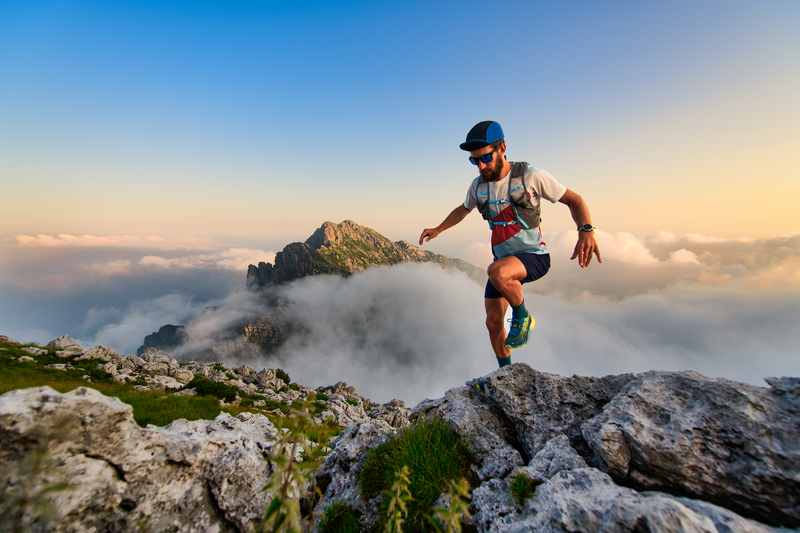
95% of researchers rate our articles as excellent or good
Learn more about the work of our research integrity team to safeguard the quality of each article we publish.
Find out more
REVIEW article
Front. Water , 22 April 2024
Sec. Environmental Water Quality
Volume 6 - 2024 | https://doi.org/10.3389/frwa.2024.1380133
This article is part of the Research Topic Water Quality Monitoring and Sustainable Use of Ambient Freshwaters View all 8 articles
Water quality issues remain a major cause of global water insecurity, and real-time low-cost monitoring solutions are central to the remediation and management of water pollution. Optical sensors, based on fluorescence, absorbance, scattering and reflectance-based principles, provide effective water quality monitoring (WQM) solutions. However, substantial challenges remain to their wider adoption across scales and environments amid cost and calibration-related concerns. This review discusses the current and future challenges in optical water quality monitoring based on multi-peak fluorescence, full-spectrum absorbance, light-scattering and remotely sensed surface reflectance. We highlight that fluorescence-based sensors can detect relatively low concentrations of aromatic compounds (e.g., proteins and humic acids) and quantify and trace organic pollution (e.g., sewage or industrial effluents). Conversely, absorbance-based sensors (Ultraviolet-Visible-Infra-red, UV-VIS-IR) are suitable for monitoring a wider range of physiochemical variables (e.g., nitrate, dissolved organic carbon and turbidity). Despite being accurate under optimal conditions, measuring fluorescence and absorbance can be demanding in dynamic environments due to ambient temperature and turbidity effects. Scattering-based turbidity sensors provide a detailed understanding of sediment transport and, in conjunction, improve the accuracy of fluorescence and absorbance measurements. Recent advances in micro-sensing components such as mini-spectrometers and light emitting diodes (LEDs), and deep computing provide exciting prospects of in-situ full-spectrum analysis of fluorescence (excitation-emission matrices) and absorbance for improved understanding of interferants to reduce the signal-to-noise ratio, improve detection accuracies of existing pollutants, and enable detection of newer contaminants. We examine the applications combining in-situ spectroscopy and remotely sensed reflectance for scaling Optical WQM in large rivers, lakes and marine bodies to scale from point observations to large water bodies and monitor algal blooms, sediment load, water temperature and oil spills. Lastly, we provide an overview of future applications of optical techniques in detecting emerging contaminants in treated and natural waters. We advocate for greater synergy between industry, academia and public policy for effective pollution control and water management.
Water pollution is a major challenge to global water security, with >25% of the world’s population unable to access safe drinking water in 2022 (WHO and UNICEF, 2023). After accounting for water quality issues, the fraction increases to 40%, with key hotspots in India, China, the Middle East, the Mediterranean and Mexico (van Vliet et al., 2021; Caretta et al., 2022). Real-time and accurate monitoring of critical water pollution variables, including heavy metals, agricultural nutrients [e.g., nitrate and phosphorus, disease-causing bacteria, viruses and emerging contaminants such as microplastics and pesticides, remains a global challenge (Hannah et al., 2022)]. The lack of infrastructure, financial and technical resources and regulatory oversight significantly hamper water quality monitoring (WQM) efforts in developing countries. Similarly, despite having financial resources, developed countries are constrained by aging infrastructure, lack of investment, and low compliance and accountability (Kirschke et al., 2020; Hannah et al., 2022). Technological improvements can lower some of the financial and infrastructure-related issues and facilitate decision-making by enabling in-situ monitoring of key water quality variables identified under global conventions such as the United Nations’s Sustainable Development Goals (SDGs) 6.3.2, European Union (EU) Water Framework Directive (WFD) and World Health Organization’s (WHO) water quality guidelines (Warner, 2020; European Union, 2021; WHO, 2022). For example, SDG 6.3.2 details a list of water quality variables, including oxygen, salinity, macronutrients (Nitrogen, Phosphorus) and pH and defines a range of target values that reflect human and ecosystem health perspectives (Warner, 2020). Similarly, in Europe, the WFD focuses on improving the chemical and ecological conditions of both surface and groundwater through monitoring and holistic river basin management (European Union, 2021). However, this monitoring occurs at coarse intervals and often misses critical periods of pollutant transport and transformation (e.g., hot moments; McClain et al., 2003). Recent WHO guidelines advocate developing early-warning systems for predicting harmful algal blooms using in-situ monitoring approaches (WHO, 2022).
The technology for in-situ monitoring of physiochemical variables, such as temperature, electrical conductivity, pH, and dissolved oxygen (DO), is well-established (Banna et al., 2014; Silva et al., 2022). Recent developments have expanded the potential variables that can be monitored in-situ to include total and dissolved organic carbon (TOC and DOC, respectively), biological and chemical oxygen demand (BOD and COD, respectively), dissolved organic matter (DOM), fecal coliforms, agricultural pollutants (e.g., ammonium, nitrate, and phosphorus), ions (chloride, fluoride) and heavy metals (e.g., iron, arsenic) (Raich, 2013; Banna et al., 2014). However, given that human-health-related thresholds are more stringent than those for ecosystem that production and environmental occurrence of emerging contaminants (ECs) continues to increase, there is an urgent requirement for monitoring technology that can detect a wide range of variables accurately and across a broad spectrum of environmental conditions. Alongside sensor technology advances, data transfer (telemetry) and data visualizations support quick and effective stakeholder decision-making (Gholizadeh et al., 2016; Zaidi Farouk et al., 2023). Technological advances are also increasingly informing policy-making and riverine ecosystem restoration efforts toward improving the drinking and bathing qualities of rivers, lakes and marine waters (Johnson et al., 2008; Mouchel et al., 2021; Tiwari et al., 2021).
Recent advances in optical water quality monitoring (OWQM) techniques have improved the understanding of eutrophication and organic pollution in aquatic ecosystems (Baker, 2002; Hudson et al., 2007; Thakur and Devi, 2022). In-situ optical sensors measuring fluorescence, absorbance, and scattering have become particularly important for aquatic monitoring. Advancements in optical components, i.e., light emitting diode (LED) technology, and miniaturization of sensing elements such as photodiodes, photo-multiplier tubes, charge-coupled device (CCD), and complementary metal-oxide-semiconductor (CMOS) sensors, etc., have made the optical sensors, such as fluorimeter, absorbance and turbidity sensors, smaller in form-factor, lowered their power requirements and improved their field usability (Mukunda et al., 2022; Zainurin et al., 2022; Goblirsch et al., 2023). Fluorescence spectroscopy relies on the principle of Stokes shift, which is the spectral shift between incident (excitation, ex) light and emitted (em) light (detected orthogonally to incident light) from shorter to longer wavelengths (Figure 1). Full-spectrum applications enable measurement of the fluorescence signature in a three-dimensional excitation-emission matrix (EEM) and use parallel factor analysis (PARAFAC) tools to characterize specific fluorescence peaks associated with specific dissolved organic compounds (Murphy et al., 2013). The key fluorescence peaks include Peak A (ex/em: 260/380–460 nm) and Peak C (ex/em: 350/420–480 nm), both of which have been associated with humic-like material derived from terrestrial environments, Peak B (ex/em: 275/310 nm) and Peak T (ex/em: 275/340 nm), associated with Tyrosine and Tryptophan-like fluorescence (TLF), respectively, and Peak M (ex/em: 310/370–420 nm) associated with humic-like material derived from marine environment. However, EEM applications are still limited primarily to the laboratory, with most in-situ applications focusing on single or dual peak fluorescence to detect dissolved organic compounds (Peak T and Peak C; Khamis et al., 2017; Li et al., 2020).
Figure 1. Schematic diagram showing principles of absorbance, fluorescence and scattering-based probes with optical components and sample results. The optical components include light sources, such as LEDs and lamps, and detectors, such as photodiodes, photo-multiplier tubes (PMTs), and CCD/CMOS sensors. Data visualization is shown as representative graphs of full spectrum absorbance spectra (bottom-left) and fluorescence excitation-emission matrix (EEM, top-right). The sample EEM is adapted from Khamis et al. (2017).
