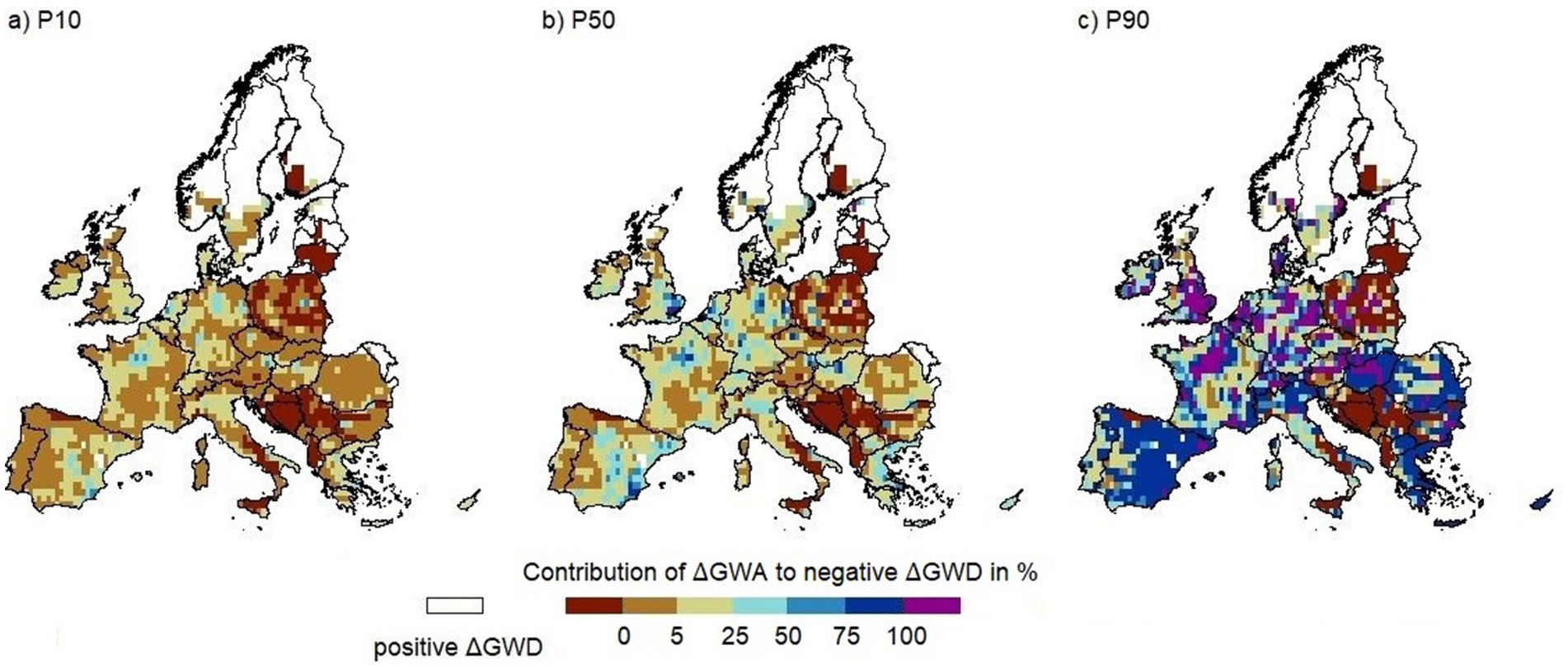
Figure 5. Simulated contribution of the total groundwater abstraction alterations to decreasing groundwater discharge until the end of the century (RCP8.5) under the high groundwater abstraction scenario (see Table 2 for scenario configuration), in %; (a) P10, (b) P50 and (c) P90. Values between 0 and 100% indicate the contribution of increasing groundwater abstractions to a decrease in groundwater discharge when groundwater recharge decreases. Red-colored grid cells (< 0%) indicate a decrease in both groundwater recharge and groundwater abstractions, but the negative alteration in groundwater recharge cannot compensate for the decrease in abstractions. Magenta-colored grid cells (> 100%) indicate an increase of both groundwater recharge and groundwater abstractions, but the increase in groundwater recharge cannot compensate for the increase in groundwater abstractions.
3.5 Mitigation scenarios
3.5.1 Scenario 1: future irrigation net abstractions remain at the present abstraction volume
Three alternative mitigation scenarios were evaluated to identify potential drivers, which may prevent decreasing GWD in the future in Europe. The GWD alterations under the mitigation scenarios can be seen in Supplementary Figure 5. S1 might reduce declining GWD by slightly elevating the GWD values compared to the standard scenario without any mitigation (Figure 6). However, in the high-stress scenario, 56% of Europe’s land area might witness a decline of GWD by at least 25% (under RCP8.5), a marginal decrease from 58% without implementing a mitigation strategy (Table 5). Nevertheless, in Southern and Eastern Europe, groundwater depletion might remain a concern when considering the high-stress scenario. S1 might solely result in a shift from negative to positive GWD values in Southern and Eastern Europe when considering the low-stress scenario (Supplementary Figure 6), although S1 might fail to induce such a shift from negative to positive GWD values when considering the high-stress or intermediate-stress scenario.
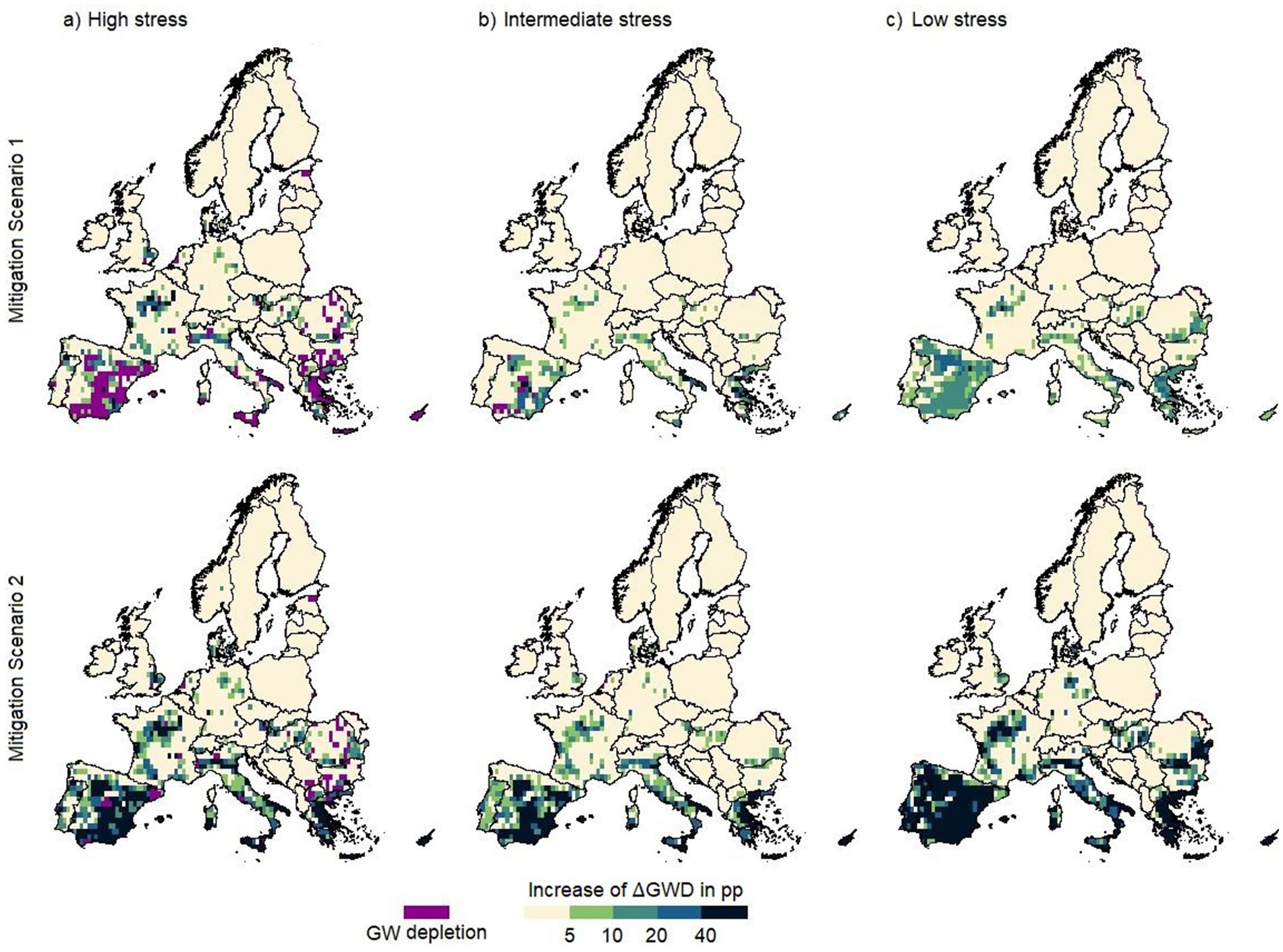
Figure 6. Projected increase of groundwater discharge caused by the mitigation scenarios S1 and S2 for the (a) high-, (b) intermediate- and (c) low stress scenarios in comparison to the standard scenario (under RCP 8.5), in pp. The dark magenta cells show an increase of groundwater discharge caused by the mitigation scenario, although groundwater depletion would still occur, even after implementing the mitigation scenarios.
