- 1Department of Chemistry and Earth Sciences, Program of Chemistry, College of Arts and Sciences, Qatar University, Doha, Qatar
- 2Central Laboratories Unit, Office of VP for Research and Graduate Studies, Qatar University, Doha, Qatar
Electrocoagulation is a water treatment technology capable to remove a variety of organic pollutants from water. It is advantageous compared to chemical coagulation due to the controlled dissolution of coagulants by regulating the current density and pH. In this work, the removal of kinetic hydrate inhibitor (KHI) (polyvinyl pyrrolidone, PVP) from water by electrocoagulation using Al electrodes was investigated. The effects of several experimental conditions including the nature of the supporting electrolyte, the current density, and the initial pH value on the electrochemical dissolution of aluminum was evaluated. The findings of the experiments revealed that both chemical and electrochemical dissolution play important roles in the generation of hydroxo-aluminum species. Corrosion studies demonstrated that the presence of chloride ions in water promotes aluminum dissolving via pitting corrosion, whereas the presence of phosphate ions inhibits aluminum corrosion by the deposition of a thick passive layer of aluminum hydroxide/phosphate on the metal surface. The theoretical and experimental amounts of aluminum, increase linearly with increasing specific electrical charge for Q< 2.5 Ah/L, which correlates well with Faraday's Law. The removal of KHI from 0.1M NaCl aqueous solutions by electrocoagulation using aluminum electrodes achieved high removal efficiency in terms of total organic carbon (TOC) up to 95%. TOC decay during galvanostatic electrolysis confirmed the removal of KHI molecules by Al-electrocoagulation at different current densities and pH conditions. The primary mechanism involved in eliminating KHI from water by electrocoagulation using Al electrodes includes mainly the adsorption of PVP molecules on the surface of Al(OH)3 flocs and their enmeshment inside the solid coagulants.
1 Introduction
Electrocoagulation is an electrochemical technique for water treatment based on the anodic dissolution of a sacrificial metal, by averaging the related reactions such as the cathodic reduction of water that gives hydroxo-metal complexes, which ensure the destabilization and the adsorption of the dissolved pollutants and the flocculation of the aggregates formed (Ghernaout, 2019; Romani et al., 2020; Jovanović et al., 2021; Lucakova et al., 2021; AlJaberi et al., 2022; Lu et al., 2022; Medina-Collana et al., 2023). It is considered a complex electrochemical process with the involvement of several synergistic mechanisms contributing to the elimination of water pollution (Bensalah, 2011; Orescanin et al., 2014; Abdulrazzaq et al., 2021; Elkhatib et al., 2021; Idusuyi et al., 2022). The electrocoagulation is a technique derived from the conventional chemical method of coagulation-flocculation. In an electrochemical coagulation process, the metal cations are generated in situ and the elimination of pollutants from the water takes place. The electrocoagulation starts by the generation of coagulants by electrochemical dissolution of the anode material, followed by neutralization of the colloidal charges, co-precipitation of heavy metals, and breaking up of oil/water emulsions, and finally the formation of flocs that sediment (Mollah et al., 2001, 2004; Akter