Absorbance spectroscopy is based on the absorption of incident light at specific wavelengths by the variable of interest, resulting in the loss of transmitted light measured at 180° angle. Fluorescence can provide high specificity and sensitivity for a set of organic compounds but can saturate at high concentrations (i.e., the inner-filtering effect) and is prone to interferences due to the sample temperature and turbidity (Fellman et al., 2010; Carstea et al., 2020; Kimball et al., 2020). Conversely, absorbance can detect a larger pool of organic and inorganic compounds across a wider range of concentrations, albeit with limited specificity (Dai et al., 2022; Carter et al., 2023). Nephelometric sensors detect undissolved/suspended solid/sediment particles in water by measuring scattering at different angles to the incident light (0°), including back-scattering (30° ± 15° angle), side-scattering (90° angle) and forward-scattering (120° ± 15° angle) (Kitchener et al., 2017; Droujko et al., 2023). These are based on inverse attenuation, i.e., a higher concentration of total suspended solids (TSS) will cause higher scattering and vary considerably with particle size, shape, and wavelength of incident light. The side-scattering-based sensors measure turbidity as a proxy of TSS with good accuracy at low concentrations. In contrast, backward-scattering sensors are accurate at high TSS concentrations, and forward-scattering sensors cover a wider range of concentrations. Together, they provide a detailed understanding of sediment transport in water bodies and are used to improve the accuracy of fluorescence and absorbance measurements (Khamis et al., 2015; Lee et al., 2015). However, substantial challenges remain to the large-scale deployment of these technologies, given costs, power requirements and calibration-related concerns. Increasingly, remotely sensed reflectance-based platforms are being used to monitor water quality events, such as algal blooms, in freshwater lakes and marine habitats, but require validation against in-situ ground observations (Shi et al., 2019; Sagan et al., 2020; Windle and Silsbe, 2021).
Several review papers have summarized recent advances in water quality monitoring (Raich, 2013; Banna et al., 2014; Gholizadeh et al., 2016; Thakur and Devi, 2022; Zainurin et al., 2022; Zaidi Farouk et al., 2023), and while some have focused explicitly on in-situ optical sensors (Hudson et al., 2007; Carstea et al., 2016, 2020; Gunter et al., 2023), there is still a need for deeper understanding of this rapidly evolving technology and its potential for water quality monitoring at different scales. In this review, we provide a concise overview of the current and future challenges in in-situ optical monitoring of freshwater and marine bodies, focusing on fluorescence, full-spectrum absorbance, scattering and reflectance-based techniques. Further, we discuss the available market solutions and data sources using field-deployable optical sensors and their strengths and limitations. Lastly, we provide an overview of future applications of optical techniques in detecting novel/emerging contaminants in water.
Early research on the use of fluorescence spectroscopy for WQM used fiber optic sensors for a wide range of applications, including the detection of organic matter (TOC, DOC), oxygen (BOD, DO), biological agents (e.g., bacteria, viruses, algal blooms, etc.) and hydrocarbons (Scully, 1998; Ahmad and Reynolds, 1999). The commercial applications of these techniques were either limited to benchtop instruments (Phillips et al., 1974) or in the form of optical fiber-based sensors in the 1980s, which, along with electrochemical techniques such as ion selective electrodes (ISEs), have been widely used for a range of aquatic and industrial monitoring activities for more than half a century (Frant, 1997; Scully, 1998; Ahmad and Reynolds, 1999). Similarly, in-situ optical turbidity sensors have long been used to measure scattering at different angles to understand the composition of suspended solids in water bodies (Kitchener et al., 2017). In comparison, the application of fluorescence and absorbance spectroscopy for in-situ WQM is a recent trend. The emergence of commercial fluorometers and absorbance probes over the last ~20 years is primarily linked to the development of UV LEDs (<400 nm) and photodetectors (Johnson and Coletti, 2002; Rode et al., 2016; Mukunda et al., 2022).
Currently, optical water quality monitoring represents a multi-billion US dollar market comprising probes based on various measurement principles, e.g., nephelometry (scattering of light), absorbance and fluorescence. The availability of cost-effective and low-powered LEDs and detectors has driven the expansion of the in-situ OWQM market. Mukunda et al. (2022) provided a comprehensive review of conventional light sources (Arc lamps, Quartz halogen lamps, mercury lamps, etc.) and highlighted cost, bulkiness, short lifespan, drift in output with prolonged flashing and UV phototoxicity as major issues. Conversely, LEDs provide high power, longer lifespan, high signal-to-noise ratio, stable outputs, compact size and low costs, making them ideal for field-deployable OWQM sensors (Hart and JiJi, 2002; Mukunda et al., 2022). The early applications of Ultraviolet–Visible-Near Infra-Red (UV–VIS–NIR) LEDs were for biomedical analytical devices and ecophysiological sensing (i.e., plant chlorophyll), which were later adopted for water quality sensors (Mukunda et al., 2022). Early sensors used single or dual-beam LEDs to focus on fluorescence or absorbance measurements for a single variable of interest, e.g., nitrate and TOC, with a second beam often used for turbidity correction. The two major gaps in the wider adoption of LEDs for OWQM are the low efficiency of deep UV (<280 nm) and scattering-induced noise in the signal. Multi-chip LEDs, which position multiple LEDs on a single chip, along with deep learning techniques, provide a better opportunity to understand the sediment composition and develop robust correction factors (Hart and JiJi, 2002; Ighalo et al., 2021). However, the cost-effectiveness and power requirements of deep UV LEDs remain a significant bottleneck in developing low-cost OWQM probes.
Similar to LEDs, the use of photodetector technology, such as photodiodes, CCDs, PMTs, and, recently, linear CMOS arrays, for WQM has also evolved significantly (Spring, 2001; Mukunda et al., 2022). Photodiodes have a number of desirable properties, namely, low-cost, high specificity, narrow or broad range of wavelengths, low power requirements, and low data processing time. Thus, paired with monochromatic or band-pass filters (which allow transmission of specific wavelengths), low-light photodiodes and PMTs contribute to the affordability and compactness of OQWM probes. Optical detectors can also improve the signal-to-noise ratio by optimizing signal gain and modulating the sensor integration time. However, PMTs and photodiodes have no spatial discrimination, i.e., they convert the intensity of light into an electrical signal and cannot differentiate them according to wavelengths, which limits their applicability to broad spectrum (UV–VIS) sensing (Spring, 2001; Yokota et al., 2021). Miniaturizing traditional benchtop spectrometers into mini and micro-spectrometers provides new opportunities for developing field-deployable UV–VIS OWQM probes (Bouyé et al., 2016; Shi et al., 2022; Goblirsch et al., 2023). The mini/micro-spectrometers use a linear CCD or CMOS imaging sensor in a Czerny-Turner design or micrograting to achieve smaller form factors (Bouyé et al., 2016; Chen et al., 2022). However, the significant challenges to adopting mini/micro-spectrometers lie in cost-effectiveness, data analytics and power management.
The use of fluorescence and absorbance spectroscopy for in-situ WQM, while relatively new in comparison to electrochemical techniques (ion-selective electrodes have been used for half a century), has become well-established over the past 20 years (Johnson and Coletti, 2002; Rode et al., 2016). Fluorescence for monitoring Chlorophyll a and UV absorbance for nitrate measurements are among the most widely cited environmental monitoring applications (Arndt et al., 2022). In this case, the sensors are used to directly measure the variables of interest (Chlorophyll a and nitrate have specific absorbance and fluorescence spectra). Concurrently, there has been increasing interest in the use of absorbance as a surrogate (or proxy) for other difficult-to-measure variables, including the development of multivariate regression models to quantify DOC/TOC (Peacock et al., 2014; Gaviria Salazar et al., 2023), fecal coliforms (Nowicki et al., 2019; Sorensen et al., 2020; Dapkus et al., 2023), and phosphorus fractions (Vaughan et al., 2018). These approaches have recently utilized emerging data science and machine learning tools to develop more robust proxy models (see Carter et al., 2023).
In-situ fluorescence monitoring has been used for both quantitative and qualitative WQM. For example, pollution sources are tracked using fluorescence peaks associated with proteinaceous compounds (e.g., TLF; Baker et al., 2004; Lenaker et al., 2023) and optical brightening agents (Finegan and Hasenmueller, 2023) as (non-conservative) tracers of wastewater. Fluorescence has also been used to quantify DOC and BOD (Khamis et al., 2017, 2021) by measuring dual fluorescence peaks (tryptophan and humic-like fluorescence) and turbidity, which is vital for developing reliable proxy models. There has also been growing interest in using fluorescence to quantify microbial contamination in rivers (Baker et al., 2015; Bridgeman et al., 2015), groundwater (Nowicki et al., 2019; Sorensen et al., 2020; Dapkus et al., 2023), and potable water (Sorensen et al., 2018; Gunter et al., 2023). However, it is important to note that most studies to date focus on single or dual fluorescence peaks; hence, the associated models tend to be relatively simple compared to those developed for absorbance sensors.
Optical indices (rather than proxy models) have recently emerged as a viable approach for monitoring water quality. There are a large number of fluorescence indices associated with Excitation Emission Matrix spectroscopy (Fellman et al., 2010; Begum et al., 2023), which have not yet been implemented for field deployable sensors, although the use of the ratio of peak T:peak C fluorescence has been used as an indicator of wastewater/anthropogenic DOM in an urban river (Croghan et al., 2021). For absorbance, it is possible to calculate a broader range of optical indices using full-spectrum measurements in-situ. For example, the spectral slope, linked to the molecular weight of DOM, has been used to assess carbon quality dynamics across a land cover gradient (Vaughan et al., 2019).
