- 1Environmental and Sustainability Studies Graduate Program, The University of Charleston, College of Charleston, Charleston, SC, United States
- 2Department of Biology, College of Charleston, Charleston, SC, United States
- 3Department of Geology and Environmental Geosciences, College of Charleston, Charleston, SC, United States
Introduction: Rapid urbanization in coastal areas worldwide, combined with intense precipitation events and coastal flooding exacerbated by climate change, poses an existential challenge for many coastal communities. Floodwater in coastal urban areas contains non-point source pollutants, such as trace metals and fecal coliforms, but the presence of fecal coliforms resistant to antibiotics poses an additional threat to human health and has yet to be reported.
Methods: In this study, floodwater samples were collected from four locations in Charleston, SC, a medium-sized coastal city in the southeastern United States. All sites were impacted by flooding: two by tidal and rainfall flooding, one by tidal flooding, and one by stormwater runoff. Since ampicillin is a commonly-prescribed antibiotic for both humans and animals, this study aimed to analyze the patterns of ampicillin-resistant coliform (AmpRC) concentrations as correlated to flood-source and land-use patterns.
Results: Floodwater from all areas contained AmpRC and trace metals, with varying contaminant concentrations based on the flood source. No correlations were observed between coliform and trace metal concentrations. Analysis of land-use patterns demonstrated a positive correlation between percent coverage of impervious surfaces and coliform concentrations at all the sites.
Discussion: Overall, the results suggest that land-use patterns increase the prevalence of antibiotic-resistant coliforms and increase the likelihood of human exposure to these potential pathogens. Climate change is expected to exacerbate the presence of antibiotic-resistant bacteria in floodwater generated from rainfall and tidal flooding in coastal cities. Cities like Charleston, SC are experiencing rapid urbanization and increased coastal flooding, making this research particularly relevant.
Introduction
A global increase in urbanization has led to a rise in population living in flood-prone areas. In 2015, 11.3% of the population lived in such areas (Neumann et al., 2015; Rentschler et al., 2023). Increased urbanization is of particular interest in the United States because of the increased migration of people from rural areas to cities, resulting in the redevelopment of more than 40 million hectares of land from 1978 to 2012 (Bounoua et al., 2018; USDA, 2019). This increase in urbanization accounts for 53% of the US population, particularly in coastal areas, with 40% of these individuals relocating to the coast (National Weather Service and NOAA, 2024; Office for Coastal Management and NOAA, 2024). This increase in urbanization can harm the environment and human health (Adams et al., 2014; Kulp and Strauss, 2019; Tellman et al., 2021).
Increased urbanization increases impervious land surface, leading to increased flooding (Khan, 2005; O'Driscoll et al., 2010; Zhang et al., 2018). Flooding from either heavy rainfall or high tides can occur, and in some instances, compound flooding may occur when both co-occur (Hendry et al., 2019; Salarieh et al., 2023). During heavy rainfall or high tide, gutters become overwhelmed and block water from leaving the streets through stormwater drains, which usually discharge water into nearby waterbodies (Despotovic et al., 2005; Qin, 2020). Floodwaters contain different nonpoint source pollutants, particularly coliforms and trace metals of concern (Petrucci et al., 2014; Chithra et al., 2015). These coliforms in natural waters can be resistant to antibiotics, such as ampicillin, typically due to wastewater discharge into streams (Niemi et al., 1983; Akiyama and Savin, 2010; Allen et al., 2010; Rizzo et al., 2013). These nonpoint source pollutants have a deleterious impact and are entering the waterbodies, negatively impacting marine and aquatic ecosystems (McCoy et al., 2015). The transfer of pollutants from runoff into coastal streams is exacerbated by increased flooding caused by rising sea levels and coastal inundation (Blair et al., 2014b; Spanger-Siegfried et al., 2014). In addition to rising sea levels, increasing frequency of storm surges and off-shore storms can create frequent higher-than-normal tides (Jongman et al., 2012; Sweet and Park, 2014). According to the National Oceanic and Atmospheric Administration (NOAA), by 2050, there will be an average of 45–85 days of high-tide flooding events across the US (Silverstein and NOAA, 2023). Runoff-related pollution is expected to be exacerbated by the increased frequency of compound flooding in coastal areas (Bevacqua et al., 2020; Ghanbari et al., 2021; Yang et al., 2021).
Flooding is also caused by intense rainfall associated with climate change, and rain across the United States has increased by an average of 13% since 1970 (Climate Central, 2023). In the southeastern United States, there is an annual precipitation of 1,260–1,530 mm, consisting mostly of liquid precipitation, with higher intensity in warmer seasons (Qian et al., 2021). Short-duration high-intensity rainfall has become more frequent in the United States and globally since 1950 (Degaetano, 2009; Skeeter et al., 2019) and the resulting coastal flooding is exacerbated by sea level rise (Ekmekcioglu et al., 2022). Compound flooding occurs when tidal and rainfall flooding co-occur, exacerbating flooding and causing impassable streets (Coz et al., 2021). There are typically two routes for transferring nonpoint source pollution from the urban environment to the waterways: stormwater discharge and tidal flooding.
Coliforms and other bacteria are commonly found in the natural environment and floodwaters. Escherichia coli is widely used as an indicator organism for identifying the presence of possible fecal contamination in water (Edberg et al., 2000; Odonkor and Ampofo, 2013). Antibiotics, such as ampicillin, as prescribed to humans, livestock, and pets to treat bacterial infections, are used for inhibiting the growth of bacteria and can either quickly degrade or persist for long periods in the environment (Bhattacharjee, 2016; Scott et al., 2016). Antibiotic resistance, or the decrease in the effectiveness of a specific antibiotic against bacteria, has become an urgent issue in public health and environmental biology (Manaia et al., 2012; Murray et al., 2022; Velazquez-Meza et al., 2022). Approximately 15 million deaths were attributed to antimicrobial resistance in 2019 (Murray et al., 2022), making antimicrobial resistance a leading cause of death globally. Furthermore, antibiotic-resistant infections can lead to limited pharmaceutical treatment, extended hospital stays, and increased financial burdens (Cosgrove, 2006).
Trace metals are also found in floodwater environments in trace amounts and can be toxic to aquatic organisms depending on the concentration and uptake rates (Bezerra et al., 2019). At high concentrations, these metals are toxic to environmental bacteria, but at lower doses, the bacteria can adapt and become resistant to those metals during chronic exposure (Biswas et al., 2021). Along with developing resistance to metals, the bacteria can become antibiotic-resistant simultaneously due to continuous stress tolerance by efflux pumping through the bacterial cell membrane (Wright et al., 2006; Biswas et al., 2021).
During urban flooding events, people often wade through the waters, unaware of the potential risk of bacterial infection (Ashley and Ashley, 2008). Exposure to coliforms by ingestion of contaminated water can cause many types of infections and diseases (Rock and Rivera, 2014; Perkins and Trimmier, 2017; Holcomb and Stewart, 2020). In 2019, there were at least 2.8 million microbial-resistant infections in the United States, with more than 35,000 mortalities, and these numbers will continue to increase if preventative measures are not utilized (CDC, 2019). When chronically exposed to antibiotics, coliforms can become antibiotic-resistant (Harwood et al., 2000). Antibiotic-resistant coliforms (ARC) will continually evolve and develop resistance to multiple antibiotics (Hamad et al., 2019), making this a long-term health issue (Domingues et al., 2021).
The purpose of this study was to evaluate urban floodwater quality in a rapidly growing coastal city in the southeastern US. Specifically, our objective was to quantify the prevalence of ampicillin-resistant coliforms (AmpRC) and to identify any relationships with other water quality parameters. We had three hypotheses: (i) the coliforms present in the floodwaters are resistant to ampicillin, (ii) there is a positive correlation between AmpRC and trace metal concentrations in the floodwater samples, and (iii) the growth of AmpRC is affected by water quality parameters such as pH and water temperature. To evaluate these hypotheses, floodwater samples were collected at sites that experience localized flooding due to heavy rainfall, tides, or compound flooding. Factors such as automobile and pedestrian traffic patterns were also considered for our choice of sampling sites. For this study, the term “floodwaters” encompasses tidal, rainfall, or compound flooding events.
Materials and methods
Study area
Charleston is located on the South Carolina coast and sits on a peninsula with the adjacent Ashley River to the west and Cooper River to the east (Figure 1). Charleston Harbor is south of the peninsula and acts as an estuarine buffer between the rivers and the Atlantic Ocean (Purcell et al., 2020). The Charleston peninsula had undergone extensive urban development, which involved filling or draining marshes and other natural areas (Butler, 2020), increasing the volume of stormwater runoff draining into the coastal waterways (Blair et al., 2014a).