et al., 2022; Morales-Figueroa et al., 2022; Othmani et al., 2022). The anodic dissolution of aluminum and iron electrodes generates the corresponding metal cations, which hydrolyze immediately and form hydroxo-metal complexes (Ghernaout, 2018; Morales-Figueroa et al., 2022; Othmani et al., 2022). Theses hydroxo-metal complexes (Ghernaout, 2018; Morales-Figueroa et al., 2022; Othmani et al., 2022) combine with the negative colloidal particles and destabilize them. The water pollutants can be eliminated by different routes including adsorption on the surface of the coagulants, co-precipitation, or by incorporation inside the structure of the hydroxo-metal complexes (Al-Qodah and Al-Shannag, 2017; Hendaoui et al., 2021; Akter et al., 2022; Othmani et al., 2022; Hu et al., 2023). They are then removed by electroflotation, or sedimentation and filtration (Al-Qodah and Al-Shannag, 2017; Hendaoui et al., 2021; Akter et al., 2022; Othmani et al., 2022; Hu et al., 2023). Thus, instead of adding chemical coagulants as in the conventional coagulation method, the coagulating agents are generated in situ (Mollah et al., 2004; Tchamango et al., 2018; Jovanović et al., 2021). Meanwhile, the electrolysis of water generates bubbles of hydrogen gas by cathodic reduction of water and oxygen gas by anodic oxidation of water. These bubbles facilitate the flotation of colloidal particles at the surface. The electrochemical reactions taking place in the medium when a sacrificial metal M is used as anode are (Mollah et al., 2001, 2004; Romani et al., 2020):
At the anode:
At the cathode:
If aluminum electrodes are used, Al3+(aq) are immediately generated. These metal cations undergo further spontaneous reactions to produce hydroxides, oxyhydroxides and/or corresponding poly-hydroxides. For example, hydrolysis of Al3+ cations can generate Al(H2O), Al(H2O)5OH2+, Al(H2O)4(OH). These hydrolysis products can form several monomeric and polymeric species such as Al(OH)2+, Al(OH), Al2(OH), Al(OH)3, Al(OH), Al6(OH), Al7(OH), Al8(OH), Al13O4(OH), Al13(OH) depending on the pH of the medium (Khadir et al., 2021; Trompette and Lahitte, 2021; Zeng et al., 2021; Morales-Figueroa et al., 2022). These hydrolysis products have a high affinity for the dispersed particles and the charged particles to cause their coagulation. The gases evolved at the electrodes can cause flotation of the flocs. The electrocoagulation is intrinsically associated with electro-flotation because of the electro-generation of hydrogen and oxygen bubbles at cathode and the anode, respectively. The success of water treatment by electrocoagulation is related to the size of the bubbles (Abdulrazzaq et al., 2021; Elkhatib et al., 2021; Biswas and Goel, 2022), as well as the agitation caused by the release of hydrogen and oxygen bubbles (Abdulrazzaq et al., 2021; Elkhatib et al., 2021). Several parameters affect the efficiency of removal of pollutants from water by electrocoagulation. The main parameters known to have a significant effect on the efficiency of the electrocoagulation process are illustrated in Figure 1A (Mamelkina et al., 2019; Asaithambi et al., 2021; Ebba et al., 2021; Zeng et al., 2021; Ansari and Shrikhande, 2022; Ansari et al., 2022; Rookesh et al., 2022).
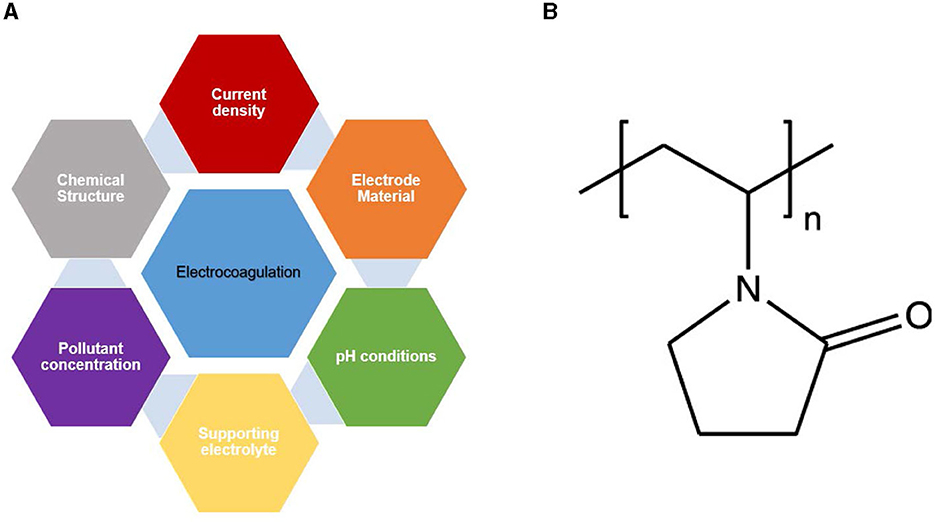
Figure 1. (A) The main parameters affecting the efficiency of electrocoagulation, (B) Chemical structure of poly(N-vinyl pyrrolidone) (PVP).