In an exhaustive market survey for current probes based on absorbance and fluorescence spectroscopy, we found more than 32 products catering to various environmental, academic, and industrial applications. The survey results are summarized in Supplementary Table S1. We relied on the datasheets and specifications provided by the retailers to glean the relevant information or lack thereof. The product marketing material (e.g., brochures, webpages, datasheets and manuals) often lacked technological specifications and was thus ignored. However, they provide helpful information on the applications, technology-based demands, supply-side constraints, and insights into their future directions. The surveyed probes were segregated based on the measurement principles: absorbance and luminescence including fluorescence; the light source: LEDs and lamps; the detector: single or arrayed photodiodes; and variables of interest: single or multivariable, design and data post-processing methods.
Absorbance-based sensors dominate the market space (72%, N = 31). The single-variable probes (38%) focused on nitrate (NO3-N), nitrite (NO2-N), DO and DOM. The multivariable probes use specific wavelength LEDs ranging from 200 to 720 nm to observe absorbance, which is converted to equivalent COD, BOD, DOC, TOC, and Total Suspended Solid (TSS) values using calibration algorithms. The absorbance measurements are taken at 180° angle from the incident light and are particularly susceptible to changes in turbidity. Thus, most surveyed probes had a turbidity compensation component based on nephelometry principles and temperature compensation. Most probes also offer single or dual-beam (more common) corrections for absorbance using reference blanks.
Luminescence probes (28%) form a smaller proportion of the market and use fluorescence-based sensing for organic matter detection, including TLF, Chromophoric Dissolved Organic Matter (CDOM), Chlorophyll a, etc. and derive other variables such as BOD and even fecal coliforms through statistical extrapolation. Fluorescence measurements are prone to matrix-specific interference, such as inner-filtering effects (IFE) and thermal quenching, creating a non-linear relationship between intensity response and concentrations, especially at high fluorophore concentrations. In ex-situ (laboratory-based) experimental studies, absorbance measurements are used to correct the inner-filtering effects and improve the accuracy of the fluorescence measurements (Kimball et al., 2020). The technique is now widely used in benchtop spectrometers with dual-mode features for absorbance and fluorescence (e.g., Duetta, Horiba Pvt. Ltd.). However, combining absorbance and fluorescence measurements to reduce noise, increase the linear range and measurement repeatability and achieve higher detection accuracies remains challenging for in-situ water quality monitoring probes.
More than half of the surveyed sensors used LEDs of specific wavelengths, while less than a third preferred lamps, and some provided no information. The availability of affordable deep-UV LEDs (<280 nm, 41%) has led to the development of multiple commercial probes for nitrate, DO and DOM detection. UV–VIS LEDs (315–550 nm, 16%) are frequently used for absorbance and fluorescence measurement of dissolved organic matter (DOM) and algal activities in large water bodies, including marine environments. VIS (~550 nm) and NIR (~850 nm) LEDs are standard for turbidity sensors nowadays. Lamps provide an alternative to multiple LEDs as a single broadband (~190 to ~750 nm) light source, including Xenon flash lamps, Deuterium and Tungsten. In comparison, mercury lamps are used for narrow-band (~254 nm) applications measuring nitrate. They benefit from simpler components and designs and allow simultaneous measurements of multiple variables. However, the tradeoffs with LEDs are in terms of power requirements and component costs, both being considerably higher for lamps. Furthermore, some sensors (24%) use more than one LED in a single-variable probe, in which case the second LED is used for turbidity or absorbance-based corrections.
Approximately two-thirds of the products do not specify the type of detector their probes use. Among the rest, photodiodes are the most common detectors in single or arrayed formations. Like LEDs, advancements in optical technology have made photodiodes affordable and miniaturized them for in-situ deployment. The LED-photodiode specificity allows for precise measurements of specific variables such as nitrate and reduces the scope of interference by other contaminants. However, the specificity limits the potential to account for background interferents, which full spectrum light source and detector combinations can observe and account for in a single measurement (Goblirsch et al., 2023). Current mini-spectrometers in the market are the size of a fingertip (C12880MA and C16767MA, Hamamatsu Pvt. Ltd.) and offer a broad wavelength UV–VIS–NIR range (225–1,000 nm, AFBR-S20M, Broadcom Inc.) in a single body. They use highly-sensitive image sensors such as CCD and CMOS and direct the incident light through optical slits and gratings. Depending on the type of applications, the mini-spectrometers can be tuned to maximum sensitivity at desired blaze angles and are likely to replace the photodiode arrays in future probes aimed at broad-spectrum sensing (Goblirsch et al., 2023). However, only one commercial product (Opus, Trios) is currently mentioned to use a mini-spectrometer (200–360 nm) as the detector, with most probes relying on an array of photodiodes, sensitive to wavelengths of interest as an alternative way of broad-spectrum sensing.
The design of the optical window of a probe is largely dependent on measurement principles. As explained earlier, the fluorescence and nephelometry measurements are taken at right angles (15–90°) to the incident light, and these probes preferred both incident light and detection inlet at the bottom of the sensor, albeit at a slight angle to each other. The design lends itself to better cleaning and antifouling properties. Conversely, the absorbance probes deploy a notched design, allowing the incident light to be 180° to the detector. This design enables path-lengths to be changed as per the application’s requirement and varied between 0.3–50 mm in the surveyed probes. Changeable path lengths are a crucial feature of absorbance probes, which allows them to target specific concentration ranges and resolutions based on the type of applications and environments (Jensen and Bak, 2002; Skouteris et al., 2018; Shi et al., 2022). For example, probes appealing to drinking water monitoring require higher sensitivity to low concentrations of pollutants and thus need longer path-lengths (10–50 mm), whereas probes serving effluent treatment plants monitor high concentration loads and require shorter path-lengths (0.3–5 mm). However, the notched design is relatively more susceptible to fouling than downward-facing probes and requires active cleaning mechanisms.
Most probes (85%) deployed one or more automatic cleaning methods such as integrated wipers (47%), compressed air (37%), compressed water (9%) and even the use of ultrasonic waves (NiCaVis, Xylem Analytics). Few probes (22%) were solely based on flow-through designs, although many offered them as accessories for drinking water and other process applications. Probes with a flow-through design tend to rely on chemical cleaning or compressed air and have built-in humidity control systems to reduce the noise. Another important approach to minimize the impacts of fouling involves the use of nano-particle coating for optical windows (6%) (Delgado et al., 2021).
The most common method of data interfacing in the probes is via a USB/R232/SI-12 cable, although newer probes come with Bluetooth and WLAN options. Most probes (63%) provided factory calibration of the sensors with optional recommendations of field calibration using standard reagents or comparisons with laboratory-based results. The data analysis and display were primarily server and web-dashboard-based. Despite considerable use in research, fewer probes mention post-processing capabilities or the use of artificial intelligence and machine learning tools, such as Liquid AI (Ighalo et al., 2021; Mustafa et al., 2021, Supplementary Table S1).
Ongoing advances in sensor technology and processing algorithms have led to multiple applications of reflectance spectroscopy for real-time monitoring of water bodies at different scales (Huang et al., 2018). Although cloud obscuration may be problematic for some platforms, the absorption and scattering properties of light are now being routinely monitored by various satellite platforms (Tyler et al., 2022), as well as airborne remote sensing and Unmanned Aerial Vehicles (UAVs) (Windle and Silsbe, 2021). In this context, the Inherent Optical Properties (IOPs) and color of water reflect the composition of optically active constituents in the medium, which include living phytoplankton (i.e., Chlorophyll a), dissolved organic matter (DOM) and inorganic matter (i.e., dissolved and particulate matter in water), as well as back-scattering by suspended particles. However, the challenges of using and applying remotely sensed optical data lie in resolving the different components of the reflectance signal, i.e., (a) downwelling irradiance, (b) sky radiance, and (c) water-leaving radiance (Mobley, 2001). While these challenges have somewhat constrained the use of remotely sensed optical data for catchment management, the examples summarized below illustrate their increasing potential to study a variety of applied problems, albeit where the real-time outputs are deemed usable after improved quality control and data processing algorithms (e.g., Matthews and Odermatt, 2015). For example, with respect to CDOM, which is often the main optical component of inland waters, the algorithms target the blue wavelengths of light, where absorption maxima occur. Still, atmospheric correction algorithms are weakest at this part of the spectrum, while studies of algal blooms focus on red wavelengths, where there is less interference from detrital pigments (Wynne et al., 2008).
The utility of satellite data for WQM can be significantly enhanced by integrating the optical output from remote sensing platforms with in-situ hydrological data, yielding increasing quantities of continuous data. Most sensor platforms are positioned in sun-synchronous, low-Earth orbits (Tyler et al., 2016). Inevitably, there is a tradeoff between spatial, spectral and temporal resolution. Their spatial resolution ranges from coarse (>200 m; AVHRR, MODIS, VIIRS), medium resolution (5–100 m; Landsat, SPOT, Sentinel-2) and high resolution (<5 m; IKONOS, RapidEye, CubeSat). The temporal resolution varies from half a day for some coarse platforms (e.g., MODIS) to between 5 and 26 days for medium-resolution sensors and to potentially daily resolution for platforms such as WorldView and RapidEye (Huang et al., 2018). However, there are ongoing challenges with respect to untangling the multiple (dynamic) relationships between reflectance and absorbance for specific locations and conditions (Moshtaghi et al., 2021).