3.5.2 Scenario 2: no future groundwater irrigation
The second scenario involves the complete abandonment of irrigation with groundwater, which can be considered as an extreme scenario to evaluate the sensitivity of GWD to groundwater irrigation. In the high-stress scenario, 50% of Europe’s land area would still witness a decline in GWD by at least 25% (under RCP8.5), compared to 56% under S1 (Table 5). Notably, groundwater depletion would be alleviated under S2 across most of Europe, even in the high-stress scenario (Figure 6). However, while ceasing groundwater irrigation would reduce groundwater depletion in most parts of Europe, only a few areas in Southern and Eastern Europe would witness a transition from negative to positive GWD in the high-stress scenario. In the low-stress scenario, Spain and Greece would experience such a shift (Supplementary Figure 6).
3.5.3 Scenario 3: climate change mitigation by achieving a greenhouse gas emission scenario RCP2.6 according to the Paris agreement
Mitigation of climate change might significantly reduce decreasing GWD in comparison to RCP8.5 (Table 5). Only 38% of Europe’s land areas would witness a modest decline of GWD of at least 25% vs. 58% without any mitigation in the high-stress scenario, while only a few land areas would experience declining GWD under climate change mitigation if the intermediate scenario would materialize, independently of the GWA modifications. In general, S2 (no GWNAirrig in the future) consistently shows the lowest values of affected land areas in Europe that would witness a decrease of GWD across both climate change scenarios (RCP2.6 and RCP8.5). Under S2 and RCP2.6, in particular, the least amount of land in Europe would be affected by a reduction in GWD compared to the other mitigation and climate change scenarios.
4 Discussion
4.1 Comparing groundwater stress computation approaches
This study assessed groundwater stress in Europe using a novel approach where GWD is defined in terms of the future change in GWD. The newly developed indicator allows to quantify how changing recharge and abstractions alter patterns of GWD, which is the most relevant variable for riverine, wetland and estuarine ecosystem dynamics, highlighted by Esteban et al. (2021). As already introduced, groundwater stress is usually computed by a ratio (GWSratio), dividing anthropogenic GWAs by GWR (considering return flows from irrigation) minus environmental groundwater requirements (E), exemplified by Equation 10. When the GWSratio is >1, groundwater is considered to be under stress (Gleeson et al., 2012; Wada and Heinrich, 2013).
To juxtapose the newly developed GWD approach with the existing groundwater stress computation that utilizes the ratio between abstractions and recharge, the GWSratio was computed for a medium GWA scenario in order to compare with the intermediate-stress scenario of GWD changes, following Equation (11):
where the GWSratio in the present period is computed using the median of the long-term mean GWNAirrig among the four GCMs and the present abstraction values for the domestic and manufacturing GWAs, as well as the P50 of the GWR among the ensemble. The environmental groundwater demand, E, constitutes 60% of the GWR per period, based on Link et al. (2023). The future GWSratio was additionally computed with an environmental groundwater demand, E, based on the present GWR value, to underscore the potential slow adaptation of the ecological groundwater demand to changing recharge patterns. The future quotient was computed using the medium GWA scenario (Table 2) and the P50 of the GWR values for the future under RCP8.5 among the ensemble.
Using the GWSratio reveals that groundwater stress exists in regions such as Southern Spain, Italy and Greece at the present time (Figure 7a). Projections show an increase of the GWSratio in already strained regions in the future when assuming that the future environmental groundwater demand is a constant fraction of GWR (Figure 7b). Decreasing GWSratio patterns also match the areas projecting an increase in GWD, mainly due to increasing GWR in the future (Northern Germany, Poland). However, as can be seen from the example data set in Table 6, the GWSratio may indicate a future improvement in groundwater stress, even though GWD and, thus, also groundwater storage and levels will decrease (Bosnia and Herzegovina and Serbia in Figure 7b). This occurs because the environmental groundwater requirements are determined as a fixed percentage of GWR, however, this is inappropriate since E cannot be expected to decline with declining GWR. If E were to remain at its level under the present conditions when evaluating changes in the GWSratio, which is preferable, then both the GWSratio and the change in GWD would indicate worse groundwater conditions in the future, particularly in South and Southeastern Europe (Figure 7c), with a change of +6 pp. in the case of the GWSratio and − 20% in the case of GWD, referring to a grid cell located between the border of Bosnia Herzegovina and Serbia (Table 6). Nevertheless, we would argue that a percent change in a flow such as GWD -20% in the example in Table 6, is easier to interpret than a percent point change in a ratio (+6 pp. in the example). In addition, when assuming that the ecological groundwater demand remains at the present level, the GWR in Southern Spain, Portugal, Bulgaria and Greece would not be sufficient to meet the ecological groundwater demands (Figure 7c). Although GWSratio is projected to increase slightly in the future for France, this is not anticipated to lead to significant groundwater stress in the future, given the threshold for stress initiation to be at a ratio exceeding 1, regardless of whether a constant E is assumed or not (Figures 7b,c) and despite GWD being projected to decrease by up to 25% (Figure 7d).
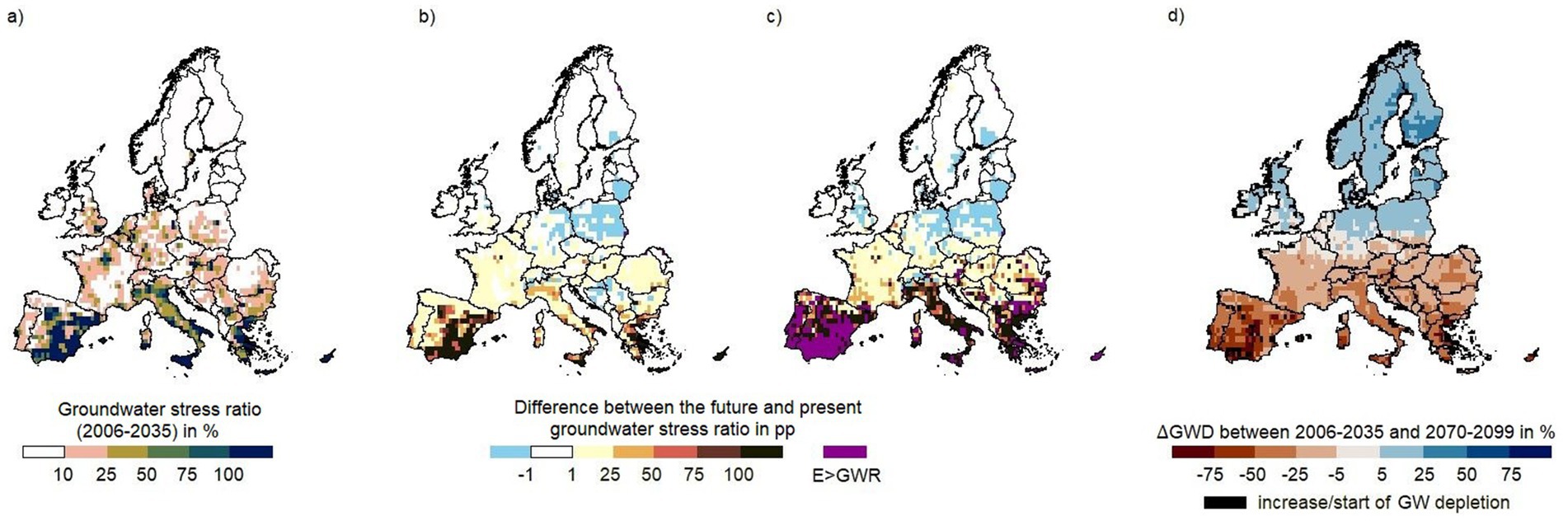
Figure 7. Simulated groundwater stress ratio ( ) in the present and future periods. (a) Present period using the medium groundwater abstraction scenario and the P50 of groundwater recharge alterations computed by the MME, in %; (b) difference between the future and present groundwater stress ratio using E based on future groundwater recharge, in pp.; (c) difference between the future and present groundwater stress ratio using E based on present groundwater recharge, in pp.; (d) groundwater discharge alteration, in % (intermediate stress under RCP8.5 from Figure 4f).