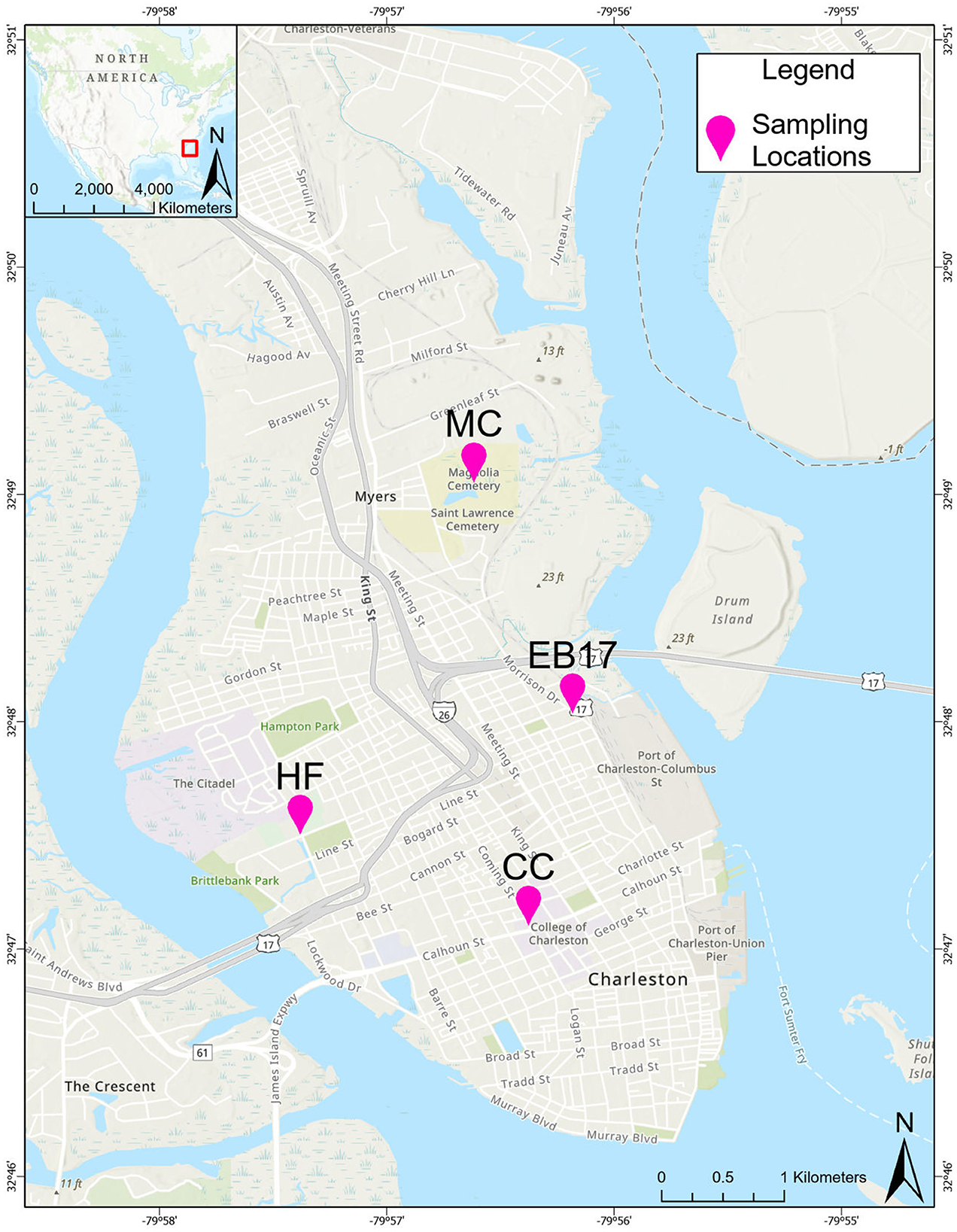
Figure 1. The floodwater sampling sites on the Charleston Peninsula are shown. The HF site is at the intersection of Hagood Ave. and Fishburne St.; the CC site is at the intersection of Calhoun St. and Coming St.; the EB 17 site is near the junction of East Bay St. and HWY 17; and the MC site is in Magnolia Cemetery.
Floodwater was sampled within the Charleston peninsula at four sites: (i) Hagood Ave. at Fishburne St. (HF) (32°47′30.0′′N 79°57′22.9′′ W), (ii) Coming St. at Calhoun St. (CC) (32°47′6.0′′N 79°56′22.6′′ W), (iii) East Bay St. at HWY 17 (EB17) (32°48′01.9′′N 79°56′10.9′′ W), and (iv) Magnolia Cemetery (MC) (32°49′3.3′′N, 79°56′37.0′′ W). The MC site is a tidal creek next to a historic cemetery and was designated as the control site as this site did not receive stormwater runoff and was only impacted by tidal flow from the Cooper River (Figure 1). These sites were chosen based on the updated flood hazard data from the US Federal Emergency Management Agency (FEMA) Flood Hazard and Risk Data Viewer (FEMA, 2020). The viewer provided a flood zone map of where potential areas in Charleston would be inundated by a flood event (FEMA, 2020). The MC, HF, and EB-17 sites experience sunny-day tidal flooding where the flood tide height is at least 2.1 m MLLW (Coz et al., 2021). Our observations during sampling confirmed commonly flooded sites during stormwater and tidal flooding.
Floodwater sampling methodology
Floodwater sampling was conducted from December 2021 to December 2022 as outlined: (i) the MC site from March 2022 to September 2022, (ii) the HF site from December 2021 to July 2022, (iii) the EB17 site during June 2022, July 2022, and December 2022, and (iv) the CC site from June 2022 and December 2022. At all sites, floodwater samples were collected near stormwater drains or culverts. The CC site was only sampled during rainfall events due to the site being farther inland and not impacted by tidal flooding. The HF and EB17 sites were sampled during both rainfall and high tide flooding events due to the proximity of the Ashley River and Cooper River, respectively.
When at least 1 cm depth of water was flowing into the drain, a floodwater sample was collected with either water flowing into the mouth of a sampling container or completely submerging the container while tightening the cap. Water samples for trace metal and coliform analyses were collected using 50 mL sterile centrifuge tubes and stored in a cooler for transport. Bright vests were worn during sample collection for visibility and personal protection and sample contamination was minimized. Measures were taken to prevent bed sediment collection and minimize turbid conditions by gently sampling water from the stormwater drain. This method is based on the United States Environmental Protection Agency (USEPA) standard method for industrial stormwater sampling (USEPA, 2021).
Tidal height and precipitation data were obtained from the NOAA online portals (Center for Operational Oceanographic Products and Services and NOAA, 2023; National Weather Service and NOAA, 2024). Tide data from the tide gauge station closest to the sampling site was used to record the most representative tide height.
Watershed delineations and impervious surface coverage
Watershed delineations were created to identify the boundaries surrounding the floodwater sites where stormwater runoff would flow toward lower elevations. ArcGIS Pro (version 3.1, ESRI, Redlands, CA) and the built-in Hydrology Tools were used to perform watershed delineations. A 1-m resolution digital elevation model (DEM) created using the available Light Detection and Ranging (LiDAR) data was used to determine the elevation contours of the peninsula. The errors in the DEM were corrected by using the Fill tool in the Hydrology Toolset to allow for a better representation of the flow direction (Arifjanov et al., 2022). Streamlines were generated after the filling of the sinks to determine where the stormwater runoff would flow and if the water would flow toward the floodwater sites by using the Flow Direction tool. Finally, a watershed boundary for all the streamlines was created for each sampling site by converting the raster to a polygon (Villines et al., 2015; Goji Tumba and Amusuk, 2017). The polygons at each site were all spatially joined to make all the watersheds into one layer. The impervious surface layer was obtained from the United States Geological Survey (USGS) National Land Cover Database (NLCD) (USGS, 2021) which provides impervious surface percentages for the Charleston peninsula in 2019. This layer was joined with the watershed boundaries layer.
Bulk water quality measurements
The Manta+20 Eureka™ Water Probe (Eureka™ Water Probes, Austin, TX) was used to collect water quality parameters, including water temperature (°C), pH, conductivity (μS/cm), and dissolved oxygen (mg/L). After all the readings for each parameter remained stable, measurements were recorded, and the probe was rinsed with deionized water (18 MΩ.cm) before and after measurements to prevent cross-contamination. Conductivity measurements obtained from the Water Quality Data Portal for Ashley River and Cooper River were averaged from 1981 to 2021 (National Water Quality Monitoring Council, 2023). The mean conductivity values for the Ashley River and Cooper River are 31,745 μS/cm and 30,867 μS/cm, respectively. The conductivities from the sampling sites that had tidal influences were compared to the corresponding river conductivity value to identify if the floodwater was brackish.
Bacterial enumeration
Bacterial analysis began within 4 h of collection to enumerate coliforms and ampicillin-resistant coliforms. All bacteria culture plates were filled with 1 mL sample volume. This was either achieved with sterile water, ampicillin solution, or a mix of site water with sterile water or ampicillin solution depending on the site. For the MC site, the Compact Dry EC plates (Hardy Diagnostics, Santa Maria, CA) were rehydrated with either ampicillin (32 mg/L) or sterile water for the membrane filtration using a sterile 0.45 μm filter of floodwaters since this site had lower bacterial concentrations (APHA et al., 2018). The Compact Dry EC plates contain chromogenic enzyme substrates, so E. coli will form blue colonies whereas other coliforms will turn pink-purple (Kodaka et al., 2006). For the other three sampling sites (HF, EB17, and CC), floodwater samples were diluted with either sterilized water or a solution of ampicillin directly applied to the plate. Plates were incubated at 35°C ± 2°C, and after 18–24 h, the colony-forming units (CFUs) were enumerated. One CFU equals one bacterium, therefore, a high number of bacteria in the floodwaters would result in high CFUs. E. coli and coliforms were enumerated to yield total coliform (TC) count and reported in CFU/100 mL. If the CFUs counted on the plates prepared with a sample were >150 CFUs, that sample was coded as Too Numerous To Count or TNTC (SC DHEC, 2019). If a 150-CFU threshold was reached for a sample, a value of 150 CFU/100 mL was reported as a conservative estimate of TC coliform concentration.