The understanding of the physico-chemical processes involved in electrocoagulation will certainly result in large applications of this sustainable advanced technology. Among the technical challenges facing the progress of electrocoagulation is related to the formation of passive layers on the surface of aluminum and iron electrodes (Rodriguez et al., 2007; Mansouri et al., 2011a, 2012; Xu et al., 2017; Romani et al., 2020; Trompette and Lahitte, 2021). The corrosion resistance of Al and Fe depends largely on the formation/breakup of the non-conductive metal oxide layer deposited on the surface. The good adhesion of the non-conductive oxide film to the anode surface decelerates the charge transfer between the solution and the electrodes, and then decreases the rate of anodic dissolution of Al in solution. Passivation of aluminum anodes results in higher energy consumption and lessens the effectiveness of electrocoagulation process. The corrosion rate of the sacrificial anode of aluminum mainly depends on the formation and growth of the passive layer of metal oxide, and its elimination by pitting corrosion. The pitting corrosion of aluminum is much more vulnerable than other metals. The addition of certain supporting electrolytes such as NaCl, can reduce the passivation of the electrodes and improve ionic conductivity of the solution (Mansouri et al., 2011a, 2012; Acharya et al., 2022; Morales-Figueroa et al., 2022; Medina-Collana et al., 2023). The use of an alternating current source with variable pulse frequency can also prevent the formation of deposits on the surface of the electrodes (Asaithambi et al., 2021; Othmani et al., 2022). However, the impacts of experimental conditions including the nature of supporting electrolyte, the pH of the medium, and the current density on the rate of formation and destruction of the protective layer, which adheres to the surface of the Al attackable electrodes have not been deeply investigated (Mansouri et al., 2011b, 2012; Trompette and Lahitte, 2021).
The hydrates of gas are like ice crystalline solids, which are produced through water lattice enclathrating specific gas molecule at confirmed thermodynamic conditions (Lim et al., 2020; Sayani et al., 2020; Li et al., 2022). Formation of the gas hydrate is a challenging concern in gas and oil industry, as it may blocks the pipelines and the processes amenities (Rebai et al., 2019; Sayani et al., 2020; Veluswamy and Linga, 2021). The formation of gas hydrate also can take place throughout the drilling operations of deep water and outcome in earnest problems (Adham et al., 2014; Anderson et al., 2016; Dehghan et al., 2021). As a result, a variety of chemical additives named hydrate inhibitors are added to prevent the problems of hydrate formation. Moreover, two types of hydrate inhibitors exist including the thermodynamic inhibitors (THI) and the low dosage hydrate inhibitors. The low dosage hydrate inhibitors are subdivided into two categories these are the kinetic hydrate inhibitors (KHIs) and anti-conglomerates. KHIs water-soluble polymers are commercially used to prevent gas hydrate formation in fossil fuels production lines (Golpour and Pakizeh, 2017; Li et al., 2019; Wang et al., 2019; Saberi et al., 2021). KHIs delay the nucleation and the growth of gas hydrate crystals in the system (Golpour and Pakizeh, 2017; Li et al., 2019; Wang et al., 2019; Saberi et al., 2021). However, organic KHIs are non-biodegradable substances, which makes them facing restraints in their utilization by several environmental agencies. Furthermore, industry regulators have concluded that the presence of KHI polymers in the injected wastewater leads to long-term reservoir damage (Golpour and Pakizeh, 2017; Li et al., 2019; Wang et al., 2019; Saberi et al., 2021). The removal of KHIs by physicochemical techniques (nanofiltration, adsorption) was the subject of few studies (Anderson et al., 2016; Golpour and Pakizeh, 2017; Dehghan et al., 2021; Mozaffar et al., 2022). These techniques failed to remove high amounts of KHIs from wastewater further to their expensive costs, strict operational conditions, and production of secondary pollution. It is essential to find a highly efficient and cost-effective technique capable to completely remove KHI polymers from oil and gas processing wastewater streams.