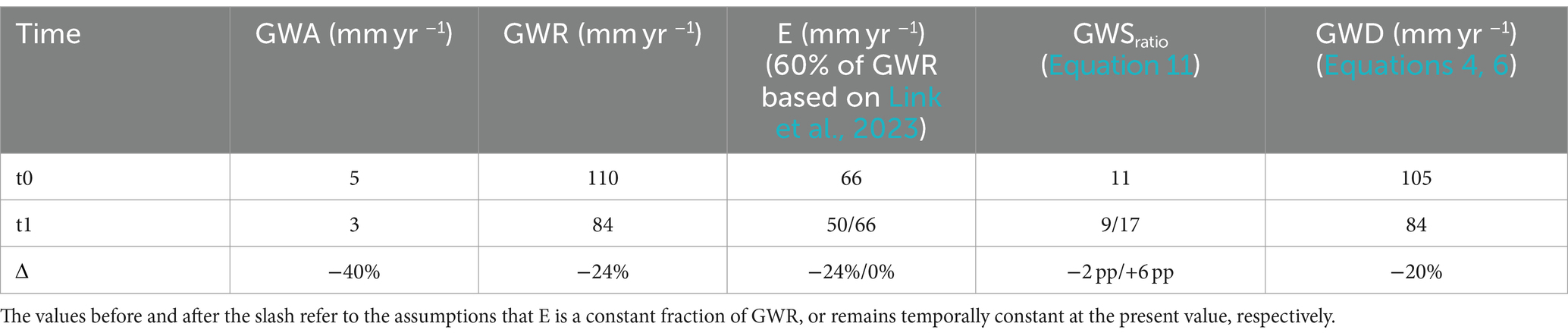
Table 6. Comparison between the different computation methodologies of groundwater stress using the rounded values of a grid cell at the border between Bosnia Herzegovina and Serbia.
4.2 Methodological limitations
The anticipation of future changes in groundwater resources and the associated demands is uncertain. To address this, we utilized a multi-model ensemble for GWR changes and scenarios for GWAs to encompass an uncertainty range. However, due to several methodological limitations, incorporating the entire uncertainty range remains challenging. Considering the inherent unpredictability of climate changes (Milly et al., 2008), future occurrences could potentially bew more severe than the current model projections (Berghuijs et al., 2024). Therefore, the uncertainties may be more pronounced because the MME in this study used only four GCMs [although the uncertainty of GWR changes is mainly caused by the GHMs rather than the GCMs (Reinecke et al., 2021)] and the results focused solely on the P10 and P90 of the MME for the GWR estimations, thus leaving values below the P10 and above the P90 unaccounted for. Moreover, some GHMs utilized in the MME do not consider active vegetation (plant transpiration responses to increasing carbon dioxide levels in the atmosphere) (van Roosmalen et al., 2009; Milly and Dunne, 2016), which could widen the uncertainty range within the MME. Therefore, enhancing the understanding of and implementing the feedback mechanisms between the hydrosphere and biosphere are crucial steps for reducing uncertainties in the GWR estimations.
Moreover, GWA data from local observations are scarcely available for the irrigation sector (Condon et al., 2021). Therefore, one GHM was employed to estimate GWNAirrig, while changes in the irrigation areas in the future were neglected due to a lack of data. The information on the irrigated areas was held constant at the 2005 levels. Consequently, the results of future alterations in GWNAirrig are attributed to climatic changes rather than changes in the extent of irrigated areas, potentially underestimating the future irrigation groundwater demand. For future domestic and manufacturing GWAs, the approach of this study made use of the official projections of population and GDP at the country and district levels. For both variables, only a single projection was used due to the lack of feasible alternatives. Hence, a business-as-usual approach was inbuilt into this data, thus neglecting potential variability in the economic prosperity, migration patterns and tourism dynamics. Moreover, the study neglected GWA for geothermal energy, which uses will likely increase in the future (Kraemer et al., 2021), and mining due to lack of data. In general, improving the monitoring and reporting of GWA for all sectors could significantly enhance the accuracy and interpretation of the modeling results and would likely provide a more comprehensive view of groundwater stress. Furthermore, groundwater use intensity is a critical variable of how water consumption is shaped in the future. Observational data for the national level was extrapolated, however, it is challenging to project the potential future alterations of water use intensities as these are highly depent on technological developments and peoples’ habits (Mannix et al., 2022). In addition, long-distance water transfers, commonly employed in various European countries (Kuhn et al., 2024), are not accounted for in this analysis. This omission could potentially result in an overestimation of GWAs in metropolitan areas that may also receive water from long-distance water transfers; these transfers were neglected in this analysis.
4.3 Groundwater management under uncertainty
The results of this study reveal that uncertainties of GWR estimations are very high, thus concurring with observations from previous studies (Reinecke et al., 2021; Gnann et al., 2023). Hence, the range of future projections of GWD alterations is highly uncertain and varies from region to region. Communicating scenarios and uncertainty ranges among projections seem a promising way to guide resilient decision-making (Piniewski et al., 2022; Deser and Phillips, 2023) since even with future research advances uncertainties in estimating the complex effects of climate change and anthropogenic developments will remain (Foley, 2010). Drawing on the approach suggested by Deser and Phillips (2023, p. 74) “using the ensemble mean for what we expect and two contrasting ensemble members for the range of what we get,” we presented the uncertainty range of GWD alterations that societies must consider for adaptation. Kause et al. (2021) and Müller and Döll (2024) recommend communicating ranges of uncertainty to enhance transparency regarding uncertain climate change information, such as changes in GWR, and to improve stakeholders’ and decision-makers’ understanding of potential climate change impacts. In this study, the range between the P10 and the P90 for recharge estimations along with the combinations of GWA scenarios were utilized to illustrate the breadth of uncertainties regarding possible alterations of GWD. On the continental scale, given the background of uncertainties presented in this article, the EU Commission formulated the precautionary principle, providing guidelines on managing uncertain risks to humans and nature when it is impossible to assign probabilities to the occurrence of such risks (Tosun, 2013):
“Although the precautionary principle is not explicitly mentioned in the Treaty except in the environmental field, its scope is far wider and covers those specific circumstances where scientific evidence is insufficient, inconclusive or uncertain and there are indications through preliminary objective scientific evaluation that there are reasonable grounds for concern that the potentially dangerous effects on the environment, human, animal or plant health may be inconsistent with the chosen level of protection” (Commission of the European Communities, 2000).
The results of this study unveiled risks to both humans and the environment stemming from changes in GWD, especially decreasing GWD under the high- and intermediate-stress scenarios. In line with the precautionary principle, policymakers are empowered to implement proactive regulatory measures to avert unnecessary harm before the risks or, in this instance, the various evaluated scenarios materialize (Tosun, 2013). In the past, the precautionary principle has been successfully applied in the EU, exemplified by the prohibition of halocarbons in the 1970s to protect the ozone layer in the atmosphere which was assumed to be being destroyed by halocarbons (Bourguignon, 2015). While the Water Framework Directive serves as a strong regulatory tool for water resources within the EU, it currently lacks provisions for adapting to future changes in (ground) water resources caused by climate change and abstractions and, thus, does not meet the required objectives so far (Zingraff-Hamed et al., 2020). The findings of this study contrast with the second evaluation of the European Water Framework Directive that indicated that only a few regions in Europe, such as the Southern UK, Southern Spain, Tenerife and Cyprus, failed to achieve the good quantitative status for groundwater bodies (European Environment Agency, 2021). Moreover, our results not only indicate high risks for groundwater resources in the future under the high-stress scenario, but also highlight significant risks for Southeastern Europe in the intermediate-stress scenario. Consequently, it can be argued that the existing level of protection may not adequately prevent harm to the beneficiaries of groundwater.