As the spatial and temporal resolution of optical data has improved, reflectance-based technology has been successfully applied for water quality monitoring in more optically complex environments, such as lake-water bodies (Kutser et al., 2005; Andrzej Urbanski et al., 2016; Botha et al., 2020), rivers (e.g., Sultana and Dewan, 2021), and marine pollution (Thorhaug et al., 2007; Lu et al., 2013; Moshtaghi et al., 2021). Further work in this area requires paired optical and biogeochemical sampling, as demonstrated by Castagna et al. (2022) in their dataset from nine coastal and inland water bodies in Belgium, which included IOPs (e.g., absorption, scattering, beam attenuation and turbidity) and biogeochemical (e.g., suspended sediment, mineral fraction, particle size distribution, pigment concentration, etc.) properties. Specifically, within boreal lakes, Erlandsson et al. (2012) investigated the UV–VIS absorbance spectra in 983 water bodies in Sweden to report that alkaline lakes generally had lower absorbance and steeper spectral slopes than acidic lakes with a shorter water retention time while providing a comparable (and cost-effective) measure of the quantity and quality of dissolved organic matter (DOM).
Given public health concerns, many studies have investigated the efficacy of using optical sensors to monitor cyanobacteria (algal blooms) in different water bodies. While cyanotoxins cannot be directly quantified, Chlorophyll a can determine algal concentrations (Stumpf et al., 2016), with an absorption peak at 620 nm. Ambrose-Igho et al. (2021) tested algorithms enabling reflectance data from Sentinel-2 for monitoring algal blooms in two reservoirs in Illinois, United States. Coffer et al. (2020) investigated the optical properties of >2,300 lakes of various sizes in the contiguous USA using data from Envisat’s Medium Resolution Imaging Spectrophometer and Sentinel 3 to examine the seasonal growth and decay of algal blooms and using a spectral shape algorithm previously described by Wynne et al. (2008). The latter suggests that cyanobacteria are present if absorbance at 681 nm is below a baseline (defined by a line drawn between the 665 nm and 709 nm absorbance bands).
This selection of studies illustrates the increasing possibilities of using and applying optical sensors on various platforms to manage water resources at different scales. While problems with data accessibility and interoperability remain (e.g., Agnoli et al., 2023), some studies have successfully combined data from other satellite platforms (e.g., Zhao et al., 2020), as well as UAVs and in-situ sensors. Shi et al. (2019) highlight the need for automated processing algorithms that can be validated at increasing spatial and temporal resolution. However, the utility of these data is likely to be enhanced when combined with information from other sources, including in-situ monitoring, laboratory analyses and numerical modeling.
The recent emergence of low-cost microcontrollers and other electronic components has led to an increase in the development of “Do It Yourself (DIY)” environmental monitoring solutions for varied applications (Mao et al., 2019). A wide range of sensors and associated data loggers have been proposed for monitoring water quantity and quality (Chan et al., 2021), from non-contact water level sensors using ultrasonic (Pereira et al., 2022) or Light Detection and Ranging (Lidar) technology (Paul et al., 2020) to water quality sondes (Kinar and Brinkmann, 2022). However, most developments have been linked to physical or electrochemical sensors, with optical sensors for monitoring water quality largely neglected, except for turbidity (Kelley et al., 2014; Droujko et al., 2023).
While there have been some examples of field deployable spectroscopy-based sensors, notably low-cost fluorometers for measuring Chlorophyll a (Leeuw et al., 2013) or organic matter (Bridgeman et al., 2015), examples of absorbance (UV–VIS) based sensors are limited, but note a recent study by Goblirsch et al. (2023). The lack of UV–VIS probes has been primarily due to technological limitations, such as the challenges associated with LED costs and stability (Kneissl et al., 2019) at the deeper UV wavelengths required for nutrient or organic matter detection (Etheridge et al., 2014). Furthermore, a lack of suitable off-the-shelf detector solutions has impeded the development of lower-cost full-spectrum absorbance sensors (Ruhala and Zarnetske, 2017). Historically, expensive and bulky monochromators have been required for precise measurements, with the recent emergence of lower-cost solutions often lacking the needed range, resolution or measurement repeatability (e.g., AS7262 Visible Spectral Sensor). The few low-cost spectrophotometers/fluorometers reported in the literature leave many critical unanswered questions. Most studies report on short-term field deployments (Leeuw et al., 2013) or laboratory testing (Power et al., 2023). Hence, understanding measurement stability (electronic drift) or fouling rates (devices lack an automated cleaning system) remains limited (Delgado et al., 2021). Further, there has been a lack of multiple devices fabricated to enable cross-comparisons between sensors to quantify inter-sensor measurement uncertainty (Deutsch et al., 2018; Fettweis et al., 2019). Also, challenges associated with the calibration of multi-node sensor networks (lower-cost sensors will potentially facilitate more extensive networks of optical sensors) and best practices for using calibration reference standards, mainly when sensors are deployed in remote locations, are poorly defined (Earp et al., 2011).
Globally, 27% of the population drinks water contaminated from fecal sources (bio-pollution), of which the majority are in Southeast Asia (34%) and rural areas (41%) (Bain et al., 2014). Changing agricultural practices and urbanization have added or enhanced several emerging contaminants (ECs), such as pesticides, microplastics, pharmaceuticals, etc., creating a cocktail of water pollutants (Rochman, 2018; Sharma et al., 2019). Besides their potentially carcinogenic effects, ECs, like microplastics, are also seen as vectors and substratum for pathogenic bacteria, particularly in low-concentration groundwater environments (Rochman, 2018; Ma et al., 2020). Thus, detecting ECs in effluents and natural waters is a high priority, although limited to traditional “gold standard” laboratory-based methods using benchtop instruments and techniques (Manivannan et al., 2022). Sgroi et al. (2017) analyzed the EEMs of samples from two river systems in Italy to report significant correlations between known fluorescence indexes and EC groups, e.g., humic-like peaks correlating with sucralose, sulfamethoxazole and carbamazepine, and ibuprofen and caffeine correlating with tyrosine-like (Peak B) fluorescent peaks. Similarly, Wasswa et al. (2019) used the PARAFAC method to analyse fluorescent signals from treated wastewater and natural waters in the USA using benchtop and portable fluorometers and reported significant associations between TLF and ibuprofen (a pharmaceutical drug), diesel and gasoline, and tyrosine-peaks with diesel, gasoline, caffeine, isoxathion (a pesticide) and lopinavir (a pharmaceutical drug). They also recommended using portable fluorometers for in-situ tracking of ECs by proxy associations, especially in waters with high background CDOM and TLF concentrations, such as tertiary wastewater, and during events of high EC contamination, such as spills and leakages. Paradina-Fernández et al. (2023) demonstrated the very-low detection limits achieved by a benchtop fluorometer and PARAFAC modeling while quantifying organic micropollutants (e.g., pharmaceuticals) in surface and wastewater in Sweden. Moshtaghi et al. (2021) used experimental VIS-IR reflectance data to validate the algorithms used to remotely detect marine microplastics. The significant challenges to using fluorescence spectroscopy lie in moderating the fluorescent quenching and reducing inner-filtering effects. Similarly, UV–VIS absorbance measurements could provide additional information for screening ECs in natural waters (Romão et al., 2017).
Table 1 summarizes the strengths and challenges of using optical sensors for in-situ water quality monitoring and the potential way forward. Currently, there is a wide range of commercially available sensors for in-situ monitoring of fluorescence and absorbance at high frequency (seconds to minutes resolution) (Banna et al., 2014; Bouyé et al., 2016; Mukunda et al., 2022). However, these remain expensive and inhibit widespread statutory or regulatory use (Hannah et al., 2022; Mukunda et al., 2022). Furthermore, high costs have constrained the development of distributed sensor networks in large river catchments, which could have helped identify sources of pollution, assess propagation and persistence and validate scaling up solutions using remotely sensed datasets. We suggest that recent reductions in component costs, both in terms of light sources (e.g., UV LEDs) and detectors (e.g., mini-spectrometers), previously barriers to the development of lower cost and power sensors, are likely to herald a new wave of affordable optical water quality sensors over the next 5 years (Goblirsch et al., 2023).
Some promising areas for future research involve integrating fluorescence, absorbance, and remote sensing (e.g., reflectance) to increase spatial coverage, particularly in large river networks (Cao et al., 2022; Castagna et al., 2022; Fang et al., 2022). Future sensor networks (i.e., node locations) should, whenever possible, align with statutory monitoring locations where manual sampling occurs routinely (Kinar and Brinkmann, 2022; Gaviria Salazar et al., 2023). This will ensure coupled datasets of laboratory and in-situ measurements, which are of comparable quality and can lead to the development of more rigorous calibration algorithms and proxy models (Ighalo et al., 2021; Paradina-Fernández et al., 2023). With the potential for more measurement data from more locations, there is a clear need for the community to embrace emerging artificial intelligence and machine learning (AI-ML) approaches to aid calibration, reduce the signal-to-noise ratio, and capture and correct drift and outliers. It will also facilitate the development of more robust proxy models for hard-to-measure variables such as phosphorus and critical classes of emerging contaminants such as pharmaceuticals and pesticides (Ighalo et al., 2021; Mustafa et al., 2021; Carter et al., 2023) and microplastics (Moshtaghi et al., 2021). Further, despite remaining unexplored, merging multiple data streams from in-situ WQM, i.e., absorbance, fluorescence, scattering and reflectance, with remotely sensed surface reflectance data can facilitate the development of predictive water quality models and early warning systems for aiding tracking and managing large-scale events, such as, e.g., nutrient enrichment and harmful algal blooms (Briciu-Burghin et al., 2023).