In this work, the removal of polyvinyl pyrrolidone (PVP) (Figure 1B) largely used as KHI in oil and gas processing by electrocoagulation process using Al electrodes was investigated. The effects of certain experimental parameters on the anodic dissolution of aluminum was evaluated in order to find out the optimal conditions of in situ production of the coagulants. In addition, the removal efficiency of PVP from water in terms of total organic carbon (TOC) content was examined at different conditions of pH, current density and TOC content.
2 Materials and methods
2.1 Analytical methods
The aqueous solutions were prepared by dissolving analytical grade reagents NaCl (0.1 M), Na2SO4 (0.05 M), and NaH2PO4 (0.1 M) in deionized water. Samples were taken at specified times, then analyzed for the pH level and dissolved aluminum concentration. Analyses for total aluminum and total soluble and insoluble aluminum concentrations released during galvanostatic electrolysis were determined by inductively coupled plasma-optical emission spectroscopy (ICP-OES) analysis. The pH of the aqueous solution was monitored using Micronal pH meter (model B474). The morphology of aluminum anodes was observed using FEI NOVA NANOSEM 450 equipment. Elemental analysis by energy dispersive spectroscopy (EDS) was performed using the same SEM equipment. KHI solutions were prepared by the dilution of a stock solution containing 1,000 ppm of industrially commercialized KHI (PVP) in 0.1 M NaCl. The experimental conditions and setup were identical with the procedure described previously. Samples were collected at different times, and then filtered using PTFE filters (0.45 μm) for further analysis. KHI removal from water was evaluated by monitoring the total organic carbon (TOC) decay. TOC was measured using Skalar FormacsHT TOC/TN analyzer. All the analyses were performed in triplicate.
2.2 Potentiodynamic polarization experiments
A three-electrode electrochemical cell was used to perform the potentiodynamic polarization experiments using a potentiostat/galvanostat (20 V, 1 A) controlled by Gamry reference 3000 potentiostat software to allow data inception and collection. The working electrode (Al plate) was inserted into a PTFE sample holder. The auxiliary and the reference electrodes were platinum wire and a saturated calomel electrode (SCE), respectively. The working electrode potential was changed at a fixed scan rate of 0.5 mV s−1 between −2.0 V up to +2.0 V vs. SCE.
2.3 Anodic dissolution and galvanostatic electrolysis
The anodic dissolution of Aluminum was carried out under galvanostatic conditions (fixed current density). Working electrodes were comprised of 1 cm × 1 cm aluminum plates (99.9% purity, 2 mm thickness). Before immersing in the electrolyte solution for each experiment, the aluminum electrode was polished, washed with HCl (1.5 M), rinsed with deionized water, and then kept to be dried at room-temperature. The electrolysis experiments were carried out in a single-compartment, temperature-controlled electrochemical cell using rectangular and parallel aluminum electrodes. Temperature was adjusted to be constant at 24–25°C. The initial pH was attuned using HCl or NaOH (0.1 M) drops to achieve the wanted pH value in each experiment. The aluminum electrodes were connected to a digital DC power supply (Monacor PS-430), providing current and voltage in the range of 0–10 A and 0–20 V, respectively. The cell voltage was recorded using a potentiometer. The aqueous solution was stirred at a constant rate of 400 rpm. The pH, voltage, and current density were measured and recorded at specified times.