Therefore, specific management strategies to prevent groundwater stress should be tailored to the risks associated with alterations of the GWD and the underlying causes of the changes of GWD. The findings reveal that in the high-stress scenario, the complete reduction of GWAs might not alleviate decreasing GWD across most parts of Europe (Figure 8a, magenta areas); this is due to the dependence of decreasing GWD on changes in GWR. Furthermore, ceasing GWAs would not offset negative alterations in GWR, especially under RCP8.5, thus perpetuating decreasing GWD. To reduce decreasing GWD under the high-stress scenario, therefore, proactive climate change mitigation measures are essential and increasing GWAs should be strictly avoided under this scenario to reduce further stress for groundwater beneficiaries. However, the findings also indicate that under the climate change scenario RCP2.6, reducing GWAs by 25 to 75% might prevent decreasing GWD in Southern Spain and Italy, even under the high-stress scenario (Figure 8a, RCP2.6). These findings highlight the sensitivity of GWR and subsequent discharge to climate change, especially in Portugal and Southeastern Europe, because even under the low-stress scenario, a reduction in GWAs would not alleviate decreasing GWD in these regions under the worst climate change scenario (Figure 8c, RCP8.5). Ultimately, applying the precautionary principle to minimize the risks for humans and nature should involve a combination of decreasing GWNAirrig and implementing climate change mitigation measures (Table 5). For local to regional decision-making, the complexity of the results requires additional explanation and enhanced visualizations tailored to regional needs and stakeholder demands. Ideally, this should take place within a participatory process, allowing scientists and stakeholders to discuss the findings and their implications for adaptation strategies, tailored to the stakeholders’ varying levels of risk aversion or affinity (Müller and Döll, 2024; Söller et al., 2024). Furthermore, the communication should be framed to emphasize the potential for avoiding losses (what can be prevented), rather than focusing on the inevitable losses (what will happen) associated with climate change (Morton et al., 2011). When conveying the results of this study to stakeholders, the emphasis should be on highlighting that groundwater stress could be avoided under some of the mitigation scenarios simulated in this study.
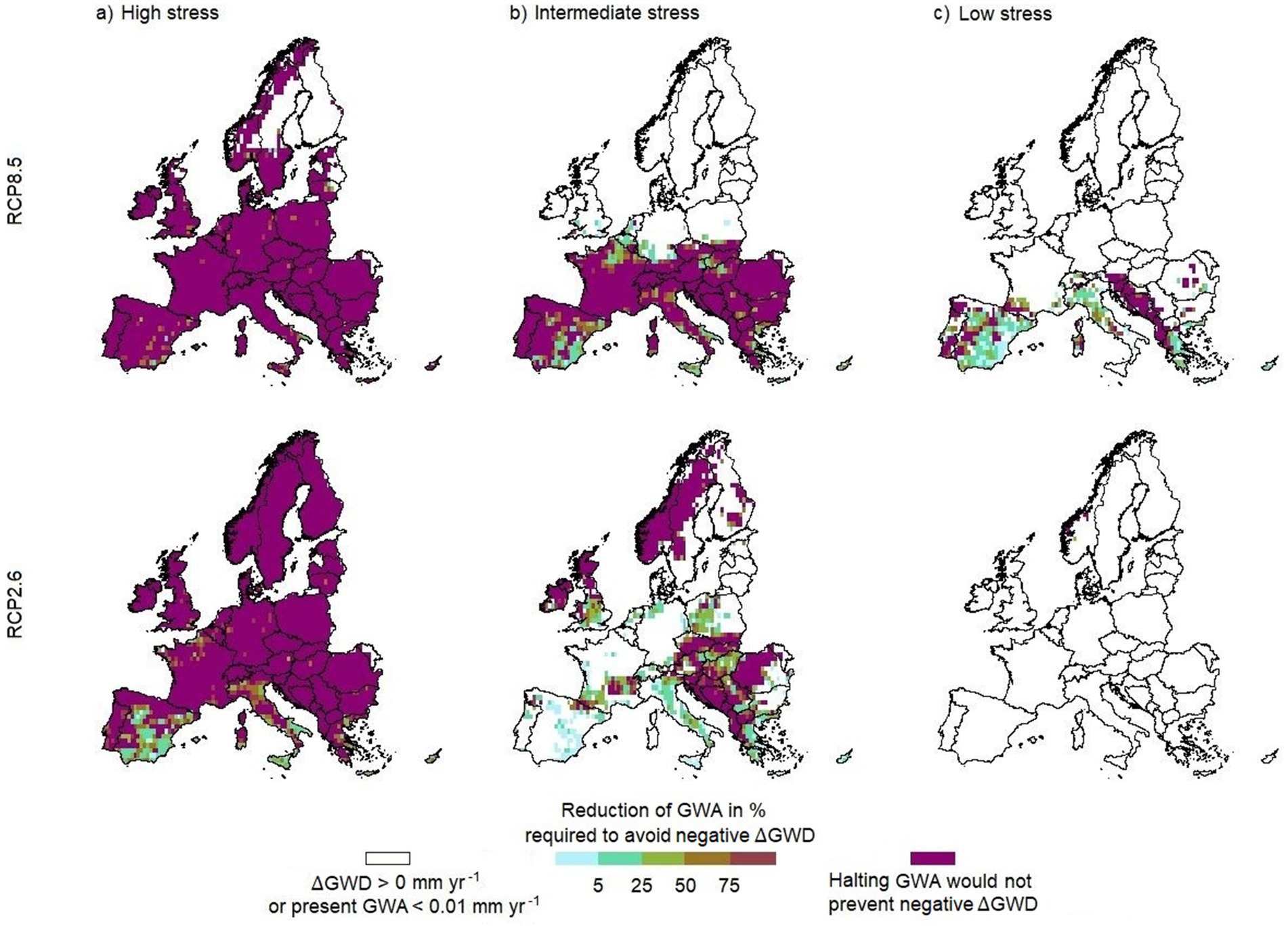
Figure 8. Simulated reduction of groundwater abstractions required to avoid decreasing groundwater discharge in the future (under RCP8.5), in %, for the (a) high-, (b) intermediate- and (c) low-stress scenarios (see Table 2 for scenario configuration).
5 Conclusion
The study evaluated the groundwater stress in Europe due to future alterations of GWD that are caused by climate change-induced GWR alterations and GWA developments, while simultaneously accounting for uncertainties using different climate change and abstraction scenarios. We propose using long-term anthropogenic change in GWD as an indicator for assessing groundwater stress since groundwater-dependent ecosystems, including surface water bodies, and also humans are adapted to a long-term equilibrium state of groundwater discharge and, thus, groundwater levels and storage. The study evaluated scenarios of groundwater stress by combining the uncertainty of future GWR and GWAs. Given that decreasing GWD causes more harm to ecosystems and the human water supply, the study analyzed how regulation scenarios (climate change mititgation and abstraction regulation) might prevent decreasing GWD. In comparison to existing groundwater stress indicators which utilize a ratio approach between abstractions and renewable groundwater resources, the newly developed GWSI reveals decreasing GWD values for Southeastern Europe. Previously unrecognized as being under stress by the ratio approach, these regions now pose a potential risk to the beneficiaries of groundwater by decreasing GWD.
However, the results reveal that the variability among hydrological models and climate change scenarios in projecting future GWR changes, and thus GWD, is very high, introducing large uncertainties into the assessment of the alteration of future groundwater resources. Uncertainties in GWD projections are only slightly higher than the GWR uncertainties; this is partly due to the restricted range of the GWA scenarios that did not encompass the alternative scenarios of irrigated areas or populations. In Europe, increases in groundwater net abstractions for irrigation are expected with the increasing severity of climate change, while decreases across most parts of Europe are expected for domestic GWAs. Manufacturing GWAs show a great variation among the future scenarios, ranging from decreases to increases across Europe, especially in Germany and Italy. In the high-stress scenario, a decrease in GWD by at least 25% is projected for approximately 58% of Europe’s land area (under RCP8.5) vs. 38% under RCP2.6. Decreasing GWD is projected, particularly for South and Southeastern Europe, under the high-stress scenario. In the case of the intermediate scenario, the mitigation of global warming might substantially reduce the affected land areas; only 1% of Europe’s land area is projected to experience a decline in GWD by at least 25% under RCP2.6 compared to 26% under RCP8.5. Reducing GWAs might improve the situation further (under both climate change scenarios), although not as significantly as climate change mitigation. Climate change-induced alterations in GWR will likely be the primary driver of decreasing GWD in Eastern and Southeastern Europe in the high GWA scenario under RCP8.5. Only in the low-stress scenario under RCP2.6, would no decline of GWD occur at all across Europe.