In this review, we have highlighted the current state of optical water quality monitoring and identified several challenges and future opportunities. The key concerns are high costs and power requirements, biofouling-induced sensor drift (particularly in dynamic environments with variable turbidity), the need for site-specific calibrations, and the limitation of current commercial WQM probes in performing full-spectrum EEMs in-situ. The rapid growth in UV-LED and full-spectrum detector technologies will likely address these issues. At the same time, the emergence of AI-ML-based data analytics holds the potential to develop universal sensor correction algorithms and proxy models for key variables of interest. Fluorescence spectroscopy may be useful in certain scenarios to detect novel/emerging contaminants in treated and natural waters. We suggest that attention should shift toward multivariable and multi-method (fluorescence, absorbance, scattering and reflectance) optical water quality monitoring, which can enhance existing water management and lead to the development of new applications. Augmenting remotely sensed surface-reflectance datasets with improved on-ground WQM infrastructure would be critical for remote monitoring of large water bodies, including marine and coastal pollution. An emerging application for multi-method monitoring and data fusion is linked to the control and monitoring of stormwater systems and combined sewer overflows. With increasing public awareness and newer environmental laws pushing for stricter monitoring of our water resources, the commercial scope of optical WQM sensors is bound to increase significantly. Thus, we advocate for greater synergy between industry, academia and public policy to ensure technological development supports effective pollution control and water management.
MK: Conceptualization, Formal analysis, Methodology, Visualization, Writing – original draft, Writing – review & editing. KK: Conceptualization, Funding acquisition, Writing – original draft, Writing – review & editing. RS: Funding acquisition, Writing – review & editing. DH: Conceptualization, Funding acquisition, Project administration, Writing – review & editing. CB: Conceptualization, Funding acquisition, Methodology, Writing – original draft, Writing – review & editing.
The author(s) declare that financial support was received for the research, authorship, and/or publication of this article. This research is supported by Innovate UK and represents outcomes from a Knowledge Transfer Partnership (KTP) between The University of Birmingham and Proteus Instruments Limited (KTP no. 13235). KK and CB are also supported by a Natural Environmental Research Council grant, NE/X011178/1.
The authors are thankful to Joshua Walmsley-Scotland, Donald Miller and Pillip Tierney at Proteus Instruments Limited for their knowledge-sharing and input. The authors thank Mayank Singh for graphical design support.
RS was employed by Proteus Instruments Ltd.
The authors declare that this study received funding from Innovate UK (KTP Grant no. 13235) in partnership with the University of Birmingham and Proteus Instruments Limited. RS is employed by Proteus Instruments Limited; and he was involved in the writing and editing of this article.
The remaining authors declare that the research was conducted in the absence of any commercial or financial relationships that could be construed as a potential conflict of interest.
The author(s) declared that they were an editorial board member of Frontiers, at the time of submission. This had no impact on the peer review process and the final decision.
All claims expressed in this article are solely those of the authors and do not necessarily represent those of their affiliated organizations, or those of the publisher, the editors and the reviewers. Any product that may be evaluated in this article, or claim that may be made by its manufacturer, is not guaranteed or endorsed by the publisher.
The Supplementary material for this article can be found online at: https://www.frontiersin.org/articles/10.3389/frwa.2024.1380133/full#supplementary-material
Agnoli, L., Urquhart, E., Georgantzis, N., Schaeffer, B., Simmons, R., Hoque, B., et al. (2023). Perspectives on user engagement of satellite Earth observation for water quality management. Technol. Forecast. Soc. Change 189:122357. doi: 10.1016/j.techfore.2023.122357
Ahmad, S. R., and Reynolds, D. M. (1999). Monitoring of water quality using fluorescence technique: Prospect of on-line process control. Water Res. 33, 2069–2074. doi: 10.1016/S0043-1354(98)00435-7
Ambrose-Igho, G., Seyoum, W. M., Perry, W. L., and O'Reilly, C. M. (2021). Spatiotemporal analysis of water quality indicators in small lakes using sentinel-2 satellite data: lake bloomington and evergreen lake, Central Illinois, USA. Environ. Process. 8, 637–660. doi: 10.1007/s40710-021-00519-x
Andrzej Urbanski, J., Wochna, A., Bubak, I., Grzybowski, W., Lukawska-Matuszewska, K., Łącka, M., et al. (2016). Application of Landsat 8 imagery to regional-scale assessment of lake water quality. Int. J. Appl. Earth Obs. Geoinf. 51, 28–36. doi: 10.1016/j.jag.2016.04.004
Arndt, J., Kirchner, J. S., Jewell, K. S., Schluesener, M. P., Wick, A., Ternes, T. A., et al. (2022). Making waves: time for chemical surface water quality monitoring to catch up with its technical potential. Water Res. 213:118168. doi: 10.1016/j.watres.2022.118168
Bain, R., Cronk, R., Hossain, R., Bonjour, S., Onda, K., Wright, J., et al. (2014). Global assessment of exposure to faecal contamination through drinking water based on a systematic review. Trop. Med. Int. Heal. 19, 917–927. doi: 10.1111/tmi.12334
Baker, A. (2002). Fluorescence properties of some farm wastes: Implications for water quality monitoring. Water Res. 36, 189–195. doi: 10.1016/S0043-1354(01)00210-X
Baker, A., Cumberland, S. A., Bradley, C., Buckley, C., and Bridgeman, J. (2015). To what extent can portable fluorescence spectroscopy be used in the real-time assessment of microbial water quality? Sci. Total Environ. 532, 14–19. doi: 10.1016/j.scitotenv.2015.05.114
Baker, A., Ward, D., Lieten, S. H., Periera, R., Simpson, E. C., and Slater, M. (2004). Measurement of protein-like fluorescence in river and waste water using a handheld spectrophotometer. Water Res. 38, 2934–2938. doi: 10.1016/j.watres.2004.04.023
Banna, M. H., Imran, S., Francisque, A., Najjaran, H., Sadiq, R., Rodriguez, M., et al. (2014). Online drinking water quality monitoring: Review on available and emerging technologies. Crit. Rev. Environ. Sci. Technol. 44, 1370–1421. doi: 10.1080/10643389.2013.781936
Begum, M. S., Park, J. H., Yang, L., Shin, K. H., and Hur, J. (2023). Optical and molecular indices of dissolved organic matter for estimating biodegradability and resulting carbon dioxide production in inland waters: A review. Water Res. 228:119362. doi: 10.1016/j.watres.2022.119362
Botha, E. J., Anstee, J. M., Sagar, S., Lehmann, E., and Medeiros, T. A. G. (2020). Classification of Australian waterbodies across a wide range of optical water types. Remote Sens. 12:3018. doi: 10.3390/RS12183018
Bouyé, C., Kolb, H., and d’Humières, B. (2016). Mini and micro spectrometers pave the way to on-field advanced analytics. Photonic Instrum. Eng. III 9754:975408. doi: 10.1117/12.2212384
Briciu-Burghin, C., Power, S., Delgado, A., and Regan, F. (2023). Sensors for Coastal and Ocean Monitoring. Annu. Rev. Anal. Chem. 16, 451–469. doi: 10.1146/annurev-anchem-091922-085746
Bridgeman, J., Baker, A., Brown, D., and Boxall, J. B. (2015). Portable LED fluorescence instrumentation for the rapid assessment of potable water quality. Sci. Total Environ. 524–525, 338–346. doi: 10.1016/j.scitotenv.2015.04.050
Cao, Z., Shen, M., Kutser, T., Liu, M., Qi, T., Ma, J., et al. (2022). What water color parameters could be mapped using MODIS land reflectance products: A global evaluation over coastal and inland waters. Earth Sci. Rev. 232:104154. doi: 10.1016/j.earscirev.2022.104154
Caretta, M. A., Mukherji, A., Arfanuzzaman, M., Betts, R. A., Gelfan, A., Hirabayashi, Y., et al. (2022). “Water” in Climate Change 2022: Impacts, Adaptation and Vulnerability. Contribution of Working Group II to the Sixth Assessment Report of the Intergovernmental Panel on Climate Change. eds. B. R. H.-O. Pörtner, D. C. Roberts, M. Tignor, E. S. Poloczanska, K. Mintenbeck, and A. Alegría, et al. (Cambridge, New York, NY: Cambridge University Press), 551–712.