The galvanostatic electrolysis experiments were performed in a one-compartment electrolytic cylindrical cell with temperature-controlled jacket equipped with two parallel and rectangular aluminum (99.9% purity, 2 mm thickness, surface area immersed in the solution, 25 cm2) electrodes was used. Temperature was adjusted to be constant at 24°C. A digital DC power supply (Monacor PS-430) was connected to the aluminum electrodes as current supplier during the removal of KHI from water at different current densities (0–10 A and 0–20 V). The aqueous solutions were stirred at a constant rate of 400 rpm. The samples withdrawn at specified times were filtered using 0.45 μm PTFE (Whatman) filter and then analyzed for pH and TOC.
3 Result and discussion
3.1 Potentiodynamic polarization of aluminum
Corrosion studies can provide useful information that helps to understand the dissolution of aluminum during electrochemical coagulation process. The corrosion behavior of Al in aerated aqueous solutions was evaluated by potentiodynamic polarization tests. Potentiodynamic polarization curves of Al plates (1 cm2) in 0.1 M NaCl, 0.05 M Na2SO4 and 0.1 M NaH2PO4 are presented in Figure 2. Tafel extrapolation method was used to determine the corrosion potential and corrosion rate in each medium. The anodic polarization branches do not have the same profile as that predicted by Tafel over the potential window. A classical passive region in which the anodic current remained almost constant with the change of the working electrode potential in Na2SO4 and NaH2PO4. However, in NaCl, a different behavior was observed: an abrupt increase in the current was observed when the value of potential reaches the pitting potential. Al corrosion potentials of in presence of NaCl and Na2SO4 are −1016 mV/SCE and −1066 mV/SCE, respectively. In NaH2PO4, the corrosion potential increased to −654 mV/SCE, which indicates that the corrosion of aluminum was inhibited in 0.1 M NaH2PO4. Aqueous solution. Furthermore, the corrosion of aluminum is kinetically and thermodynamically was the most favorable in 0.1 M NaCl due to pitting corrosion that was observed only in presence of chloride ions. The rate of corrosion of Al electrodes depends mainly on (i) the deposition of a non-conductive film of aluminum oxide, and (ii) the peeling off the film by pitting corrosion. The electrochemical corrosion of aluminum in presence of chloride ions is accelerated by pitting (Mansouri et al., 2011a; Bani-Melhem et al., 2023).
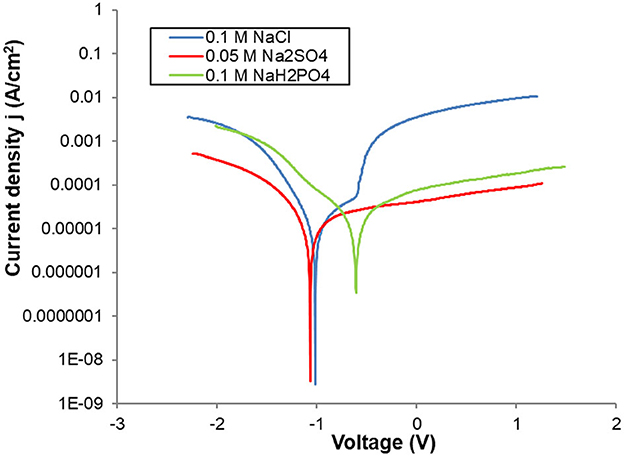
Figure 2. Potentiodynamic polarization curves of Al electrodes in different electrolytes in semi-logarithmic scale (for current density). Experimental conditions: working electrode: Al plate (1 cm2), Counter electrode: Platine wire, Reference electrode: Saturated Calomel electrode (SCE), T = 23°C.
The measurement of amount of dissolved Al species during galvanostatic electrolysis is very important because the amount and the speciation of dissolved Al species affects largely the efficiency of electrocoagulation process. The theoretical amount of dissolved aluminum during galvanostatic electrolysis can be calculated based on Faraday's law considering that the supplied energy into Al3+: Al ⇆ Al3+ + 3e. Based on Faraday's law, the amount of dissolved Al can be calculated using the following equation:
where [Al] is the amount of aluminum electrogenerated (in g/L); MMAl is the molecular weight of aluminum (MMAl = 26.98 g/mol); F is the Faraday's constant (F = 96,500 C/mol); 3,600 is a factor to convert h into s, and Q is the specific electrical charge (in Ah/L).