Trace metals analysis
Total concentrations of dissolved trace metals (Al, Sb, As, Ba, Be, Cd, Cr, Co, Cu, Pb, Mn, Mo, Ni, Se, Ag, Tl, Th, U, V, and Zn) in the water samples were determined using an inductively coupled plasma mass spectrometer (ICP-MS) (iCAP RQ ICP-MS, Thermo Fisher Scientific, Waltham, MA) based on the revised USEPA Method 200.2 Revision 2.8 (Martin et al., 1994). The water samples were filtered using 0.45 μm polyethersulfone (PES) syringe filters and acidified to 2% v/v using nitric acid. A multi-element standard mix (High Purity Standards, North Charleston, SC) was diluted to calibrate the inductively coupled plasma mass spectrometer (ICP-MS) consisting of serial dilutions from 10−4 to 10 mg/L. Check standards and blanks were incorporated for each set of water samples.
Statistical analyses
The raw data were organized, and outliers were removed for the average colony concentrations for E. coli, coliforms, and TC by using the interquartile range and calculating the inner and outer fences to identify the boundaries of the data (Schwertman and de Silva, 2007). After removing the outliers (63% of total samples removed), principal component analysis (PCA) was performed on the data using R software. The normalized data from all the experimental variables were transformed into principal components (PCs), which are linear projections that are arranged by the size of the eigenvalue. This analysis reduced the dimensionality and identified any relationships between multiple variables (Demšar et al., 2013). Additional R packages, including factoextra, were used to generate biplots (Kassambara and Mundt, 2020), ggplot2 was used to make plots aesthetically appealing (Wickham, 2016), and psych was used to calculate the cumulative variance (Revelle, 2023).
Results
Watershed delineation of sampling sites
The four sampling sites were located on different parts of an urban peninsula, which had varying percentages of impervious surfaces. The MC site had the least impervious coverage on the peninsula, ranging between 1% and 19%. The CC site had the highest impervious coverage, ranging from 80% to 100%. The HF and EB17 sites had similar impervious coverages, between 50% and 79%.
The HF site had the smallest watershed boundary, which was only 3.23 hectares. The EB17 site, on the other hand, had the largest watershed boundary of 55.04 hectares. Both these sites were affected by compound flooding. The MC site had the second-largest watershed boundary of 19.02 hectares and was mainly affected by tidal flooding. Finally, the CC site watershed boundary was 10.52 hectares and experienced only rainfall flooding (Figure 2).
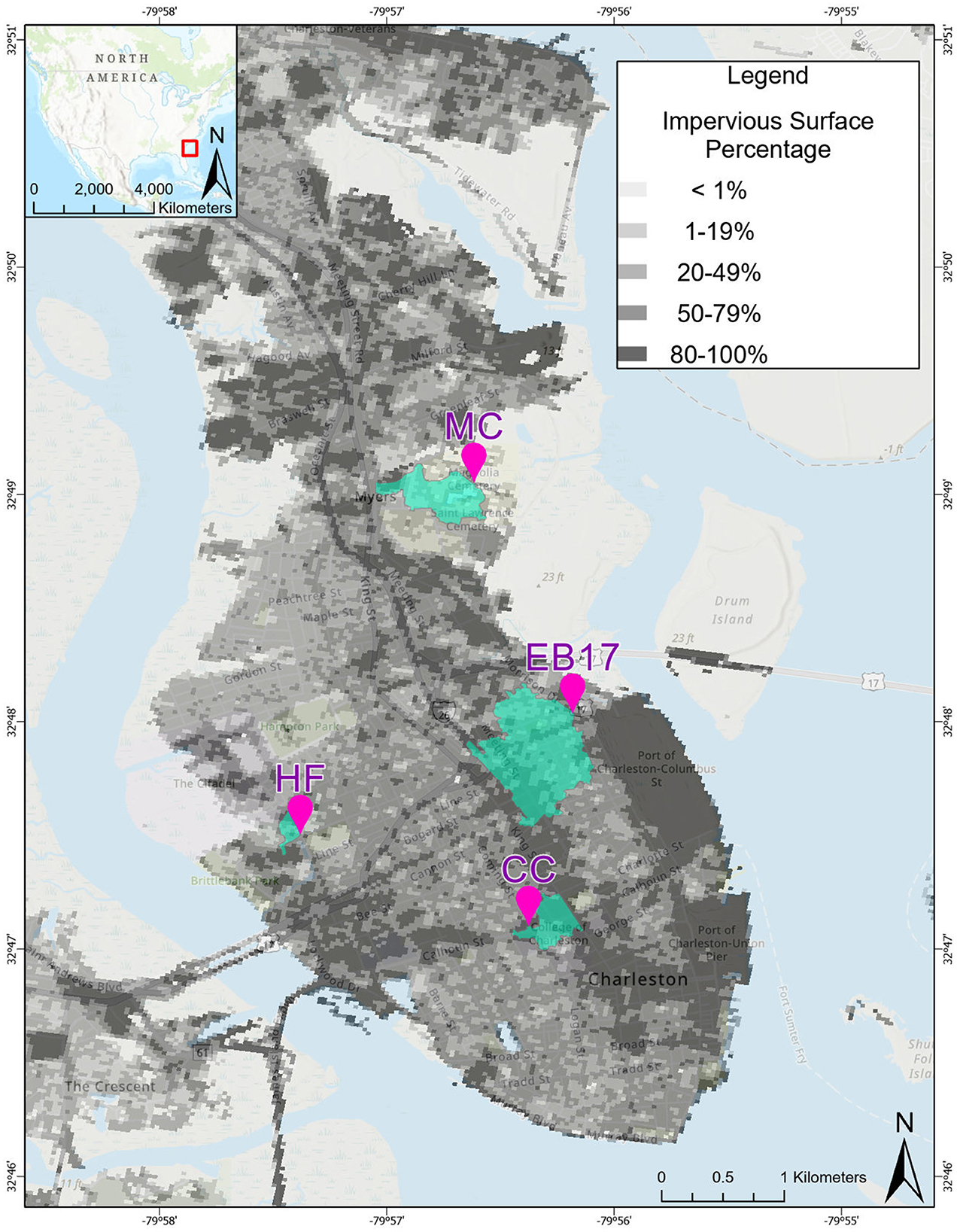
Figure 2. Watershed delineation was conducted using the USGS National Land Cover Database (NLCD) layer. The impervious surface coverage is shown as a percentage of total land coverage. The stormwater runoff watershed boundaries for each sampling site are overlain on top of the impervious surface layer.
Contaminants in floodwater
Chemical characterization was performed independently for each site due to their unique geography and flooding influences. The medians, first and third quartiles, were used because these water quality data are not normally distributed. The conductivity data indicated that the MC site had brackish water in the floodwater with a median conductivity of 27,130 μS/cm. The median tide height at this site was 1.32 m MLLW, and no rainfall was recorded at this site during the sampling events. The median TC and AmpRC colony concentrations were 4,259 CFU/100 mL and 764 CFU/100 mL, respectively, or AmpRC counts were 17.93% of TC counts for this site. Of trace metals, only As was detected at this site with a median value of 4 μg/L (Table 1).
The CC site was only influenced by stormwater runoff, and the stormwater had a median conductivity of 100 μS/cm and a median rainfall of 1.82 cm during the sampling events at this site. The median TC and AmpRC colony concentrations were 33,850 CFU/100 mL and 259 CFU/100 mL, respectively, or the AmpRC counts were 0.76% of TC counts at this site. Both As and Cr were detected in the floodwater at this site with median concentrations of 3 μg/L and 5 μg/L, respectively (Table 1).
The median conductivity of 6,340 μS/cm confirmed that HF site was impacted by compound flooding (typical conductivity maximum values for freshwater and saltwater are 700 μS/cm and 25,000 μS/cm, respectively). The median rainfall amount and tide height at this site during sampling events were 1.75 cm and 1.32 m MLLW, respectively. The median TC and AmpRC colony concentrations were 12,300 CFU/100 mL and 6,334 CFU/100 mL, respectively or the AmpRC counts were 51.49% of TC counts at this site. Only As was detected at this site with a median concentration of 3 μg/L (Table 1).
The EB17 site was also impacted by compound flooding like the HF site, and the floodwater had a median conductivity of 976 μS/cm, which is above the threshold of freshwater conductivity of 700 μS/cm. The median rainfall amount and tide height at this site during sampling events were 0.97 cm and 1.32 m MLLW, respectively. The median TC and AmpRC colony concentrations were 49,500 CFU/100 mL and 9,334 CFU/100 mL, respectively or the AmpRC counts were 18.86% of TC counts at this site. Trace metals, As and Cr, were detected in the floodwater with median concentrations of 4 μg/L and 8 μg/L, respectively (Table 1).