Management strategies should be tailored to address the dominating underlying causes of alterations of GWD, whether it be the effects of climate change on GWR or GWAs. In particular, to reduce the decreasing GWD, especially under the high-stress scenario, climate change mitigation is imperative. Under RCP2.6, reductions in GWAs, particularly for irrigation, by 25 to 75% could avoid decreasing GWD in parts of Spain and Italy where the GWAs are high, even in the high-stress scenario, while the same GWA reductions would yield similar results only in the low-stress scenario under RCP8.5. Notably, in the high-stress scenario under RCP8.5, the simulation revealed no changes of GWAs would reduce decreasing GWD. Groundwater demand management alone might not prevent the groundwater-related risks associated with climate change under the high-stress scenario, thus highlighting the critical importance of climate change mitigation and halting further increases in GWAs.
To support decision-making in tackling uncertainties arising from climate change and societal shifts, we recommend communicating a diverse range of potential scenarios in order to foster transparency regarding uncertainties and to enable decision-makers to identify strategies for reducing groundwater stress. In light of the persisting uncertainties, both in the present and future, this precautionary principle stands out as a potential tool for identifying management strategies once the potential risks have been assessed. Within the framework of the precautionary principle, addressing decreasing GWD under the high-stress scenario emerges as a proactive strategy to minimize the potential harm to humans and nature that is especially caused by decreasing GWD. Thus, climate change mitigation is considered a low-regret strategy, likely preventing further declines in GWD even under the most severe scenario. However, regional assessments require additional explanation and simplified visualization tailored to regional needs. Referring to the myriad challenges that many regions in Europe have faced, and continue to face, concerning groundwater resources and, seemingly, not adequately addressed in the European Water Framework Directive, the newly developed GWSI has the potential to enhance the understanding of the interaction between groundwater recharge and abstraction patterns on the continental scale in the future, as well as the associated risks for all beneficiaries of groundwater.
Data availability statement
The groundwater recharge datasets analyzed for this study can be found in the ISIMIP Data Repository https://data.isimip.org/. Scripts for groundwater recharge and groundwater stress data analyzing and vizualisation can be accessed on Zenodo at https://doi.org/10.5281/zenodo.11580673. Moreover, an interactive dashboard displaying groundwater abstractions and stress across Europe at the county level can be found here: https://experience.arcgis.com/experience/07325d0c698c458bbe0a1e129de837f8.
Author contributions
LS: Conceptualization, Formal analysis, Methodology, Visualization, Writing – original draft, Writing – review & editing. RL: Conceptualization, Formal analysis, Funding acquisition, Methodology, Project administration, Supervision, Writing – original draft, Writing – review & editing. HMS: Data curation, Methodology, Writing – review & editing. PD: Conceptualization, Funding acquisition, Methodology, Resources, Supervision, Writing – review & editing.
Funding
The author(s) declare that financial support was received for the research, authorship, and/or publication of this article. The Federal Ministry of Education and Research (BMBF) is funding the project “regulate -Regulation of Groundwater in Telecoupled Social-Ecological Systems” within the framework of the “Research for Sustainability” strategy (FONA) (https://www.fona.de/en/) as part of its Social-Ecological Research funding priority (grant no. 01UU2003B). Responsibility for the content of this publication lies with the authors.
Acknowledgments
We would like to thank Emmanuel Nyenah for his valuable feedback on the manuscript, as well as the Goethe Research Academy for Early Career Researchers (GRADE) proofreading service for revising the manuscript and improving its readability. Additionally, the authors acknowledge the support of ChatGPT (Version GPT-4 by OpenAI) in enhancing coding and language revision. We would also like to thank the two reviewers whose comments helped improve the readability and clarity of the research.
Conflict of interest
The authors declare that the research was conducted in the absence of any commercial or financial relationships that could be construed as a potential conflict of interest.
Publisher’s note
All claims expressed in this article are solely those of the authors and do not necessarily represent those of their affiliated organizations, or those of the publisher, the editors and the reviewers. Any product that may be evaluated in this article, or claim that may be made by its manufacturer, is not guaranteed or endorsed by the publisher.
Supplementary material
The Supplementary material for this article can be found online at: https://www.frontiersin.org/articles/10.3389/frwa.2024.1448625/full#supplementary-material
References
Ahmadi, M. S., Sušnik, J., Veerbeek, W., and Zevenbergen, C. (2020). Towards a global day zero? Assessment of current and future water supply and demand in 12 rapidly developing megacities. Sustain. Cities Soc. 61:102295. doi: 10.1016/j.scs.2020.102295
Akbar, H., Nilsalab, P., Silalertruksa, T., and Gheewala, S. H. (2022). Comprehensive review of groundwater scarcity, stress and sustainability index-based assessment. Groundw. Sustain. Dev. 18:100782. doi: 10.1016/j.gsd.2022.100782
Ashraf, B., AghaKouchak, A., Alizadeh, A., Mousavi Baygi, M., Moftakhari, R., Mirchi, A., et al. (2017). Quantifying anthropogenic stress on groundwater resources. Sci. Rep. 7:12910. doi: 10.1038/s41598-017-12877-4
Barlow, P. M., and Leake, S. A. (2012). Streamflow depletion by Wells—understanding and managing the effects of groundwater pumping on streamflow. Available at: https://pubs.usgs.gov/circ/1376/pdf/circ1376_barlow_report_508.pdf (Accessed September 30, 2024).
Becher, J., Englisch, C., Griebler, C., and Bayer, P. (2022). Groundwater fauna downtown - drivers, impacts and implications for subsurface ecosystems in urban areas. J. Contam. Hydrol. 248:104021. doi: 10.1016/j.jconhyd.2022.104021
Berghuijs, W. R., Collenteur, R. A., Jasechko, S., Jaramillo, F., Luijendijk, E., Moeck, C., et al. (2024). Groundwater recharge is sensitive to changing long-term aridity. Nat. Clim. Chang. 14, 357–363. doi: 10.1038/s41558-024-01953-z
Bourguignon, D. (2015). The precautionary principle: Definitions, applications and governance. Available at: https://www.europarl.europa.eu/RegData/etudes/IDAN/2015/573876/EPRS_IDA(2015)573876_EN.pdf (Accessed September 30, 2024).
Commission of the European Communities (2000). Communication from the Commission on the precautionary principle. Available at: https://eur-lex.europa.eu/LexUriServ/LexUriServ.do?uri=COM:2000:0001:FIN:en:PDF (Accessed September 30, 2024).
Condon, L. E., Kollet, S., Bierkens, M. F. P., Fogg, G. E., Maxwell, R. M., Hill, M. C., et al. (2021). Global groundwater modeling and monitoring: opportunities and challenges. Water Resour. Res. 57:e2020WR029500. doi: 10.1029/2020WR029500
Crameri, F., Shephard, G. E., and Heron, P. J. (2020). The misuse of colour in science communication. Nat. Commun. 11:5444. doi: 10.1038/s41467-020-19160-7
Crosbie, R. S., Pickett, T., Mpelasoka, F. S., Hodgson, G., Charles, S. P., and Barron, O. V. (2013). An assessment of the climate change impacts on groundwater recharge at a continental scale using a probabilistic approach with an ensemble of GCMs. Clim. Chang. 117, 41–53. doi: 10.1007/s10584-012-0558-6
de Graaf, I. E. M., Gleeson, T., van Beek, L. P. H., Sutanudjaja, E. H., and Bierkens, M. F. P. (2019). Environmental flow limits to global groundwater pumping. Nature 574, 90–94. doi: 10.1038/s41586-019-1594-4
Deser, C., and Phillips, A. S. (2023). A range of outcomes: the combined effects of internal variability and anthropogenic forcing on regional climate trends over Europe. Nonlin. Processes Geophys. 30, 63–84. doi: 10.5194/npg-30-63-2023
Döll, P. (2009). Vulnerability to the impact of climate change on renewable groundwater resources: a global-scale assessment. Environ. Res. Lett. 4:35006. doi: 10.1088/1748-9326/4/3/035006
Döll, P., Jiménez-Cisneros, B., Oki, T., Arnell, N. W., Benito, G., Cogley, J. G., et al. (2015). Integrating risks of climate change into water management. Hydrol. Sci. J. 60, 4–13. doi: 10.1080/02626667.2014.967250
Döll, P., Müller Schmied, H., Schuh, C., Portmann, F. T., and Eicker, A. (2014). Global-scale assessment of groundwater depletion and related groundwater abstractions: combining hydrological modeling with information from well observations and GRACE satellites. Water Resour. Res. 50, 5698–5720. doi: 10.1002/2014WR015595
Donheiser, M., Huth, K., Joeres, A., Wörpel, S., and Steeger, G. (2022). Grundwasser-Atlas: Wo in Deutschland die Wasserspiegel sinken. Available at: https://correctiv.org/aktuelles/kampf-um-wasser/2022/10/25/klimawandel-grundwasser-in-deutschland-sinkt/?bbox=-0.8746202480758143%2C47.14736893159511%2C22.174620248078725%2C56.39754870297196&zoom=4.790502223347596#tool (Accessed September 30, 2024).