In order to investigate the anodic dissolution of Al during galvanostatic electrolyses under different experimental conditions, the concentration of Al dissolved at desired times was monitored by atomic spectroscopy. The experimental concentration of dissolved Al was compared to the concentration predicted by Faraday's law. As it can be seen from Figure 3, the experimental amounts of aluminum increase linearly with the specific electrical charge for Q < 0.5 Ah/L independently of current density. For Q< 0.5 Ah/L, the experimental amounts of aluminum fit with Faraday's Law. However, for Q >0.5 Ah/L, the experimental values surpassed those predicted Faraday's Law and the difference between them broadens with the increase of Q. The excessive amounts of dissolved Al can be due to the dissolution of Al anodes by chemical corrosion.
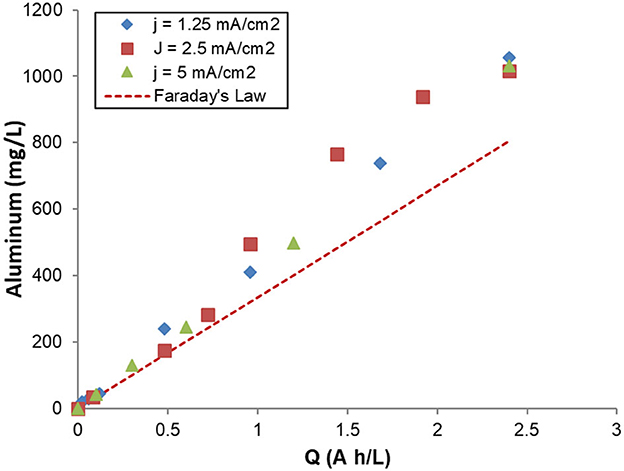
Figure 3. Changes of dissolved aluminum concentrations during galvanostatic electrolysis with specific electrical charge at different current densities. Operating conditions: initial pH = 7, supporting electrolytes: 0.1 M NaCl, stirring rate: 400 rpm.
Figure 4A presents the effect of initial pH on the amount of dissolved Al during electrolysis in 0.1 M NaCl at a current density of 2.5 mA/cm2. It is clear that the initial pH affects the changes of Al dissolved during electrolysis. For Q ≤ 1 Ah/L, dissolved Al increases linearly with Q independently of initial pH in the range 2.8–12.2 and similar amounts of Al are dissolved in acidic, neutral or alkaline media. However, For Q ≥ 1.5 Ah/L, Al dissolved at pH 9.2 and 12 deviate from linearity and reached plateaus, which can be due to the deposition of a nonconductive layer of hydroxo-aluminum species.