Statistical analysis
Principal component analysis (PCA) was used to correlate the physical, chemical, and microbial contaminant data from all the sites. Eight principal components (PCs) comprising eight variables (contaminant data), including TC, AmpRC, tide height, rainfall, conductivity, As, Cr, and Zn, were calculated. The proportion of variances for each PC were 33%, 21%, 14%, 11%, 9%, 7%, 3%, and 2%, respectively. The first two PCs (PC1 and PC2) were used for the biplots as they accounted for the two highest variances (33.1% and 20.6%, respectively). Rainfall, TC, and AmpRC were strongly and positively correlated with each other. The tide height and conductivity are strongly correlated to each other and negatively correlated with rainfall and TC, and less strongly correlated with AmpRC counts. The trace metals all were strongly correlated with each other and less correlated with TC and AmpRC concentrations (Figure 3).
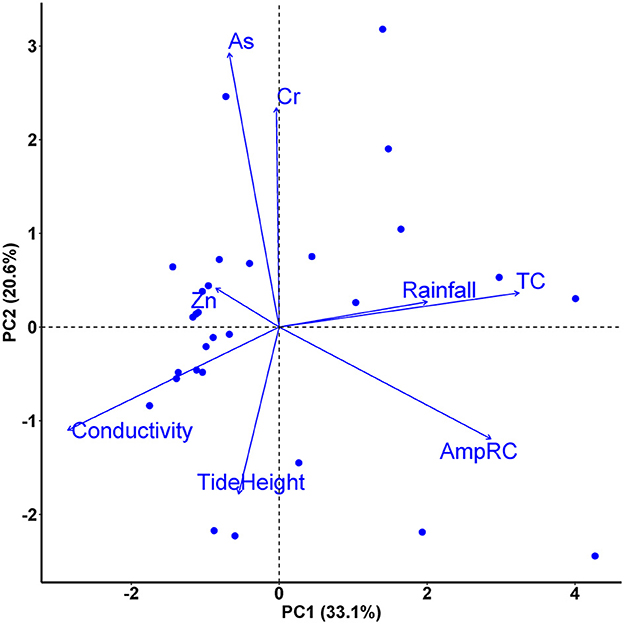
Figure 3. The principal component analysis biplot. The biplot has individual observations (dots) and variables (arrows) for PC1 and PC2. The variables include AmpRC, TC, tide height, rainfall amount, conductivity, and three trace metals (As, Cr, and Zn).
Discussion
This study showed that AmpRC was present in floodwaters from rainfall and tidal events in Charleston under all rainfall or tidal conditions and in all locations. The impervious surfaces and compound flooding impacted both the TC and AmpRC concentrations. Trace metals were also present in the floodwaters, but no co-resistance with AmpRC was established from data analysis.
Impact of precipitation and high tide events
The coliforms (TC) and AmpRC observed in the floodwaters in this study can originate from many sources, as observed in similar coastal areas (Selvakumar and Borst, 2006). The detection of AmpRC in the nearby tidal creeks, Shem Creek and Kushiwah Creek (DeLorenzo et al., 2012; Emery et al., 2022), also confirms that one of the main sources of AmpRC in coastal waterways is the floodwater generated within the urban watersheds. Other cities in the United States have found coliforms and antibiotic-resistant bacteria (ARB) in different urban waterbodies from stormwater discharge (Singh et al., 2019) and antibiotic-resistant microbes, an emerging public health threat (Scott et al., 2016). These bacteria are discharged directly into the waterbodies via stormwater drains, negatively affecting the marine ecosystem and human health (Parker et al., 2010). In addition, there is the potential for antibiotic-resistant genes, such as ampicillin-resistant genes, to be shared among different microbes (Uyaguari et al., 2011, 2013; Horton, 2022) such as Vibrio spp. which are highly prevalent and known human health threats to seafood safety and contact recreation. The mechanism for resistance to ampicillin is typically due to β-lactamase activity. Genes for this enzyme are typically encoded on mobile genetic elements allowing for ease of horizontal gene transfer to other environmental bacteria, which can impact human health (Uyaguari et al., 2011, 2013). Increased surveillance of AmpRC as well as these genetic elements would allow for targeted interventions to prevent environmentally acquired antibiotic resistance infection (Djordjevic et al., 2023).
Recent studies of urban floodwaters in the aftermath of tropical cyclones have shown an increased presence of contaminants, including coliforms and antibiotic genes, which exacerbates potential AmpRC presence in floodwaters (Presley et al., 2005; Yang et al., 2021). The possible sources for the transfer of coliforms during storms and sunny-day flooding include potential septage leaks from aging sewer lines, dog waste, and wildlife (Whitlock et al., 2002; DeLorenzo et al., 2012; Almakki et al., 2019; Powers et al., 2020). The AmpRC analytical method used in this study may be used to identify pollution sources from pets, as ampicillin is often prescribed for skin disorders in dogs and cats. Elevated levels of ampicillin-resistant E. coli were found at sites with high concentrations of pets, such as urban dog parks (Kelsey et al., 2003). In this study, the HF site had nearly 51.5% of the TC in the form of AmpRC bacteria (or, AmpRC/TC ratio was 51.5%) and is near a city park frequented by pets. The AmpRC/TC ratio was much lower at the other sites in this study, ranging from 7.6%−18.9%.
The rise in coastal sea level is exacerbating the flooding for many communities along the coasts across the world, including the United States. The global average sea level in 2022 was 101.2 mm higher than the 1993 level, with high tide flooding across the United States being over 300–900% more frequent than it was 50 years ago (Lindsey, 2022). The increased impervious surfaces in coastal cities, coastal erosion, groundwater pumping, and subsidence exacerbate flooding and, thus, increase pollution of floodwater and coastal waterways (Chithra et al., 2015; Lindsey, 2022; Ohenhen et al., 2023). Climate change has been increasing the number of storms, including short-duration storms and major storms like hurricanes (Lau et al., 2022), which can also coincide with high tides as more intense compound flooding (Bevacqua et al., 2020).
The conductivity was used as an indicator to identify if the floodwaters originated from freshwater sources (rainfall) or brackish (tidal) sources. For freshwater, the conductivity that was <700 μS/cm was typically associated with rainfall. Brackish water typically has a range between 700–25,000 μS/cm, which would come from tidal flooding and not directly from the ocean (saltwater) (Rusydi, 2018).
Impact of impervious surfaces
The USGS's land coverage (NLCD raster) data from 2019 was used to represent the changing landscape of Charleston (USGS, 2021). Combining these land cover data with high-resolution (1-m) ground elevation (LiDAR), data allowed us to closely examine the impact of impervious surfaces on the contamination of floodwater. The percentage of impervious surfaces showed urbanization surrounding the study sites correlated inversely with the coliform data. On average, the MC site had fewer coliforms than the other three study sites which had more impervious surfaces. This high-resolution LiDAR watershed delineation identified where stormwater can accumulate and flow toward each of our sampling sites but did not directly address tidal flooding. Tidal conditions impacted how quickly the storm drains were emptied or if they backed up—these aspects were not addressed in our watershed delineation analysis and would need to be addressed in the future.
The region continues to experience population growth, according to the 2020 Decennial Census (US Census Bureau, 2023). This urbanization within the greater Charleston region is expected to increase between 2% and 30% by 2030, with urban sprawl continuing to impact the region (Dickes et al., 2016). Rising sea levels in the coastal areas, increasingly intense precipitation, and the associated rise of the water table increase concerns about the loss of floodwater's ability to seep into the ground (Sweet and Park, 2014; Neumann et al., 2015; Kulp and Strauss, 2019). Loss of permeable ground surfaces increases local flooding and the transport of floodwater-associated contaminants into coastal waterbodies. The HF and MC sites have higher vegetation buffers and less impervious surfaces than the other two sites, which allowed increased seeping of floodwater into the ground instead of localized flooding to some extent. A more systematic study would be required to confirm this observation. The HF and MC sites also had lower TC and AmpRC colony concentrations than the CC and EB17 sites. Studies reported increased fecal coliform and trace metal concentrations in stormwater after an increase in impervious surfaces in urban areas (Bhandari et al., 2017; Zhang et al., 2021). These studies confirm a positive correlation between the increased presence of E. coli in stormwater and increasing urbanization.