Carstea, E. M., Bridgeman, J., Baker, A., and Reynolds, D. M. (2016). Fluorescence spectroscopy for wastewater monitoring: A review. Water Res. 95, 205–219. doi: 10.1016/j.watres.2016.03.021
Carstea, E. M., Popa, C. L., Baker, A., and Bridgeman, J. (2020). In situ fluorescence measurements of dissolved organic matter: A review. Sci. Total Environ. 699:134361. doi: 10.1016/j.scitotenv.2019.134361
Carter, J. B., Huffaker, R., Singh, A., and Bean, E. (2023). HUM: A review of hydrochemical analysis using ultraviolet-visible absorption spectroscopy and machine learning. Sci. Total Environ. 901:165826. doi: 10.1016/j.scitotenv.2023.165826
Castagna, A., Martínez, L. A., Bogorad, M., Daveloose, I., Dasseville, R., Dierssen, H. M., et al. (2022). Optical and biogeochemical properties of diverse Belgian inland and coastal waters. Earth Syst. Sci. Data 14, 2697–2719. doi: 10.5194/essd-14-2697-2022
Chan, K., Schillereff, D. N., Baas, A. C. W., Chadwick, M. A., Main, B., Mulligan, M., et al. (2021). Low-cost electronic sensors for environmental research: Pitfalls and opportunities. Prog. Phys. Geogr. Earth Environ. 45, 305–338. doi: 10.1177/0309133320956567
Chen, S., Jia, Y., Chen, H., Yang, W., Luo, Y., Li, Z., et al. (2022). Dual-wavelength-excitation aerosol fluorescence spectra detection using combined spectrometer with Czerny-Turner design. Spectrochim. Acta A Mol. Biomol. Spectrosc. 277:121260. doi: 10.1016/j.saa.2022.121260
Coffer, M. M., Schaeffer, B. A., Darling, J. A., Urquhart, E. A., and Salls, W. B. (2020). Quantifying national and regional cyanobacterial occurrence in US lakes using satellite remote sensing. Ecol. Indic. 111:105976. doi: 10.1016/j.ecolind.2019.105976
Croghan, D., Khamis, K., Bradley, C., Van Loon, A. F., Sadler, J., and Hannah, D. M. (2021). Combining in-situ fluorometry and distributed rainfall data provides new insights into natural organic matter transport dynamics in an urban river. Sci. Total Environ. 755:142731. doi: 10.1016/j.scitotenv.2020.142731
Dai, Y., Wang, H., Wang, J., Wang, X., Wang, Z., and Ge, X. (2022). Prediction of water quality based on SVR by fluorescence excitation-emission matrix and UV–Vis absorption spectrum. Spectrochim. Acta Part A Mol. Biomol. Spectrosc. 273:121059. doi: 10.1016/j.saa.2022.121059
Dapkus, R. T., Fryar, A. E., Tobin, B. W., Byrne, D. M., Sarker, S. K., Bettel, L., et al. (2023). Utilization of tryptophan-like fluorescence as a proxy for E. coli contamination in a mixed-land-use karst basin. Hydrology 10, 1–12. doi: 10.3390/hydrology10040074
Delgado, A., Briciu-Burghina, C., and Regan, F. (2021). Antifouling strategies for sensors used in water monitoring: Review and future perspectives. Sensors (Switzerland) 21, 1–25. doi: 10.3390/s21020389
Deutsch, E. S., Alameddine, I., and El-Fadel, M. (2018). Monitoring water quality in a hypereutrophic reservoir using Landsat ETM+ and OLI sensors: how transferable are the water quality algorithms? Environ. Monit. Assess. 190:141. doi: 10.1007/s10661-018-6506-9
Droujko, J., Kunz, F., and Molnar, P. (2023). Ötz-T: 3D-printed open-source turbidity sensor with Arduino shield for suspended sediment monitoring. HardwareX 13:e00395. doi: 10.1016/j.ohx.2023.e00395
Earp, A., Hanson, C. E., Ralph, P. J., Brando, V. E., Allen, S., Baird, M., et al. (2011). Review of fluorescent standards for calibration of in situ fluorometers: Recommendations applied in coastal and ocean observing programs. Opt. Express 19:26768. doi: 10.1364/oe.19.026768
Erlandsson, M., Futter, M. N., Kothawala, D. N., and Köhler, S. J. (2012). Variability in spectral absorbance metrics across boreal lake waters. J. Environ. Monit. 14, 2643–2652. doi: 10.1039/c2em30266g
Etheridge, J. R., Birgand, F., Osborne, J. A., Osburn, C. L., Burchell, M. R., and Irving, J. (2014). Using in situ ultraviolet-visual spectroscopy to measure nitrogen, carbon, phosphorus, and suspended solids concentrations at a high frequency in a brackish tidal marsh. Limnol. Oceanogr. Methods 12, 10–22. doi: 10.4319/lom.2014.12.10
European Union (2021). Good-quality water in Europe (EU Water Directive). 13–15. Available at: http://eur-lex.europa.eu/legal-content/EN/LSU/?uri=CELEX:32000L0060.
Fang, C., Song, K., Paerl, H. W., Jacinthe, P. A., Wen, Z., Liu, G., et al. (2022). Global divergent trends of algal blooms detected by satellite during 1982–2018. Glob. Chang. Biol. 28, 2327–2340. doi: 10.1111/gcb.16077
Fellman, J. B., Hood, E., and Spencer, R. G. M. (2010). Fluorescence spectroscopy opens new windows into dissolved organic matter dynamics in freshwater ecosystems: A review. Limnol. Oceanogr. 55, 2452–2462. doi: 10.4319/lo.2010.55.6.2452
Fettweis, M., Riethmüller, R., Verney, R., Becker, M., Backers, J., Baeye, M., et al. (2019). Uncertainties associated with in situ high-frequency long-term observations of suspended particulate matter concentration using optical and acoustic sensors. Prog. Oceanogr. 178:102162. doi: 10.1016/j.pocean.2019.102162
Finegan, C. R., and Hasenmueller, E. A. (2023). Using in situ measurements of optical brighteners for rapid reconnaissance of wastewater inputs to water resources. Sci. Total Environ. 881:163378. doi: 10.1016/j.scitotenv.2023.163378
Frant, M. S. (1997). Where did ion selective electrodes come from? The story of their development and commercialization. J. Chem. Educ. 74, 159–166. doi: 10.1021/ed074p159
Gaviria Salazar, C., Roebuck, J. A., Myers-Pigg, A. N., and Ziegler, S. (2023). Self-diagnosis of model suitability for continuous measurements of stream-dissolved organic carbon derived from in situ UV–visible spectroscopy. Limnol. Oceanogr. Methods 21, 478–494. doi: 10.1002/lom3.10559
Gholizadeh, M. H., Melesse, A. M., and Reddi, L. (2016). A comprehensive review on water quality parameters estimation using remote sensing techniques. Sensors (Switzerland) 16:1298. doi: 10.3390/s16081298
Goblirsch, T., Mayer, T., Penzel, S., Rudolph, M., and Borsdorf, H. (2023). In situ water quality monitoring using an optical multiparameter sensor probe. Sensors 23:9545. doi: 10.3390/s23239545
Gunter, H., Bradley, C., Hannah, D. M., Manaseki-Holland, S., Stevens, R., and Khamis, K. (2023). Advances in quantifying microbial contamination in potable water: Potential of fluorescence-based sensor technology. Wiley Interdiscip. Rev. Water 10, 1–19. doi: 10.1002/wat2.1622
Hannah, D. M., Abbott, B. W., Khamis, K., Kelleher, C., Lynch, I., Krause, S., et al. (2022). Illuminating the 'invisible water crisis' to address global water pollution challenges. Hydrol. Process. 36, 1–5. doi: 10.1002/hyp.14525
Hart, S. J., and JiJi, R. D. (2002). Light emitting diode excitation emission matrix fluorescence spectroscopy. Analyst 127, 1693–1699. doi: 10.1039/b207660h
Huang, C., Chen, Y., Zhang, S., and Wu, J. (2018). Detecting, extracting, and monitoring surface water from space using optical sensors: a review. Rev. Geophys. 56, 333–360. doi: 10.1029/2018RG000598
Hudson, N., Baker, A., and Reynolds, D. (2007). Fluorescence analysis of dissolved organic matter in natural, waste and polluted waters—a review. River Res. Appl. 23, 631–649. doi: 10.1002/rra
Ighalo, J. O., Adeniyi, A. G., and Marques, G. (2021). Artificial intelligence for surface water quality monitoring and assessment: a systematic literature analysis. Model. Earth Syst. Environ. 7, 669–681. doi: 10.1007/s40808-020-01041-z
Jensen, P. S., and Bak, J. (2002). Near-infrared transmission spectroscopy of aqueous solutions: Influence of optical path-length on signal-to-noise ratio. Appl. Spectrosc. 56, 1600–1606. doi: 10.1366/000370202321115878
Johnson, K. S., and Coletti, L. J. (2002). In situ ultraviolet spectrophotometry for high resolution and long-term monitoring of nitrate, bromide and bisulfide in the ocean. Deep. Res. Part I Oceanogr. Res. Pap. 49, 1291–1305. doi: 10.1016/S0967-0637(02)00020-1
Johnson, E. K., Moran, D., and Vinten, A. J. A. (2008). A framework for valuing the health benefits of improved bathing water quality in the River Irvine catchment. J. Environ. Manag. 87, 633–638. doi: 10.1016/j.jenvman.2007.06.021
Kelley, C. D., Krolick, A., Brunner, L., Burklund, A., Kahn, D., Ball, W. P., et al. (2014). An affordable open-source turbidimeter. Sensors (Switzerland) 14, 7142–7155. doi: 10.3390/s140407142
Khamis, K., Bradley, C., Gunter, H. J., Basevi, G., Stevens, R., and Hannah, D. M. (2021). Calibration of an in-situ fluorescence-based sensor platform for reliable BOD5 measurement in wastewater. Water Sci. Technol. 83, 3075–3091. doi: 10.2166/wst.2021.197
Khamis, K., Bradley, C., Stevens, R., and Hannah, D. M. (2017). Continuous field estimation of dissolved organic carbon concentration and biochemical oxygen demand using dual-wavelength fluorescence, turbidity and temperature. Hydrol. Process. 31, 540–555. doi: 10.1002/hyp.11040
Khamis, K., Ouellet, V., Croghan, D., Hernandez Gonzalez, L. M., Packman, A. I., Hannah, D. M., et al. (2023). The Autobot-WQ: A portable, low-cost autosampler to provide new insight into urban spatio-temporal water quality dynamics. Front. Built Environ. 9:1072757. doi: 10.3389/fbuil.2023.1072757
Khamis, K., Sorensen, J. P. R., Bradley, C., Hannah, D. M., Lapworth, D. J., and Stevens, R. (2015). In situ tryptophan-like fluorometers: Assessing turbidity and temperature effects for freshwater applications. Environ Sci Process Impacts 17, 740–752. doi: 10.1039/c5em00030k
Kimball, J., Chavez, J., Ceresa, L., Kitchner, E., Nurekeyev, Z., Doan, H., et al. (2020). On the origin and correction for inner filter effects in fluorescence Part I: Primary inner filter effect-the proper approach for sample absorbance correction. Methods Appl. Fluoresc. 8:033002. doi: 10.1088/2050-6120/ab947c
Kinar, N. J., and Brinkmann, M. (2022). Development of a sensor and measurement platform for water quality observations: design, sensor integration, 3D printing, and open-source hardware. Environ. Monit. Assess. 194:207. doi: 10.1007/s10661-022-09825-9