Earman, S., and Dettinger, M. (2011). Potential impacts of climate change on groundwater resources – a global review. J. Water Clim. Change 2, 213–229. doi: 10.2166/wcc.2011.034
Esteban, E., Calvo, E., and Albiac, J. (2021). Ecosystem shifts: implications for groundwater management. Environ. Resour. Econ. 79, 483–510. doi: 10.1007/s10640-021-00569-7
European Environment Agency (2021). Groundwater bodies: Proportion failing to achieve good status, by RBD: Water bodies (excluding unknown status) failing to achieve Good Quantitative status, by RBD. Available at: https://water.europa.eu/freshwater/resources/metadata/dashboards/quantitative-status/groundwater-bodies-proportion-failing-to-achieve-good-status-by-rbd-map (Accessed September 30, 2024).
European Environment Agency (2022). Europe’s groundwater - a key resource under pressure. Available at: https://www.eea.europa.eu/publications/europes-groundwater/europes-groundwater/download.pdf.static (Accessed September 30, 2024).
EUROSTAT (2023a). Annual freshwater abstraction by source and sector (env_wat_abs). Available at: https://ec.europa.eu/eurostat/databrowser/view/env_wat_abs/default/table?lang=en (Accessed September 30, 2024).
EUROSTAT (2023b). Population on 1st January by age, sex, type of projection and NUTS 3 region (proj_19rp3). Available at: https://ec.europa.eu/eurostat/databrowser/view/proj_19rp3/default/table?lang=en (Accessed September 30, 2024).
Fabbri, P., Piccinini, L., Marcolongo, E., Pola, M., Conchetto, E., and Zangheri, P. (2016). Does a change of irrigation technique impact on groundwater resources? A case study in northeastern Italy. Environ. Sci. Pol. 63, 63–75. doi: 10.1016/j.envsci.2016.05.009
Fallah, A., Sungmin, O., and Orth, R. (2020). Climate-dependent propagation of precipitation uncertainty into the water cycle. Hydrol. Earth Syst. Sci. 24, 3725–3735. doi: 10.5194/hess-24-3725-2020
Federal Statistical Office of Germany (2023). Statistical Report - Environmental Economic Accounts - Water Accounts - Reporting Period 2001–2019. Available at: https://www.destatis.de/DE/Themen/Gesellschaft-Umwelt/Umwelt/UGR/rohstoffe-materialfluesse-wasser/Publikationen/Downloads/statistischer-bericht-ugr-wassergesamtrechnung-5851401199005.html (Accessed September 30, 2024).
Federal Statistical Office of Germany (2024). Umweltökonomische Gesamtrechnungen im Überblick 2024. Available at: https://www.destatis.de/DE/Themen/Gesellschaft-Umwelt/Umwelt/UGR/ueberblick/Publikationen/Downloads/statistischer-bericht-ugr-ueberblick-5850026249005.html (Accessed September 30, 2024).
Flörke, M., Kynast, E., Bärlund, I., Eisner, S., Wimmer, F., and Alcamo, J. (2013). Domestic and industrial water uses of the past 60 years as a mirror of socio-economic development: a global simulation study. Glob. Environ. Chang. 23, 144–156. doi: 10.1016/j.gloenvcha.2012.10.018
Foley, A. M. (2010). Uncertainty in regional climate modelling: a review. Prog. Phys. Geography Earth Environ. 34, 647–670. doi: 10.1177/0309133310375654
Frieler, K., Lange, S., Piontek, F., Reyer, C. P. O., Schewe, J., Warszawski, L., et al. (2017). Assessing the impacts of 1.5 °C global warming – simulation protocol of the inter-sectoral impact model Intercomparison project (ISIMIP2b). Geosci. Model Dev. 10, 4321–4345. doi: 10.5194/gmd-10-4321-2017
Gelati, E., Zajac, Z., Ceglar, A., Bassu, S., Bisselink, B., Adamovic, M., et al. (2020). Assessing groundwater irrigation sustainability in the Euro-Mediterranean region with an integrated agro-hydrologic model. Adv. Sci. Res. 17, 227–253. doi: 10.5194/asr-17-227-2020
Gleeson, T., and Wada, Y. (2013). Assessing regional groundwater stress for nations using multiple data sources with the groundwater footprint. Environ. Res. Lett. 8:4010. doi: 10.1088/1748-9326/8/4/044010
Gleeson, T., Wada, Y., Bierkens, M. F. P., and van Beek, L. P. H. (2012). Water balance of global aquifers revealed by groundwater footprint. Nature 488, 197–200. doi: 10.1038/nature11295
Gnann, S., Reinecke, R., Stein, L., Wada, Y., Thiery, W., Müller Schmied, H., et al. (2023). Functional relationships reveal differences in the water cycle representation of global water models. Nat. Water 1, 1079–1090. doi: 10.1038/s44221-023-00160-y
Gosling, S. N., Müller Schmied, H., Burek, P., Chang, J., Ciais, P., Döll, P., et al. (2023). ISIMIP2b simulation data from the global water sector. ISIMIP Rep. 2023:626689. doi: 10.48364/ISIMIP.626689
Griebler, C., and Avramov, M. (2015). Groundwater ecosystem services: a review. Freshwater Sci. 34, 355–367. doi: 10.1086/679903
Griebler, C., Malard, F., and Lefébure, T. (2014). Current developments in groundwater ecology - from biodiversity to ecosystem function and services. Curr. Opin. Biotechnol. 27, 159–167. doi: 10.1016/j.copbio.2014.01.018
Hahn, H. J., Schweer, C., and Griebler, C. (2018). Grundwasserökosysteme im Recht? Grundwasser 23, 209–218. doi: 10.1007/s00767-018-0394-3
Hänsel, S., Ustrnul, Z., Łupikasza, E., and Skalak, P. (2019). Assessing seasonal drought variations and trends over Central Europe. Adv. Water Resour. 127, 53–75. doi: 10.1016/j.advwatres.2019.03.005
Herbert, C., and Döll, P. (2019). Global assessment of current and future groundwater stress with a focus on transboundary aquifers. Water Resour. Res. 55, 4760–4784. doi: 10.1029/2018WR023321
Hughes, A., Mansour, M., Ward, R., Kieboom, N., Allen, S., Seccombe, D., et al. (2021). The impact of climate change on groundwater recharge: national-scale assessment for the British mainland. J. Hydrol. 598:126336. doi: 10.1016/j.jhydrol.2021.126336
Ionita, M., Nagavciuc, V., Kumar, R., and Rakovec, O. (2020). On the curious case of the recent decade, mid-spring precipitation deficit in Central Europe. NPJ Clim. Atmos Sci. 3:812. doi: 10.1038/s41612-020-00153-8
Kause, A., Bruine de Bruin, W., Domingos, S., Mittal, N., Lowe, J., and Fung, F. (2021). Communications about uncertainty in scientific climate-related findings: a qualitative systematic review. Environ. Res. Lett. 16:53005. doi: 10.1088/1748-9326/abb265
Kløve, B., Ala-aho, P., Bertrand, G., Boukalova, Z., Ertürk, A., Goldscheider, N., et al. (2011). Groundwater dependent ecosystems. part I: Hydroecological status and trends. Environ. Sci. Pol. 14, 770–781. doi: 10.1016/j.envsci.2011.04.002
Koch, F., Menberg, K., Schweikert, S., Spengler, C., Hahn, H. J., and Blum, P. (2021). Groundwater fauna in an urban area – natural or affected? Hydrol. Earth Syst. Sci. 25, 3053–3070. doi: 10.5194/hess-25-3053-2021
Kraemer, D. K., Ball, D. M., Re, V., Simmons, C. T., Bothwell, T., Verweij, H. J., et al. (2021). “The future of groundwater science and research” in Global groundwater: Source, scarcity, sustainability, security, and solutions. eds. A. Mukherjee, B. R. Scanlon, A. Aureli, S. Langan, H. Guo, and A. A. Mckenzie (Cambridge, MA: Elsevier), 503–518.