Trace metals in floodwater
Trace metals are commonly associated with urban runoff under a variety of conditions—during normal or extreme precipitation events (Joshi and Balasubramanian, 2010; Barber et al., 2017; Bhandari et al., 2017; Kirker and Vulava, 2022). There are no guidelines or regulations that inform safe levels of trace metals in urban floodwater. Still, there are the USEPA's published guidelines, such as the “National Recommended Water Quality Criteria-Aquatic Life Criteria Table” for acceptable concentrations of water contaminants (USEPA, 2023). Typically, floodwater and urban runoff can be more concentrated compared to the final receiving waterbodies, where contaminants are diluted (Joshi and Balasubramanian, 2010). Three trace metals (As, Cr, and Zn) were detected in the floodwater during this study and none of these trace metals approached levels that would be deemed concerning from aquatic life criteria (USEPA, 2023). We note that all floodwater samples in our study were filtered and analyzed—however, there was a significant suspended sediment load in most floodwater samples. Analysis of composited water samples would most likely have shown a significantly higher concentration of trace metals in the runoff (Jeong et al., 2020). Charleston region has abundant marine-derived phosphate rock deposits (Malde, 1959) and a history of phosphate mining, resulting in elevated arsenic in regional waterbodies of this area and the floodwater in our study. Arsenic pollution in urban watersheds and the estuaries of the southeastern US and many parts of the world is primarily from the leaching of As from phosphate rocks and industrial activities (Chirenje et al., 2003; Cotti-Rausch et al., 2018). Similarly, Cr and Zn in urban floodwaters can be associated with a combination of rock weathering, industrial activities, automobile wear and tear, etc. (Joshi and Balasubramanian, 2010; Cotti-Rausch et al., 2018).
Contaminant interrelationships
Identifying relationships between different water quality data has given insight into the interaction between the variables. Trace metals as stressors on ARB or the possibility of co-resistance between the two have been frequently studied (Hamilton et al., 2020; Biswas et al., 2021; Horton, 2022), however, further studies are needed in the Charleston region. The relationships between rainfall amounts and tide height with coliforms (TC and AmpRC) are well-known, especially in the urban environment (Liu and Huang, 2012; Kirker and Vulava, 2022). High trace metal concentrations may be toxic to Vibrio and Enterococcus, while low chronic doses stimulate growth and possibly induce antibiotic resistance (Horton, 2022).
The bacteria concentrations at all study sites affirmed the presence of AmpRC in the urban floodwaters of Charleston. Additional studies would be required to identify these bacteria source(s). Coliforms resistant to other common antibiotics may be present in these floodwaters—an analytical method designed to identify the resistance to multiple antibiotics would provide more insights. Multidrug-resistant coliforms have been isolated from a surrounding waterway (Emery et al., 2022). Future assessments of urban floodwaters should assess the prevalence of coliforms resistant to multiple commonly human and animal-prescribed antibiotics.
The trace metals measured in this study did not positively correlate with TC or AmpRC concentrations, but other trace metals that are likely to be associated with suspended sediment may correlate with both types of bacteria. An extended sampling period and additional sampling sites would allow more comprehensive data. Comparisons between trace metal concentrations in tidal creeks and stormwater ponds within the tidal creek watersheds in coastal South Carolina confirmed the presence of Cd, Cu, Cr, and Zn (Sanger et al., 1999; Allen et al., 2019; Horton, 2022).
The PCA method allowed for the simplification of complex variables while still retaining trends (Lever et al., 2017), but using other statistical methods would provide more insight into the relationships with other variables from this study.
While flooding on the Charleston peninsula continues in a changing climate, the utilization of best management practices (BMPs) for the stormwater infrastructure would mitigate contamination of water bodies. For example, bioretention landscapes or rain gardens that include vegetation, filtration media, and drains can retain and filter stormwater (Li et al., 2019). Many people in Charleston are frequently exposed to these contaminated floodwaters. Installing BMPs may alleviate the exposure to contamination and help lower the microbial load in the floodwaters (Ahmed et al., 2019).
To decrease human exposure to these antibiotic-resistant potential pathogens, members of communities with high levels of flooding should be informed. Active public educational outreach about contaminants present in the floodwaters will contribute significantly to positive health outcomes (Meinhardt, 2006; MacDonald and Tippett, 2020). For example, the development of a short one-page fact sheet warning the public of the bacterial and other pollution hazards posed by floodwater would alert the public of exposure. The USEPA uses such infographics to warn the public about pollutants, such as Pb found in drinking water (USEPA, 2017).
Conclusion
TC and AmpRC were present in the floodwaters of peninsular Charleston during tidal, rainfall, and compound flooding events. Impervious surfaces exacerbated flooding on the Charleston peninsula and increased TC and AmpRC concentrations, which were positively correlated with each other and with rainfall. However, no correlation was observed with trace metals in the floodwaters, and there was a negative correlation with the conductivity of the floodwaters. The population of Charleston is continuing to grow with more infrastructure and impervious surfaces, likely leading to more pollution. High concentrations of bacteria (TC and AmpRC), trace metals, and other contaminants (e.g., organic compounds) in floodwaters pose a public health risk for the many residents and visitors to the city. Flooding is an increasingly common occurrence in coastal areas due to climate change, and many people are unaware of the potential health risks associated with exposure to floodwater. BMPs should be utilized to improve the stormwater infrastructure to alleviate flooding, and the public should be made aware of the health risks associated with exposure to these floodwaters.
Data availability statement
The original contributions presented in the study are included in the article/supplementary material, further inquiries can be directed to the corresponding author.
Author contributions
KS: Data curation, Formal analysis, Investigation, Methodology, Project administration, Software, Validation, Visualization, Writing—original draft, Writing—review & editing. HF: Conceptualization, Data curation, Formal analysis, Funding acquisition, Methodology, Project administration, Resources, Software, Supervision, Validation, Visualization, Writing—review & editing. VV: Conceptualization, Data curation, Formal analysis, Funding acquisition, Methodology, Project administration, Resources, Software, Supervision, Validation, Visualization, Writing—review & editing.
Funding
The author(s) declare that financial support was received for the research, authorship, and/or publication of this article. The project was funded internally by the School of Sciences and Mathematics, the Graduate School, the M.S. in Environmental and Sustainability Student Association (MESSA), and the Undergraduate Research Program.
Conflict of interest
The authors declare that the research was conducted in the absence of any commercial or financial relationships that could be construed as a potential conflict of interest.
Publisher's note
All claims expressed in this article are solely those of the authors and do not necessarily represent those of their affiliated organizations, or those of the publisher, the editors and the reviewers. Any product that may be evaluated in this article, or claim that may be made by its manufacturer, is not guaranteed or endorsed by the publisher.
References
Adams, J., Speakman, T., Zolman, E., Mitchum, G., Wirth, E., Bossart, G. D., et al. (2014). The relationship between land use and emerging and legacy contaminants in an Apex predator, the bottlenose dolphin (Tursiops truncatus), from two adjacent estuarine watersheds. Environ. Res. 135, 346–353. doi: 10.1016/j.envres.2014.08.037
Ahmed, W., Hamilton, K., Toze, S., Cook, S., and Page, D. (2019). A review on microbial contaminants in stormwater runoff and outfalls: potential health risks and mitigation strategies. Sci. Total Environ. 692, 1304–1321. doi: 10.1016/j.scitotenv.2019.07.055
Akiyama, T., and Savin, M. C. (2010). Populations of antibiotic-resistant coliform bacteria change rapidly in a wastewater effluent dominated stream. Sci. Total Environ. 408, 6192–6201. doi: 10.1016/j.scitotenv.2010.08.055
Allen, H. K., Donato, J., Wang, H. H., Cloud-Hansen, K. A., Davies, J., and Handelsman, J. (2010). Call of the wild: antibiotic resistance genes in natural environments. Nat. Rev. Microbiol. 8, 251–259. doi: 10.1038/nrmicro2312
Allen, T. R., Crawford, T., Montz, B., Whitehead, J., Lovelace, S., Hanks, A. D., et al. (2019). Linking water infrastructure, public health, and sea level rise: integrated assessment of flood resilience in coastal cities. Public Works Manage. 24, 110–139. doi: 10.1177/1087724X18798380
Almakki, A., Jumas-Bilak, E., Marchandin, H., and Licznar-Fajardo, P. (2019). Antibiotic resistance in urban runoff. Sci. Total Environ. 667, 64–76. doi: 10.1016/j.scitotenv.2019.02.183
APHA, AWWA, and WEF. (2018). “9222 membrane filter technique for members of the coliform group,” in Standard Methods For the Examination of Water and Wastewater, eds. W. C. Lipps, T. E. Baxter, and E. Braun-Howland (Washington, DC: APHA Press).