Kirschke, S., Avellán, T., Bärlund, I., Bogardi, J. J., Carvalho, L., Chapman, D., et al. (2020). Capacity challenges in water quality monitoring: understanding the role of human development. Environ. Monit. Assess. 192:298. doi: 10.1007/s10661-020-8224-3
Kitchener, B. G. B., Wainwright, J., and Parsons, A. J. (2017). A review of the principles of turbidity measurement. Prog. Phys. Geogr. 41, 620–642. doi: 10.1177/0309133317726540
Kneissl, M., Seong, T. Y., Han, J., and Amano, H. (2019). The emergence and prospects of deep-ultraviolet light-emitting diode technologies. Nat. Photonics 13, 233–244. doi: 10.1038/s41566-019-0359-9
Kutser, T., Pierson, D. C., Kallio, K. Y., Reinart, A., and Sobek, S. (2005). Mapping lake CDOM by satellite remote sensing. Remote Sens. Environ. 94, 535–540. doi: 10.1016/j.rse.2004.11.009
Lee, E. J., Yoo, G. Y., Jeong, Y., Kim, K. U., Park, J. H., and Oh, N. H. (2015). Comparison of UV-VIS and FDOM sensors for in situ monitoring of stream DOC concentrations. Biogeosciences 12, 3109–3118. doi: 10.5194/bg-12-3109-2015
Leeuw, T., Boss, E. S., and Wright, D. L. (2013). In situ measurements of phytoplankton fluorescence using low cost electronics. Sensors (Switzerland) 13, 7872–7883. doi: 10.3390/s130607872
Lenaker, P. L., Corsi, S. R., De Cicco, L. A., Olds, H. T., Dila, D. K., Danz, M. E., et al. (2023). Modeled predictions of human-associated and Faecal-indicator bacteria concentrations and loadings in the Menomonee River, Wisconsin using in-situ optical sensors. PLoS One 18, 1–25. doi: 10.1371/journal.pone.0286851
Li, L., Wang, Y., Zhang, W., Yu, S., Wang, X., and Gao, N. (2020). New advances in fluorescence excitation-emission matrix spectroscopy for the characterization of dissolved organic matter in drinking water treatment: A review. Chem. Eng. J. 381:122676. doi: 10.1016/j.cej.2019.122676
Lu, Y., Li, X., Tian, Q., Zheng, G., Sun, S., Liu, Y., et al. (2013). Progress in marine oil spill optical remote sensing: detected targets, spectral response characteristics, and theories. Mar. Geod. 36, 334–346. doi: 10.1080/01490419.2013.793633
Ma, Y., Wang, L., Wang, T., Chen, Q., and Ji, R. (2020). Microplastics as vectors of chemicals and microorganisms in the environment. In: Particulate Plastics in Terrestrial and Aquatic Environments, 209–230.
Manivannan, B., Nallathambi, G., and Devasena, T. (2022). Alternative methods of monitoring emerging contaminants in water: a review. Environ Sci Process Impacts 24, 2009–2031. doi: 10.1039/d2em00237j
Mao, F., Khamis, K., Krause, S., Clark, J., and Hannah, D. M. (2019). Low-cost environmental sensor networks: recent advances and future directions. Front. Earth Sci. 7, 1–7. doi: 10.3389/feart.2019.00221
Matthews, M. W., and Odermatt, D. (2015). Improved algorithm for routine monitoring of cyanobacteria and eutrophication in inland and near-coastal waters. Remote Sens. Environ. 156, 374–382. doi: 10.1016/j.rse.2014.10.010
McClain, M. E., Boyer, E. W., Dent, C. L., Gergel, S. E., Grimm, N. B., Groffman, P. M., et al. (2003). Biogeochemical hot spots and hot moments at the interface of terrestrial and aquatic ecosystems. Ecosystems 6, 301–312. doi: 10.1007/s10021-003-0161-9
Mobley, C. D. (2001). “Radiative transfer in the ocean” in Encyclopedia of Ocean Sciences, 2321–2330.
Moshtaghi, M., Knaeps, E., Sterckx, S., Garaba, S., and Meire, D. (2021). Spectral reflectance of marine macroplastics in the VNIR and SWIR measured in a controlled environment. Sci. Rep. 11:5436. doi: 10.1038/s41598-021-84867-6
Mouchel, J. M., Lucas, F. S., Moulin, L., Wurtzer, S., Euzen, A., Haghe, J. P., et al. (2021). Bathing activities and microbiological river water quality in the paris area: a long-term perspective. Handb. Environ. Chem. 90, 323–353. doi: 10.1007/698_2019_397
Mukunda, D. C., Joshi, V. K., and Mahato, K. K. (2022). Light emitting diodes (LEDs) in fluorescence-based analytical applications: a review. Appl. Spectrosc. Rev. 57, 1–38. doi: 10.1080/05704928.2020.1835939
Murphy, K. R., Stedmon, C. A., Graeber, D., and Bro, R. (2013). Fluorescence spectroscopy and multi-way techniques, PARAFAC. Anal. Methods 5, 6557–6566. doi: 10.1039/c3ay41160e
Mustafa, H. M., Mustapha, A., Hayder, G., and Salisu, A. (2021). Applications of IoT and artificial intelligence in water quality monitoring and prediction: a review. Proceedings of the 6th International Conference on Computer Technology 2021, 968–975.
Nowicki, S., Lapworth, D. J., Ward, J. S. T., Thomson, P., and Charles, K. (2019). Tryptophan-like fluorescence as a measure of microbial contamination risk in groundwater. Sci. Total Environ. 646, 782–791. doi: 10.1016/j.scitotenv.2018.07.274
Paradina-Fernández, L., Wünsch, U., Bro, R., and Murphy, K. (2023). Direct measurement of organic micropollutants in water and wastewater using fluorescence spectroscopy. ACS EST Water 3, 3905–3915. doi: 10.1021/acsestwater.3c00323
Paul, J. D., Buytaert, W., and Sah, N. (2020). A technical evaluation of lidar-based measurement of river water levels. Water Resour. Res. 56:e2019WR026810. doi: 10.1029/2019WR026810
Peacock, M., Evans, C. D., Fenner, N., Freeman, C., Gough, R., Jones, T. G., et al. (2014). UV-visible absorbance spectroscopy as a proxy for peatland dissolved organic carbon (DOC) quantity and quality: Considerations on wavelength and absorbance degradation. Environ Sci Process Impacts 16, 1445–1461. doi: 10.1039/c4em00108g
Pereira, T. S. R., de Carvalho, T. P., Mendes, T. A., and Formiga, K. T. M. (2022). Evaluation of water level in flowing channels using ultrasonic sensors. Sustain. For. 14, 1–20. doi: 10.3390/su14095512
Phillips, S. L., Mack, D. A., and MacLeod, W. D. (1974). Instrumentation for water quality monitoring. Anal. Chem. 46, 345A–356A. doi: 10.1021/ac60339a715
Power, S. M., Free, L., Delgado, A., Richards, C., Alvarez-Gomez, E., Briciu-Burghina, C., et al. (2023). A novel low-cost plug-and-play multi-spectral LED based fluorometer, with application to chlorophyll detection. Anal. Methods 15, 5474–5482. doi: 10.1039/d3ay00991b
Raich, J. (2013). Review of sensors to monitor water quality. European reference network for critical infrastructure protection (ERNCIP) project. doi: 10.2788/35499
Rochman, C. M. (2018). Microplastics research — from sink to source in freshwater systems. Science 360, 28–29. doi: 10.1126/science.aar7734
Rode, M., Wade, A. J., Cohen, M. J., Hensley, R. T., Bowes, M. J., Kirchner, J. W., et al. (2016). Sensors in the stream: the high-frequency wave of the present. Environ. Sci. Technol. 50, 10297–10307. doi: 10.1021/acs.est.6b02155
Romão, J., Barata, D., Ribeiro, N., Habibovic, P., Fernandes, H., and Mul, G. (2017). High throughput screening of photocatalytic conversion of pharmaceutical contaminants in water. Environ. Pollut. 220, 1199–1207. doi: 10.1016/j.envpol.2016.11.015
Ruhala, S. S., and Zarnetske, J. P. (2017). Using in-situ optical sensors to study dissolved organic carbon dynamics of streams and watersheds: A review. Sci. Total Environ. 575, 713–723. doi: 10.1016/j.scitotenv.2016.09.113
Sagan, V., Peterson, K. T., Maimaitijiang, M., Sidike, P., Sloan, J., Greeling, B. A., et al. (2020). Monitoring inland water quality using remote sensing: potential and limitations of spectral indices, bio-optical simulations, machine learning, and cloud computing. Earth Sci. Rev. 205:103187. doi: 10.1016/j.earscirev.2020.103187
Scully, P. (1998). Optical techniques for water monitoring. Monit. Water Qual. Elsevier Science BV. 15–35. doi: 10.1016/b978-008043340-0/50003-5
Sgroi, M., Roccaro, P., Korshin, G. V., and Vagliasindi, F. G. A. (2017). Monitoring the behavior of emerging contaminants in wastewater-impacted rivers based on the use of fluorescence excitation emission matrixes (EEM). Environ. Sci. Technol. 51, 4306–4316. doi: 10.1021/acs.est.6b05785
Sharma, B. M., Bečanová, J., Scheringer, M., Sharma, A., Bharat, G. K., Whitehead, P. G., et al. (2019). Health and ecological risk assessment of emerging contaminants (pharmaceuticals, personal care products, and artificial sweeteners) in surface and groundwater (drinking water) in the Ganges River Basin, India. Sci. Total Environ. 646, 1459–1467. doi: 10.1016/j.scitotenv.2018.07.235
Shi, Z., Chow, C. W. K., Fabris, R., Liu, J., and Jin, B. (2022). Applications of online UV-vis spectrophotometer for drinking water quality monitoring and process control: a review. Sensors 22, 1–21. doi: 10.3390/s22082987
Shi, K., Zhang, Y., Qin, B., and Zhou, B. (2019). Remote sensing of cyanobacterial blooms in inland waters: present knowledge and future challenges. Sci. Bull. 64, 1540–1556. doi: 10.1016/j.scib.2019.07.002
Silva, G. M. E., Campos, D. F., Brasil, J. A. T., Tremblay, M., Mendiondo, E. M., and Ghiglieno, F. (2022). Advances in technological research for online and in situ water quality monitoring—a review. Sustain. For. 14, 1–28. doi: 10.3390/su14095059
Skouteris, G., Webb, D. P., Shin, K. L. F., and Rahimifard, S. (2018). Assessment of the capability of an optical sensor for in-line real-time wastewater quality analysis in food manufacturing. Water Resour. Ind. 20, 75–81. doi: 10.1016/j.wri.2018.10.002
Sorensen, J. P. R., Baker, A., Cumberland, S. A., Lapworth, D. J., MacDonald, A. M., Pedley, S., et al. (2018). Real-time detection of faecally contaminated drinking water with tryptophan-like fluorescence: defining threshold values. Sci. Total Environ. 622–623, 1250–1257. doi: 10.1016/j.scitotenv.2017.11.162
Sorensen, J. P. R., Diaw, M. T., Pouye, A., Roffo, R., Diongue, D. M. L., Faye, S. C., et al. (2020). In-situ fluorescence spectroscopy indicates total bacterial abundance and dissolved organic carbon. Sci. Total Environ. 738:139419. doi: 10.1016/j.scitotenv.2020.139419
Spring, K. R. (2001). “Detectors for fluorescence microscopy” in Methods in cellular imaging. ed. A. Periasamy, 40–52.