Kuhn, D., Luetkemeier, R., Frick-Trzebitzky, F., Söller, L., and Fehrs, K. (2024). Infrastructural lock-ins in the temporal and spatial development of a long-distance water transfer in Germany. J. Hydrol. 634:131070. doi: 10.1016/j.jhydrol.2024.131070
Link, A., El-Hokayem, L., Usman, M., Conrad, C., Reinecke, R., Berger, M., et al. (2023). Groundwater-dependent ecosystems at risk – global hotspot analysis and implications. Environ. Res. Lett. 18:acea97. doi: 10.1088/1748-9326/acea97
Lüdtke, D. U., Luetkemeier, R., Schneemann, M., and Liehr, S. (2021). Increase in daily household water demand during the first wave of the COVID-19 pandemic in Germany. Water 13:260. doi: 10.3390/w13030260
Luetkemeier, R., Söller, L., and Frick-Trzebitzky, F. (2022). “Anthropogenic pressures on groundwater” in Encyclopedia of Inland Waters. ed. T. Mehner (Cambridge, MA: Elsevier), 548–559.
Mannix, D. H., Birkenholtz, T. L., Abrams, D. B., and Cullen, C. (2022). Uncertain waters: participatory groundwater modelling in Chicago’s suburbs. Geoforum 132, 182–194. doi: 10.1016/j.geoforum.2021.09.006
Mansour, M. M., and Hughes, A. G. (2017). Summary of results for national scale recharge modelling under conditions of predicted climate change. Available at: https://nora.nerc.ac.uk/id/eprint/521605/1/OR17026.pdf (Accessed September 30, 2024).
Martinsen, G., Bessiere, H., Caballero, Y., Koch, J., Collados-Lara, A. J., Mansour, M., et al. (2022). Developing a pan-European high-resolution groundwater recharge map - combining satellite data and national survey data using machine learning. Sci. Total Environ. 822:153464. doi: 10.1016/j.scitotenv.2022.153464
Marx, A., Boeing, F., and Samaniego, L. (2022). Zur Entwicklung des Wasserdargebotes im Kontext des Klimawandels: Ergebnisse des Forschungsprojekts “UFZ-Klimafolgenstudie” für das DVGW Zukunftsprogramm Wasser. Bonn: Energie Wasser-Praxis.
Milly, P. C. D., Betancourt, J., Falkenmark, M., Hirsch, R. M., Kundzewicz, Z. W., Lettenmaier, D. P., et al. (2008). Climate change. Stationarity is dead: whither water management? Science 319, 573–574. doi: 10.1126/science.1151915
Milly, P. C. D., and Dunne, K. A. (2016). Potential evapotranspiration and continental drying. Nat. Clim. Change 6, 946–949. doi: 10.1038/nclimate3046
Morton, T. A., Rabinovich, A., Marshall, D., and Bretschneider, P. (2011). The future that may (or may not) come: how framing changes responses to uncertainty in climate change communications. Glob. Environ. Change 21, 103–109. doi: 10.1016/j.gloenvcha.2010.09.013
Müller, L., and Döll, P. (2024). Quantifying and communicating uncertain climate change hazards in participatory climate change adaptation processes. Geosci. Commun. 7, 121–144. doi: 10.5194/gc-7-121-2024
Müller Schmied, H., Cáceres, D., Eisner, S., Flörke, M., Herbert, C., Niemann, C., et al. (2021). The global water resources and use model WaterGAP v2.2d: model description and evaluation. Geosci. Model Dev. 14, 1037–1079. doi: 10.5194/gmd-14-1037-2021
Müller Schmied, H., Flörke, M., and Döll, P. (2022). “Global water use” in The Palgrave handbook of global sustainability. ed. R. Brinkmann (Cham: Palgrave Macmillan), 1–15.
NISRA (2020). 2018-based Population Projections for Northern Ireland. Available at: https://www.nisra.gov.uk/publications/2018-based-population-projections-northern-ireland (Accessed September 30, 2024).
NRS (2020). Population Projections for Scottish Areas (2018-based). Available at: https://www.nrscotland.gov.uk/statistics-and-data/statistics/statistics-by-theme/population/population-projections/sub-national-population-projections/2018-based (Accessed September 30, 2024).
OECD (2017). Real GDP long-term forecast. Available at: https://data.oecd.org/gdp/real-gdp-long-term-forecast.htm (Accessed September 30, 2024).
ONS (2020). Projections of the future size and age structure of the population of the UK and its constituent countries. Based on mid-year population estimates and assumptions of future fertility, mortality and migration. Available at: https://www.ons.gov.uk/peoplepopulationandcommunity/populationandmigration/populationprojections/bulletins/subnationalpopulationprojectionsforengland/2018based (Accessed September 30, 2024).
Pedersen, T. L., and Crameri, F. (2023). Colour palettes based on the scientific colour-maps: R package scico [version 1.5.0]. Available at: https://cran.r-project.org/package=scico (Accessed September 30, 2024).
Peiris, T. A., and Döll, P. (2023). Improving the quantification of climate change hazards by hydrological models: a simple ensemble approach for considering the uncertain effect of vegetation response to climate change on potential evapotranspiration. Hydrol. Earth Syst. Sci. 27, 3663–3686. doi: 10.5194/hess-27-3663-2023
Piniewski, M., Eini, M. R., Chattopadhyay, S., Okruszko, T., and Kundzewicz, Z. W. (2022). Is there a coherence in observed and projected changes in riverine low flow indices across Central Europe? Earth Sci. Rev. 233:104187. doi: 10.1016/j.earscirev.2022.104187
Poff, L. N., Allan, D. J., Bain, M. B., Karr, J. R., Prestegaard, K. L., Richter, B. D., et al. (1997). The natural flow regime: a paradigm for river conservation and restoration. Bioscience 47, 769–784. doi: 10.2307/1313099
Pop, M. M., Di Lorenzo, T., and Iepure, S. (2023). Living on the edge – an overview of invertebrates from groundwater habitats prone to extreme environmental conditions. Front. Ecol. Evol. 10:1054841. doi: 10.3389/fevo.2022.1054841
R Core Team (2023). R: A Language and Environment for Statistical Computing. Available at: https://www.R-project.org/ (Accessed September 30, 2024).
Reinecke, R., Müller Schmied, H., Trautmann, T., Andersen, L. S., Burek, P., Flörke, M., et al. (2021). Uncertainty of simulated groundwater recharge at different global warming levels: a global-scale multi-model ensemble study. Hydrol. Earth Syst. Sci. 25, 787–810. doi: 10.5194/hess-25-787-2021
Research Institute for Agriculture and Fisheries Mecklenburg-Western Pomerania (2024). Mitteilungen der LFA, Heft 65: Der Baltische Stör. Available at: https://www.landwirtschaft-mv.de/static/LFA/Dateien/Hefte/MdLFA_Heft65.pdf (Accessed September 30, 2024).
Richey, A. S., Thomas, B. F., Lo, M.-H., Reager, J. T., Famiglietti, J. S., Voss, K., et al. (2015). Quantifying renewable groundwater stress with GRACE. Water Resour. Res. 51, 5217–5238. doi: 10.1002/2015WR017349
Riedel, T., and Weber, T. K. D. (2020). Review: the influence of global change on Europe’s water cycle and groundwater recharge. Hydrogeol. J. 28, 1939–1959. doi: 10.1007/s10040-020-02165-3
Riedel, T., Weber, T. K. D., and Bergmann, A. (2023). Near constant groundwater recharge efficiency under global change in a central European catchment. Hydrol. Process. 37:e14805. doi: 10.1002/hyp.14805
Ruppaner, M. (2021). “Weniger Wasser – mehr Bedarf: Ausbleibende Niederschläge, höhere Verdunstung und sinkende Grundwasserstände werden ein immer größeres gesellschaftliches Konfliktfeld” in Der kritische Agrarbericht 2021: Welt im Fieber - Klima & Wandel. ed. E. V. Agrarbündnis (Rockville, MD: ABL Verlag), 218–222.