Arifjanov, A., Atakulov, D., Akhmedov, I., and Hoshimov, A. (2022). “Modern technologies in the study of processes in channels,” in IOP Conference Series: Earth and Environmental Science, 012137. doi: 10.1088/1755-1315/1112/1/012137
Ashley, S. T., and Ashley, W. S. (2008). Flood fatalities in the United States. J. Appl. Meteorol. Climatol. 47, 805–818. doi: 10.1175/2007JAMC1611.1
Barber, L. B., Paschke, S. S., Battaglin, W. A., Douville, C., Fitzgerald, K. C., Keefe, S. H., et al. (2017). Effects of an extreme flood on trace elements in river water - from urban stream to major river basin. Environ. Sci. Technol. 51, 10344–10356. doi: 10.1021/acs.est.7b01767
Bevacqua, E., Vousdoukas, M. I., Zappa, G., Hodges, K., Shepherd, T. G., Maraun, D., et al. (2020). More meteorological events that drive compound coastal flooding are projected under climate change. Commun. Earth Environ. 1, 1–11. doi: 10.1038/s43247-020-00044-z
Bezerra, M. F., Lacerda, L. D., and Lai, C.-T. (2019). Trace metals and persistent organic pollutants contamination in batoids (Chondrichthyes: Batoidea): a systematic review. Environ. Pollut. 248, 684–695. doi: 10.1016/j.envpol.2019.02.070
Bhandari, S., Maruthi Sridhar, B. B., and Wilson, B. L. (2017). Effect of land cover changes on the sediment and water quality characteristics of brays bayou watershed. Water Air Soil Pollut. 228:336. doi: 10.1007/s11270-017-3538-7
Bhattacharjee, M. K. (2016). Chemistry of Antibiotics and Related Drugs. Cham: Springer, 1–25. doi: 10.1007/978-3-319-40746-3
Biswas, R., Halder, U., Kabiraj, A., Mondal, A., and Bandopadhyay, R. (2021). Overview on the role of heavy metals tolerance on developing antibiotic resistance in both Gram-negative and Gram-positive bacteria. Arch. Microbiol. 203, 2761–2770. doi: 10.1007/s00203-021-02275-w
Blair, A., Lovelace, S., Sanger, D., Holland, A. F., Vandiver, L., and White, S. (2014a). Exploring impacts of development and climate change on stormwater runoff. Hydrol. Proc. 28, 2844–2854. doi: 10.1002/hyp.9840
Blair, A., Sanger, D., White, D., Holland, A. F., Vandiver, L., Bowker, C., et al. (2014b). Quantifying and simulating stormwater runoff in watersheds. Hydrol. Proc. 28, 559–569. doi: 10.1002/hyp.9616
Bounoua, L., Nigro, J., Zhang, P., Thome, K., and Lachir, A. (2018). Mapping urbanization in the United States from 2001 to 2011. Appl. Geogr. 90, 123–133. doi: 10.1016/j.apgeog.2017.12.002
Butler, C. R. (2020). Lowcountry at High Tide: A History of Flooding, Drainage, and Reclamation in Charleston, South Carolina. University of South Carolina Press. doi: 10.2307/j.ctvrxk36b
CDC (2019). 2019 Antibiotic Resistance Threats Report. Available online at: https://www.cdc.gov/drugresistance/biggest-threats.html (accessed August 14, 2023).
Center for Operational Oceanographic Products and Services and NOAA (2023). Tide Predictions - NOAA Tides and Currents. Available online at: https://tidesandcurrents.noaa.gov/noaatidepredictions.html?id=8665530andlegacy=1 (accessed October 11, 2023).
Chirenje, T., Ma, L. Q., Chen, M., and Zillioux, E. J. (2003). Comparison between background concentrations of arsenic in urban and non-urban areas of Florida. Adv. Environ. Res. 8, 137–146. doi: 10.1016/S1093-0191(02)00138-7
Chithra, S. V., Nair, M. V. H., Amarnath, A., and Anjana, N. S. (2015). Impacts of impervious surfaces on the environment. Int. J. Eng. Sci. Invent. 4, 27–31.
Climate Central (2023). Rising Hourly Rainfall Intensity. Available online at: https://www.climatecentral.org/climate-matters/rising-hourly-rainfall-intensity-2023 (accessed October 16, 2023).
Cosgrove, S. E. (2006). The relationship between antimicrobial resistance and patient outcomes: mortality, length of hospital stay, and health care costs. Clin. Infect. Dis. 42, S82–S89. doi: 10.1086/499406
Cotti-Rausch, B. E., Majidzadeh, H., and DeVoe, M. R. (2018). Stormwater ponds in coastal South Carolina - 2018 State of Knowledge Report. Charleston, SC. Available online at: https://www.scseagrant.org/publication/stormwater-ponds-sok/ (accessed August 14, 2023).
Coz, J., Alsheimer, F., and Lindner, B. L. (2021). A climatology-based forecast tool for coastal flooding in the low country. J. Appl. Meteorol. Climatol. 60, 893–908. doi: 10.1175/JAMC-D-20-0256.1
Degaetano, A. T. (2009). Time-dependent changes in extreme-precipitation return-period amounts in the continental United States. J. Appl. Meteorol. Climatol. 48, 2086–2099. doi: 10.1175/2009JAMC2179.1
DeLorenzo, M. E., Thompson, B., Cooper, E., Moore, J., Fulton, M. H., DeLorenzo, M. E., et al. (2012). A long-term monitoring study of chlorophyll, microbial contaminants, and pesticides in a coastal residential stormwater pond and its adjacent tidal creek. Environ. Monit. Assess 184, 343–359. doi: 10.1007/s10661-011-1972-3
Demšar, U., Harris, P., Brunsdon, C., Fotheringham, A. S., and Mcloone, S. (2013). Principal component analysis on spatial data: an overview. Ann. Assoc. Am. Geogr. 103, 106–128. doi: 10.1080/00045608.2012.689236
Despotovic, J., Plavsic, J., Stefanovic, N., and Pavlovic, D. (2005). Inefficiency of storm water inlets as a source of urban floods. Water Sci. Technol. 51, 139–145. doi: 10.2166/wst.2005.0041
Dickes, L. A., Allen, J., Jalowiecka, M., and Buckley, K. (2016). A policy lens of south carolina coastal stormwater management. J. South Carol. Water Resour. 3, 31–41. doi: 10.34068/JSCWR.03.04
Djordjevic, S. P., Jarocki, V. M., Seemann, T., Cummins, M. L., Watt, A. E., Drigo, B., et al. (2023). Genomic surveillance for antimicrobial resistance — a One Health perspective. Nat. Rev. Genet. 25, 142–157. doi: 10.1038/s41576-023-00649-y
Domingues, C. P. F., Rebelo, J. S., Pothier, J., Monteiro, F., Nogueira, T., and Dionisio, F. (2021). The perfect condition for the rising of superbugs: Person-to-person contact and antibiotic use are the key factors responsible for the positive correlation between antibiotic resistance gene diversity and virulence gene diversity in human metagenomes. Antibiotics 10:605. doi: 10.3390/antibiotics10050605
Edberg, S. C., Rice, E. W., Karlin, R. J., and Allen, M. J. (2000). Escherichia coli: the best biological drinking water indicator for public health protection. J. Appl. Microbiol. 88, 106S–116S. doi: 10.1111/j.1365-2672.2000.tb05338.x
Ekmekcioglu, Ö., Koc, K., Özger, M., and Işik, Z. (2022). Exploring the additional value of class imbalance distributions on interpretable flash flood susceptibility prediction in the Black Warrior River basin, Alabama, United States. J. Hydrol. 610:127877. doi: 10.1016/j.jhydrol.2022.127877
Emery, B., Fullerton, H., and Bossak, B. (2022). Resistance on the rise: assessment of antibiotic-resistant indicator organisms in Shem Creek, Charleston, South Carolina. Dialog. Health 1:100063. doi: 10.1016/j.dialog.2022.100063
FEMA (2020). Flood Zones. Available online at: https://www.fema.gov/glossary/flood-zones (accessed August 14, 2023).
Ghanbari, M., Arabi, M., Kao, S. C., Obeysekera, J., and Sweet, W. (2021). Climate change and changes in compound coastal-riverine flooding hazard along the U.S. coasts. Earths Fut. 9:e2021EF002055. doi: 10.1029/2021EF002055
Goji Tumba, A., and Amusuk, D. J. (2017). Hydrological modelling of potential flood areas from ASTER DEM using arc hydro tools and HEC-HMS. J. Multidisc. Eng. Sci. Technol. 4, 2458–9403.
Hamad, M., Al-Marzooq, F., Orive, G., and Al-Tel, T. H. (2019). Superbugs but no drugs: steps in averting a post-antibiotic era. Drug. Discov. Today 24, 2225–2228. doi: 10.1016/j.drudis.2019.08.004
Hamilton, K. A., Garner, E., Joshi, S., Ahmed, W., Ashbolt, N., Medema, G., et al. (2020). Antimicrobial-resistant microorganisms and their genetic determinants in stormwater: a systematic review. Curr. Opin. Environ. Sci. Health 16, 101–112. doi: 10.1016/j.coesh.2020.02.012
Harwood, V. J., Whitlock, J., and Withington, V. (2000). Classification of antibiotic resistance patterns of indicator bacteria by discriminant analysis: use in predicting the source of fecal contamination in subtropical waters. Appl. Environ. Microbiol. 66, 3698–3704. doi: 10.1128/AEM.66.9.3698-3704.2000
Hendry, A., Haigh, I. D., Nicholls, R. J., Winter, H., Neal, R., Wahl, T., et al. (2019). Assessing the characteristics and drivers of compound flooding events around the UK coast. Hydrol. Earth Syst. Sci. 23, 3117–3139. doi: 10.5194/hess-23-3117-2019
Holcomb, D. A., and Stewart, J. R. (2020). Microbial indicators of fecal pollution: recent progress and challenges in assessing water quality. Curr. Environ. Health Rep. 7, 311–324. doi: 10.1007/s40572-020-00278-1
Horton, C. (2022). Coastal Stormwater Pond Pollutants and the Potential for Development of Antimicrobial Resistance in Vibrio and Enterococcus Bacteria. Theses and Dissertations. Available online at: https://scholarcommons.sc.edu/etd/7029 (accessed August 14, 2023).