Stumpf, R. P., Davis, T. W., Wynne, T. T., Graham, J. L., Loftin, K. A., Johengen, T. H., et al. (2016). Challenges for mapping cyanotoxin patterns from remote sensing of cyanobacteria. Harmful Algae 54, 160–173. doi: 10.1016/j.hal.2016.01.005
Sultana, M. S., and Dewan, A. (2021). A reflectance-based water quality index and its application to examine degradation of river water quality in a rapidly urbanising megacity. Environ. Adv. 5:100097. doi: 10.1016/j.envadv.2021.100097
Thakur, A., and Devi, P. (2022). A comprehensive review on water quality monitoring devices: materials advances, current status, and future perspective. Crit. Rev. Anal. Chem. 1–26. doi: 10.1080/10408347.2022.2070838
Thorhaug, A., Richardson, A. D., and Berlyn, G. P. (2007). Spectral reflectance of the seagrasses: Thalassia testudinum, Halodule wrightii, Syringodium filiforme and five marine algae. Int. J. Remote Sens. 28, 1487–1501. doi: 10.1080/01431160600954662
Tiwari, A., Oliver, D. M., Bivins, A., Sherchan, S. P., and Pitkänen, T. (2021). Bathing water quality monitoring practices in europe and the United States. Int. J. Environ. Res. Public Health 18:5513. doi: 10.3390/ijerph18115513
Tyler, A., Hunter, P., De Keukelaere, L., Ogashawara, I., and Spyrakos, E. (2022). Remote sensing of inland water quality. Encycl. Inl. Waters Second Ed. 4, 570–584. doi: 10.1016/B978-0-12-819166-8.00213-9
Tyler, A. N., Hunter, P. D., Spyrakos, E., Groom, S., Constantinescu, A. M., and Kitchen, J. (2016). Developments in Earth observation for the assessment and monitoring of inland, transitional, coastal and shelf-sea waters. Sci. Total Environ. 572, 1307–1321. doi: 10.1016/j.scitotenv.2016.01.020
van Vliet, M. T. H., Jones, E. R., Flörke, M., Franssen, W. H. P., Hanasaki, N., Wada, Y., et al. (2021). Global water scarcity including surface water quality and expansions of clean water technologies. Environ. Res. Lett. 16:024020. doi: 10.1088/1748-9326/abbfc3
Vaughan, M. C. H., Bowden, W. B., Shanley, J. B., Vermilyea, A., and Schroth, A. W. (2019). Shining light on the storm: in-stream optics reveal hysteresis of dissolved organic matter character. Biogeochemistry 143, 275–291. doi: 10.1007/s10533-019-00561-w
Vaughan, M. C. H., Bowden, W. B., Shanley, J. B., Vermilyea, A., Wemple, B., and Schroth, A. W. (2018). Using in situ UV-Visible spectrophotometer sensors to quantify riverine phosphorus partitioning and concentration at a high frequency. Limnol. Oceanogr. Methods 16, 840–855. doi: 10.1002/lom3.10287
Warner, S. (2020). SDG Indicator 6.3.2 Technical Guidance Document No. 2: TARGET VALUES. 20200508. Available at: https://communities.unep.org/download/attachments/32407814/CDC_GEMI2_TechDoc2_Targetvalues_20200508.pdf?version=2&modificationDate=1595432590204&api=v2.
Wasswa, J., Mladenov, N., and Pearce, W. (2019). Assessing the potential of fluorescence spectroscopy to monitor contaminants in source waters and water reuse systems. Environ. Sci. Water Res. Technol. 5, 370–382. doi: 10.1039/c8ew00472b
WHO (2022). Guidelines for drinking-water quality: fourth edition incorporating the first and second addenda. Available at: https://iris.who.int/bitstream/handle/10665/352532/9789240045064-eng.pdf?sequence=1.
WHO and UNICEF (2023). Progress on Household Drinking Water, Sanitation and Hygiene (Jmp). Available at: http://apps.who.int/bookorders.
Windle, A. E., and Silsbe, G. M. (2021). Evaluation of unoccupied aircraft system (UAS) remote sensing reflectance retrievals for water quality monitoring in coastal waters. Front. Environ. Sci. 9, 1–15. doi: 10.3389/fenvs.2021.674247
Wynne, T. T., Stumpf, R. P., Tomlinson, M. C., Warner, R. A., Tester, P. A., Dyble, J., et al. (2008). Relating spectral shape to cyanobacterial blooms in the Laurentian Great Lakes. Int. J. Remote Sens. 29, 3665–3672. doi: 10.1080/01431160802007640
Yokota, H., Fukasawa, A., Hirano, M., and Ide, T. (2021). Low-light photodetectors for fluorescence microscopy. Appl. Sci. 11:2773. doi: 10.3390/app11062773
Zaidi Farouk, M. I. H., Jamil, Z., and Abdul Latip, M. F. (2023). Towards online surface water quality monitoring technology: A review. Environ. Res. 238:117147. doi: 10.1016/j.envres.2023.117147
Zainurin, S. N., Wan Ismail, W. Z., Mahamud, S. N. I., Ismail, I., Jamaludin, J., Ariffin, K. N. Z., et al. (2022). Advancements in monitoring water quality based on various sensing methods: a systematic review. Int. J. Environ. Res. Public Health 19:14080. doi: 10.3390/ijerph192114080
Zhao, Y., Liu, D., and Wei, X. (2020). Monitoring cyanobacterial harmful algal blooms at high spatiotemporal resolution by fusing Landsat and MODIS imagery. Environ. Adv. 2:100008. doi: 10.1016/j.envadv.2020.100008
Keywords: optical spectroscopy, fluorescence, absorbance, water quality monitoring, sensors, in-situ
Citation: Kumar M, Khamis K, Stevens R, Hannah DM and Bradley C (2024) In-situ optical water quality monitoring sensors—applications, challenges, and future opportunities. Front. Water. 6:1380133. doi: 10.3389/frwa.2024.1380133
Received: 01 February 2024; Accepted: 04 April 2024;
Published: 22 April 2024.
Edited by:
Timothy Sullivan, University College Cork, IrelandReviewed by:
Matthew Daniel Stocker, Agricultural Research Service (USDA), United StatesCopyright © 2024 Kumar, Khamis, Stevens, Hannah and Bradley. This is an open-access article distributed under the terms of the Creative Commons Attribution License (CC BY). The use, distribution or reproduction in other forums is permitted, provided the original author(s) and the copyright owner(s) are credited and that the original publication in this journal is cited, in accordance with accepted academic practice. No use, distribution or reproduction is permitted which does not comply with these terms.
*Correspondence: Manish Kumar, bS5rdW1hci4yQGJoYW0uYWMudWs=
†ORCID: Manish Kumar, http://orcid.org/0000-0002-6780-9336
Kieran Khamis, http://orcid.org/0000-0002-5203-3221
Rob Stevens, https://orcid.org/0009-0004-4725-0573
David M. Hannah, http://orcid.org/0000-0003-1714-1240
Chris Bradley, http://orcid.org/0000-0003-4042-867X
Disclaimer: All claims expressed in this article are solely those of the authors and do not necessarily represent those of their affiliated organizations, or those of the publisher, the editors and the reviewers. Any product that may be evaluated in this article or claim that may be made by its manufacturer is not guaranteed or endorsed by the publisher.
Research integrity at Frontiers
Learn more about the work of our research integrity team to safeguard the quality of each article we publish.