Saccò, M., Mammola, S., Altermatt, F., Alther, R., Bolpagni, R., Brancelj, A., et al. (2024). Groundwater is a hidden global keystone ecosystem. Glob. Chang. Biol. 30:e17066. doi: 10.1111/gcb.17066
Scanlon, B. R., Fakhreddine, S., Rateb, A., Graaf, I., Famiglietti, J., Gleeson, T., et al. (2023). Global water resources and the role of groundwater in a resilient water future. Nat. Rev. Earth Environ. 4, 87–101. doi: 10.1038/s43017-022-00378-6
Schwingshackl, C., Davin, E. L., Hirschi, M., Sørland, S. L., Wartenburger, R., and Seneviratne, S. I. (2019). Regional climate model projections underestimate future warming due to missing plant physiological CO2 response. Environ. Res. Lett. 14:114019. doi: 10.1088/1748-9326/ab4949
Siebert, S., Burke, J., Faures, J. M., Frenken, K., Hoogeveen, J., Döll, P., et al. (2010). Groundwater use for irrigation – a global inventory. Hydrol. Earth Syst. Sci. 14, 1863–1880. doi: 10.5194/hess-14-1863-2010
Siebert, S., Henrich, V., Frenken, K., and Burke, J. (2013). Update of the digital global map of irrigation areas to version 5. Rome: Institute of Crop Science and Resource Conservation, University of Bonn, Germany; land and water division, food and agriculture Organization of the United Nations.
Siebert, S., Kummu, M., Porkka, M., Döll, P., Ramankutty, N., and Scanlon, B. R. (2015). A global data set of the extent of irrigated land from 1900 to 2005. Hydrol. Earth Syst. Sci. 19, 1521–1545. doi: 10.5194/hess-19-1521-2015
Skiadaresis, G., Schwarz, J., Stahl, K., and Bauhus, J. (2021). Groundwater extraction reduces tree vitality, growth and xylem hydraulic capacity in Quercus robur during and after drought events. Sci. Rep. 11:5149. doi: 10.1038/s41598-021-84322-6
Smerdon, B. D., and Drewes, J. E. (2017). Groundwater recharge: the intersection between humanity and hydrogeology. J. Hydrol. 555, 909–911. doi: 10.1016/j.jhydrol.2017.10.075
Söller, L., Hodžić, D., and Luetkemeier, R. (2024). Water futures on Krk Island - guiding principles for achieving a sustainable water-tourism-Nexus (available in English and Croatian). Available at: https://zenodo.org/records/10907296 (Accessed September 30, 2024).
Stigter, T. Y., Miller, J., Chen, J., and Re, V. (2023). Groundwater and climate change: threats and opportunities. Hydrogeol. J. 31, 7–10. doi: 10.1007/s10040-022-02554-w
Tabarmayeh, M., Zarei, M., and Batelaan, O. (2022). A new approach to quantification of groundwater resource stress. J. Hydrol. Reg. Stu. 42:101161. doi: 10.1016/j.ejrh.2022.101161
Taylor, C. J., and Alley, W. M. (2001). Ground-water-level monitoring and the importance of long-term water-level Dataent. Denver, CO: US Geological Survey.
Taylor, R. G., Scanlon, B., Döll, P., Rodell, M., van Beek, R., Wada, Y., et al. (2013). Ground water and climate change. Nat. Clim. Change 3, 322–329. doi: 10.1038/nclimate1744
Telteu, C.-E., Müller Schmied, H., Thiery, W., Leng, G., Burek, P., Liu, X., et al. (2021). Understanding each other's models: an introduction and a standard representation of 16 global water models to support intercomparison, improvement, and communication. Geosci. Model Dev. 14, 3843–3878. doi: 10.5194/gmd-14-3843-2021
Thissen, W., Kwakkel, J., Mens, M., van der Sluijs, J., Stemberger, S., Wardekker, A., et al. (2017). Dealing with uncertainties in fresh water supply: experiences in the Netherlands. Water Resour. Manag. 31, 703–725. doi: 10.1007/s11269-015-1198-1
Tosun, J. (2013). How the EU handles uncertain risks: understanding the role of the precautionary principle. J. Eur. Publ. Policy 20, 1517–1528. doi: 10.1080/13501763.2013.834549
van Roosmalen, L., Sonnenborg, T. O., and Jensen, K. H. (2009). Impact of climate and land use change on the hydrology of a large-scale agricultural catchment. Water Resour. Res. 45:6760. doi: 10.1029/2007WR006760
Vandecasteele, I., Bianchi, A., Batista e Silva, F., Lavalle, C., and Batelaan, O. (2014). Mapping current and future European public water withdrawals and consumption. Hydrol. Earth Syst. Sci. 18, 407–416. doi: 10.5194/hess-18-407-2014
Vergnes, J.-P., Caballero, Y., and Lanini, S. (2023). Assessing climate change impact on French groundwater resources using a spatially distributed hydrogeological model. Hydrol. Sci. J. 68, 209–227. doi: 10.1080/02626667.2022.2150553
Verkaik, J., Sutanudjaja, E. H., Essink, O., Gualbert, H. P., Lin, H. X., and Bierkens, M. F. P. (2024). GLOBGM v1.0: a parallel implementation of a 30 arcsec PCR-GLOBWB-MODFLOW global-scale groundwater model. Geosci. Model Dev. 17, 275–300. doi: 10.5194/gmd-17-275-2024
Wada, Y., and Heinrich, L. (2013). Assessment of transboundary aquifers of the world—vulnerability arising from human water use. Environ. Res. Lett. 8:24003. doi: 10.1088/1748-9326/8/2/024003
Wan, W., Döll, P., and Müller Schmied, H. (2024). Global-scale groundwater recharge modeling is improved by tuning against ground-based estimates for karst and non-karst areas. Water Resour. Res. 60:e2023WR036182. doi: 10.1029/2023WR036182
Welsh Government (2020). Subnational population projections (local authority) additional variant projections: 2018-based. Available at: https://www.gov.wales/subnational-population-projections-local-authority-additional-variant-projections-2018-based (Accessed September 30, 2024).
Wunsch, A., Liesch, T., and Broda, S. (2022). Deep learning shows declining groundwater levels in Germany until 2100 due to climate change. Nat. Commun. 13:1221. doi: 10.1038/s41467-022-28770-2
Xanke, J., and Liesch, T. (2022). Quantification and possible causes of declining groundwater resources in the Euro-Mediterranean region from 2003 to 2020. Hydrogeol. J. 30, 379–400. doi: 10.1007/s10040-021-02448-3
Zheng, Y., Yang, H., Huang, J., Wang, L., and Lv, A. (2021). The impacts of the geographic distribution of manufacturing plants on groundwater withdrawal in China. Water 13:1158. doi: 10.3390/w13091158
Keywords: climate change, Europe, groundwater abstractions, groundwater recharge, groundwater discharge, groundwater stress, uncertainty
Citation: Söller L, Luetkemeier R, Müller Schmied H and Döll P (2024) Groundwater stress in Europe—assessing uncertainties in future groundwater discharge alterations due to water abstractions and climate change. Front. Water. 6:1448625. doi: 10.3389/frwa.2024.1448625
Edited by:
Dioni Pérez-Blanco, University of Salamanca, SpainReviewed by:
Francesca Pianosi, University of Bristol, United KingdomLaura Gil, University of Salamanca, Spain
Copyright © 2024 Söller, Luetkemeier, Müller Schmied and Döll. This is an open-access article distributed under the terms of the Creative Commons Attribution License (CC BY). The use, distribution or reproduction in other forums is permitted, provided the original author(s) and the copyright owner(s) are credited and that the original publication in this journal is cited, in accordance with accepted academic practice. No use, distribution or reproduction is permitted which does not comply with these terms.
*Correspondence: Linda Söller, U29lbGxlckBnZW8udW5pLWZyYW5rZnVydC5kZQ==