Jeong, H., Choi, J. Y., Lee, J., Lim, J., and Ra, K. (2020). Heavy metal pollution by road-deposited sediments and its contribution to total suspended solids in rainfall runoff from intensive industrial areas. Environ. Pollut. 265:115028. doi: 10.1016/j.envpol.2020.115028
Jongman, B., Ward, P. J., and Aerts, J. C. J. H. (2012). Global exposure to river and coastal flooding: long term trends and changes. Global Environ. Change 22, 823–835. doi: 10.1016/j.gloenvcha.2012.07.004
Joshi, U. M., and Balasubramanian, R. (2010). Characteristics and environmental mobility of trace elements in urban runoff. Chemosphere 80, 310–318. doi: 10.1016/j.chemosphere.2010.03.059
Kassambara, A., and Mundt, F. (2020). factoextra: Extract and Visualize the Results of Multivariate Data Analyses. R package version 1.0.7.99. Available online at: http://www.sthda.com/english/rpkgs/factoextra (accessed December 20, 2023).
Kelsey, R. H., Scott, G. I., Porter, D. E., Thompson, B., and Webster, L. (2003). “Using multiple antibiotic resistance and land use characteristics to determine sources of fecal coliform bacterial pollution,” in Coastal Monitoring through Partnerships: Proceedings of the Fifth Symposium on the Environmental Monitoring and Assessment Program (EMAP) Pensacola Beach, FL, USA (Springer Netherlands), 337–348. doi: 10.1007/978-94-017-0299-7_28
Khan, S. D. (2005). Urban development and flooding in Houston Texas, inferences from remote sensing data using neural network technique. Environ. Geol. 47, 1120–1127. doi: 10.1007/s00254-005-1246-x
Kirker, A., and Vulava, V. M. (2022). Identification of stormwater pollution hotspots in charleston peninsula. J. South Carolina Water Resour. 8:7. doi: 10.34068/JSCWR/08.01.07
Kodaka, H., Teramura, H., Nirazuka, T., Mizuochi, S., Goins, D., Odumeru, J., et al. (2006). Comparison of the compact dry CF with the most probable number method (AOAC Official Method 966.24) for enumeration of coliform bacteria in raw meats: performance-tested methodSM 110401. J. AOAC Int. 89, 115–126. doi: 10.1093/jaoac/89.1.115
Kulp, S. A., and Strauss, B. H. (2019). New elevation data triple estimates of global vulnerability to sea-level rise and coastal flooding. Nat. Commun. 10, 1–12. doi: 10.1038/s41467-019-12808-z
Lau, Y. Y., Yip, T. L., Dulebenets, M. A., Tang, Y. M., and Kawasaki, T. (2022). A review of historical changes of tropical and extra-tropical cyclones: a comparative analysis of the United States, Europe, and Asia. Int. J. Environ. Res. Public Health 19:4499. doi: 10.3390/ijerph19084499
Lever, J., Krzywinski, M., and Altman, N. (2017). Points of significance: principal component analysis. Nat. Methods 14, 641–642. doi: 10.1038/nmeth.4346
Li, C., Peng, C., Chiang, P. C., Cai, Y., Wang, X., and Yang, Z. (2019). Mechanisms and applications of green infrastructure practices for stormwater control: a review. J. Hydrol. 568, 626–637. doi: 10.1016/j.jhydrol.2018.10.074
Lindsey, R. (2022). Climate Change: Global Sea Level. Available online at: https://www.climate.gov/news-features/understanding-climate/climate-change-global-sea-level (accessed December 20, 2023).
Liu, W.-C., and Huang, W.-C. (2012). Modeling the transport and distribution of fecal coliform in a tidal estuary. Sci. Total Environ. 431, 1–8. doi: 10.1016/j.scitotenv.2012.05.016
MacDonald, K., and Tippett, M. (2020). Reducing public exposure to common, harmful well water contaminants through targeted outreach to highly susceptible neighborhoods as a method of increasing the likelihood of testing and treatment of water from private wells. J. Water Health 18, 522–532. doi: 10.2166/wh.2020.059
Manaia, C. M., Vaz-Moreira, I., and Nunes, O. C. (2012). Antibiotic resistance in waste water and surface water and human health implications. Handb. Environ. Chem. 20, 173–212. doi: 10.1007/698_2011_118
Martin, T. D., Creed, J. T., and Brockhoff, C. A. (1994). Sample preparation procedure for spectrochemical determination of total recoverable elements. U.S. Environmental Protection Agency Report, Revision 2.8, EMMC Version, 12. Available online at: https://www.nemi.gov/methods/method_summary/9799/ (accessed December 20, 2023).
McCoy, N., Chao, B., and Gang, D. D. (2015). Nonpoint source pollution. Water Environ. Res. 87, 1576–1594. doi: 10.2175/106143015X14338845156263
Meinhardt, P. L. (2006). Recognizing waterborne disease and the health effects of water contamination: a review of the challenges facing the medical community in the United States. J. Water Health 4, 27–34. doi: 10.2166/wh.2006.0041
Murray, C. J., Ikuta, K. S., Sharara, F., Swetschinski, L., Robles Aguilar, G., Gray, A., et al. (2022). Global burden of bacterial antimicrobial resistance in 2019: a systematic analysis. Lancet 399, 629–655. doi: 10.1016/S0140-6736(21)02724-0
National Water Quality Monitoring Council (2023). Water Quality Data Home. Available online at: https://www.waterqualitydata.us/ (accessed November 13, 2023).
National Weather Service and NOAA (2024). Climate - National Weather Service. Available online at: https://www.weather.gov/wrh/Climate?wfo=chs (accessed October 15, 2023).
Neumann, B., Vafeidis, A. T., Zimmermann, J., and Nicholls, R. J. (2015). Future coastal population growth and exposure to sea-level rise and coastal flooding - a global assessment. PLoS ONE 10:e0118571. doi: 10.1371/journal.pone.0118571
Niemi, M., Sibakov, M., and Niemela, S. (1983). Antibiotic resistance among different species of fecal coliforms isolated from water samples. Appl. Environ. Microbiol. 45, 79–83. doi: 10.1128/aem.45.1.79-83.1983
Odonkor, S. T., and Ampofo, J. K. (2013). Escherichia coli as an indicator of bacteriological quality of water: an overview. Microbiol. Res. 4:e2. doi: 10.4081/mr.2013.e2
O'Driscoll, M., Clinton, S., Jefferson, A., Manda, A., and McMillan, S. (2010). Urbanization effects on watershed hydrology and in-stream processes in the Southern United States. Water 2, 605–648. doi: 10.3390/w2030605
Office for Coastal Management and NOAA (2024). Economics and Demographics. Available online at: https://coast.noaa.gov/states/fast-facts/economics-and-demographics.html (accessed March 7, 2024).
Ohenhen, L. O., Shirzaei, M., Ojha, C., and Kirwan, M. L. (2023). Hidden vulnerability of US Atlantic coast to sea-level rise due to vertical land motion. Nat. Commun. 14:2038. doi: 10.1038/s41467-023-37853-7
Parker, J. K., McIntyre, D., and Noble, R. T. (2010). Characterizing fecal contamination in stormwater runoff in coastal North Carolina, USA. Water Res. 44, 4186–4194. doi: 10.1016/j.watres.2010.05.018
Perkins, A., and Trimmier, M. (2017). Recreational waterborne illnesses: recognition, treatment, and prevention. Am. Fam. Phys. 95, 554–560.
Petrucci, G., Gromaire, M. C., Shorshani, M. F., and Chebbo, G. (2014). Nonpoint source pollution of urban stormwater runoff: a methodology for source analysis. Environ. Sci. Pollut. Res. 21, 10225–10242. doi: 10.1007/s11356-014-2845-4
Powers, N. C., Wallgren, H. R., Marbach, S., and Turner, J. W. (2020). Relationship between Rainfall, fecal pollution, antimicrobial resistance, and microbial diversity in an urbanized subtropical bay. Appl. Environ. Microbiol. 86, e01229–20. doi: 10.1128/AEM.01229-20
Presley, S. M., Rainwater, T. R., Austin, G. P., Platt, S. G., Zak, J. C., Cobb, G. P., et al. (2005). Assessment of pathogens and toxicants in New Orleans, LA following hurricane katrina. Environ. Sci. Technol. 40, 468–474. doi: 10.1021/es052219p
Purcell, A. D., Khanal, P. N., Straka, T. J., and Cook, E. D. (2020). South Carolina's Coastal Habitats and Regional Comparison. Available online at: https://repository.library.noaa.gov/view/noaa/38546 (accessed August 14, 2023).
Qian, J. H., Viner, B., Noble, S., and Werth, D. (2021). Precipitation characteristics of warm season weather types in the southeastern United States of America. Atmosphere 12:1001. doi: 10.3390/atmos12081001
Qin, Y. (2020). Urban flooding mitigation techniques: a systematic review and future studies. Water 12:3579. doi: 10.3390/w12123579
Rentschler, J., Avner, P., Marconcini, M., Su, R., Strano, E., Vousdoukas, M., et al. (2023). Global evidence of rapid urban growth in flood zones since 1985. Nature 622, 87–92. doi: 10.1038/s41586-023-06468-9
Revelle, W. (2023). The psych package for personality and psychological research. Available online at: https://personality-project.org/r/psych/ (accessed December 11, 2023).
Rizzo, L., Manaia, C., Merlin, C., Schwartz, T., Dagot, C., Ploy, M. C., et al. (2013). Urban wastewater treatment plants as hotspots for antibiotic resistant bacteria and genes spread into the environment: a review. Sci. Total Environ. 447, 345–360. doi: 10.1016/j.scitotenv.2013.01.032
Rock, C., and Rivera, B. (2014). Water quality, E. coli and your health. College of Agriculture and Life Sciences, 4. Available online at: https://extension.arizona.edu/sites/extension.arizona.edu/files/pubs/az1624.pdf (accessed December 20, 2023).
Rusydi, A. F. (2018). “Correlation between conductivity and total dissolved solid in various type of water: a review,” in IOP Conference Series: Earth and Environmental Science, 118. doi: 10.1088/1755-1315/118/1/012019
Salarieh, B., Ugwu, I. A., and Salman, A. M. (2023). Impact of changes in sea surface temperature due to climate change on hurricane wind and storm surge hazards across US Atlantic and Gulf coast regions. SN Appl. Sci. 5, 1–19. doi: 10.1007/s42452-023-05423-7
Sanger, D. M., Holland, A. F., and Scott, G. I. (1999). Tidal creek and salt marsh sediments in South Carolina coastal estuaries: II. Distribution of organic contaminants. Arch. Environ. Contam. Toxicol. 37, 458–471. doi: 10.1007/s002449900540
SC DHEC (2019). Counting Rules for Membrane Filtration Methods for Coliform Bacteria. Available online at: https://scdhec.gov/sites/default/files/docs/Environment/docs/MF-Counting-Rules-1-15-2010.pdf (accessed October 12, 2023).
Schwertman, N. C., and de Silva, R. (2007). Identifying outliers with sequential fences. Comput. Stat. Data Anal. 51, 3800–3810. doi: 10.1016/j.csda.2006.01.019
Scott, G. I., Porter, D. E., Norman, R. S., Scott, C. H., Uyaguari-Diaz, M. I., Maruya, K. A., et al. (2016). Antibiotics as CECs: an overview of the hazards posed by antibiotics and antibiotic resistance. Front. Mar. Sci. 3:180207. doi: 10.3389/fmars.2016.00024
Selvakumar, A., and Borst, M. (2006). Variation of microorganism concentrations in urban stormwater runoff with land use and seasons. J. Water Health 4, 109–124. doi: 10.2166/wh.2006.0009
Silverstein, K., and NOAA (2023). U.S. high tide flooding continues to break records. Available online at: https://www.noaa.gov/news-release/us-high-tide-flooding-continues-to-break-records (accessed October 12, 2023).
Singh, R., Singh, A. P., Kumar, S., Giri, B. S., and Kim, K. H. (2019). Antibiotic resistance in major rivers in the world: A systematic review on occurrence, emergence, and management strategies. J. Clean Prod. 234, 1484–1505. doi: 10.1016/j.jclepro.2019.06.243
Skeeter, W. J., Senkbeil, J. C., and Keellings, D. J. (2019). Spatial and temporal changes in the frequency and magnitude of intense precipitation events in the southeastern United States. Int. J. Climatol. 39, 768–782. doi: 10.1002/joc.5841
Spanger-Siegfried, E., Fitzpatrick, M., and Dahl, K. (2014). Encroaching Tides: How Sea Level Rise and Tidal Flooding Threaten U.S. East and Gulf Coast Communities over the Next 30 Years. Available online at: http://conservancy.umn.edu/handle/11299/189228 (accessed October 12, 2023).
Sweet, W. V., and Park, J. (2014). From the extreme to the mean: Acceleration and tipping points of coastal inundation from sea level rise. Earths Fut. 2, 579–600. doi: 10.1002/2014EF000272
Tellman, B., Sullivan, J. A., Kuhn, C., Kettner, A. J., Doyle, C. S., Brakenridge, G. R., et al. (2021). Satellite imaging reveals increased proportion of population exposed to floods. Nature 596, 80–86. doi: 10.1038/s41586-021-03695-w
US Census Bureau (2023). Charleston County, SC - Census Bureau Tables. Available online at: https://data.census.gov/table?q=Charleston+County,+SCandy=2020 (accessed October 12, 2023).
USDA (2019). Major Land Uses. Available online at: https://www.ers.usda.gov/topics/farm-economy/land-use-land-value-tenure/major-land-uses/ (accessed October 12, 2023).
USEPA (2017). Infographic: Lead in Drinking Water. Available online at: https://www.epa.gov/sites/default/files/2017-08/documents/epa_lead_in_drinking_water_final_8.21.17.pdf (accessed December 20, 2023).
USEPA (2021). Industrial Stormwater Monitoring and Sampling Guide. Washington, DC. Available online at: https://www.epa.gov/sites/default/files/2015-11/documents/msgp_monitoring_guide.pdf (accessed October 15, 2023).
USEPA (2023). National Recommended Water Quality Criteria - Aquatic Life Criteria Table. Available online at: https://www.epa.gov/wqc/national-recommended-water-quality-criteria-aquatic-life-criteria-table (accessed October 12, 2023).
USGS (2021). National Land Cover Database. Available online at: https://www.usgs.gov/centers/eros/science/national-land-cover-database (accessed October 12, 2023).
Uyaguari, M., Norman, S., Gooch, J., Jackson, K., and Scott, G. I. (2011). The discovery of novel bacterial antibiotic resistance genes in activated sludge using a metagenomic approach. J. Appl. Environ. Microbiol. 77, 8226–8233. doi: 10.1128/AEM.02732-10
Uyaguari, M. I., Scott, G. I., and Norman, R. S. (2013). Abundance of class 1–3 integrons in South Carolina estuarine ecosystems under high and low levels of anthropogenic influence. Mar. Pollut. Bull. 76, 77–84. doi: 10.1016/j.marpolbul.2013.09.027
Velazquez-Meza, M. E., Galarde-López, M., Carrillo-Quiróz, B., and Alpuche-Aranda, C. M. (2022). Antimicrobial resistance: one Health approach. Vet. World 15, 743–749. doi: 10.14202/vetworld.2022.743-749
Villines, J. A., Agouridis, C. T., Warner, R. C., and Barton, C. D. (2015). Using GIS to delineate headwater stream origins in the appalachian coalfields of kentucky. JAWRA 51, 1667–1687. doi: 10.1111/1752-1688.12350
Whitlock, J. E., Jones, D. T., and Harwood, V. J. (2002). Identification of the sources of fecal coliforms in an urban watershed using antibiotic resistance analysis. Water Res. 36, 4273–4282. doi: 10.1016/S0043-1354(02)00139-2
Wickham, H. (2016). ggplot2 - Elegant Graphics for Data Analysis., 2nd Edn. Cham: Springer International Publishing.
Wright, M. S., Peltier, G. L., Stepanauskas, R., and McArthur, J. V. (2006). Bacterial tolerances to metals and antibiotics in metal-contaminated and reference streams. FEMS Microbiol. Ecol. 58, 293–302. doi: 10.1111/j.1574-6941.2006.00154.x
Yang, S. H., Chen, C. H., and Chu, K. H. (2021). Fecal indicators, pathogens, antibiotic resistance genes, and ecotoxicity in Galveston Bay after Hurricane Harvey. J. Hazard Mater. 411:124953. doi: 10.1016/j.jhazmat.2020.124953
Zhang, W., Villarini, G., Vecchi, G. A., and Smith, J. A. (2018). Urbanization exacerbated the rainfall and flooding caused by hurricane Harvey in Houston. Nature 563, 384–388. doi: 10.1038/s41586-018-0676-z
Keywords: antibiotic resistance, flooding, urbanization, fecal coliforms, ampicillin
Citation: Squiggins KT, Fullerton H and Vulava VM (2024) The presence of ampicillin-resistant coliforms in urban floodwaters of a coastal city in the southeastern United States. Front. Water 6:1359196. doi: 10.3389/frwa.2024.1359196
Received: 20 December 2023; Accepted: 01 April 2024;
Published: 17 April 2024.
Edited by:
Prabhakar Sharma, Nagaland University, IndiaReviewed by:
Salom Gnana Thanga Vincent, University of Kerala, IndiaSabu Thomas, Mahatma Gandhi University, India
Copyright © 2024 Squiggins, Fullerton and Vulava. This is an open-access article distributed under the terms of the Creative Commons Attribution License (CC BY). The use, distribution or reproduction in other forums is permitted, provided the original author(s) and the copyright owner(s) are credited and that the original publication in this journal is cited, in accordance with accepted academic practice. No use, distribution or reproduction is permitted which does not comply with these terms.
*Correspondence: Vijay M. Vulava, dnVsYXZhdkBjb2ZjLmVkdQ==