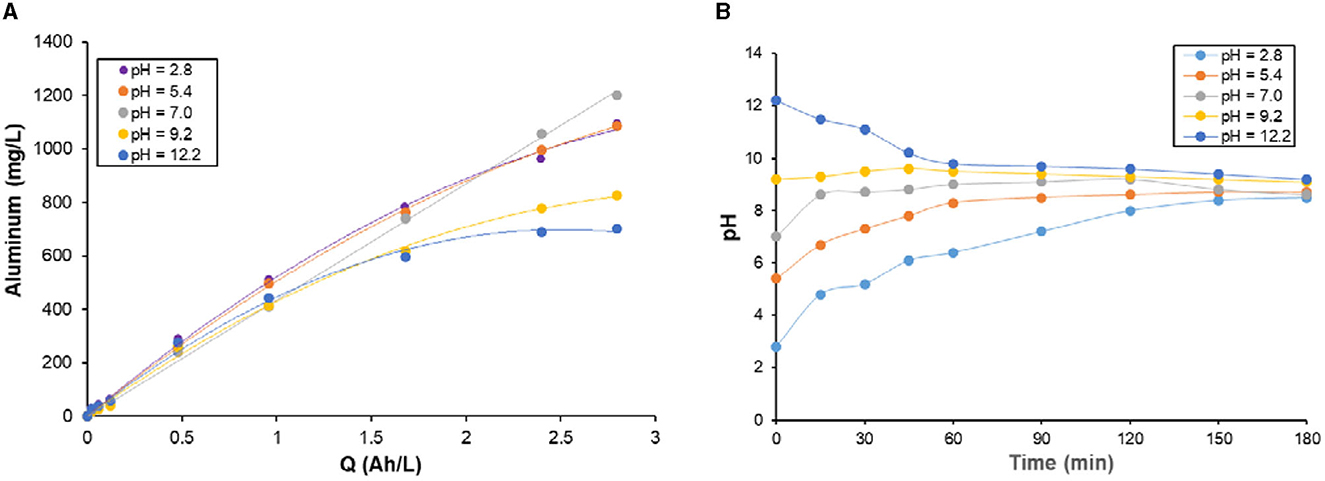
Figure 4. (A) Changes of experimental concentrations of aluminum dissolved, (B) Changes of pH with time during galvanostatic electrolysis with specific electrical charge at different pH in 0.1 M NaCl. Operating conditions: current density j = 2.5 mA/cm2, stirring rate: 400 rpm.
To better understand the effect of initial pH, the changes of pH vs. time are given in Figure 4B. As it can be seen, the changes of pH with time during galvanostatic electrolysis depends on the initial pH. The trend of pH with time started by rapid change (increase or decrease) of the pH up to 60 min, then no significant change in the pH was observed until the end of the electrolysis. The graphs of pH with time showed an increase of pH for aqueous solutions having initial pH values ≤ 7.0; however, a decrease in the pH was observed for solutions having initial pH values > 7.0. The increase of pH observed in acidic and neutral solutions can be due to the neutralization reaction by hydroxides ions (OH−) formed during the electrochemical reduction of H+/H2O at the cathode. In these conditions, it seems that not all the OH− ions formed by cathodic reduction of H+/H2O were engaged in the production of hydroxo-aluminum complexes, and the excessive amount of OH− ions increased the pH. In contrast, the decrease of pH for alkaline solutions could be ascribed the formation of anionic hydroxo-aluminum species such as Al(OH) and Al(OH), which consumes higher amounts of OH− ions. By the end of electrolysis, the pH of the aqueous solutions was maintained at pH in the range 8.5–9.2. As can be observed, the stabilization of the pH was reached quickly for initial pH values close to 9. The change of pH with time can be explicated by the buffering capacity of hydroxo-aluminum species (Mansouri et al., 2011b, 2012). This buffering capacity maintain the pH value in pH range 8.5–9.2 by the formation of monomeric and polymeric complexes of aluminum hydroxides.
Furthermore, morphological observations by scanning electron microscope (SEM) of Al anode surfaces after electrolyses at j = 2.5 mA/cm2 during 180 min in 0.1 NaCl, 0.05 M Na2SO4, and 0.1 M NaH2PO4 at pH 7 are shown in Figure 5. Large localized pitting corrosion was observed on the surface of Al anode in 0.1 M NaCl aqueous solutions. However, in the case of Na2SO4, uniform distributed pitting corrosion was observed. In contrast, in the presence of NaH2PO4, the surface of Al anode was completely covered with a thick layer of AlPO4 (Acharya et al., 2022). These results confirmed the importance of supporting electrolyte in the corrosion of aluminum (Mansouri et al., 2011b; Romani et al., 2020). Al corrosion is accelerated by the presence of chloride ions than sulfate or phosphate ions due to autocatalytic pitting corrosion. However, the formation of AlPO4 in 0.1 M NaH2PO4 that adheres to the surface of Al electrodes inhibits the corrosion of aluminum.
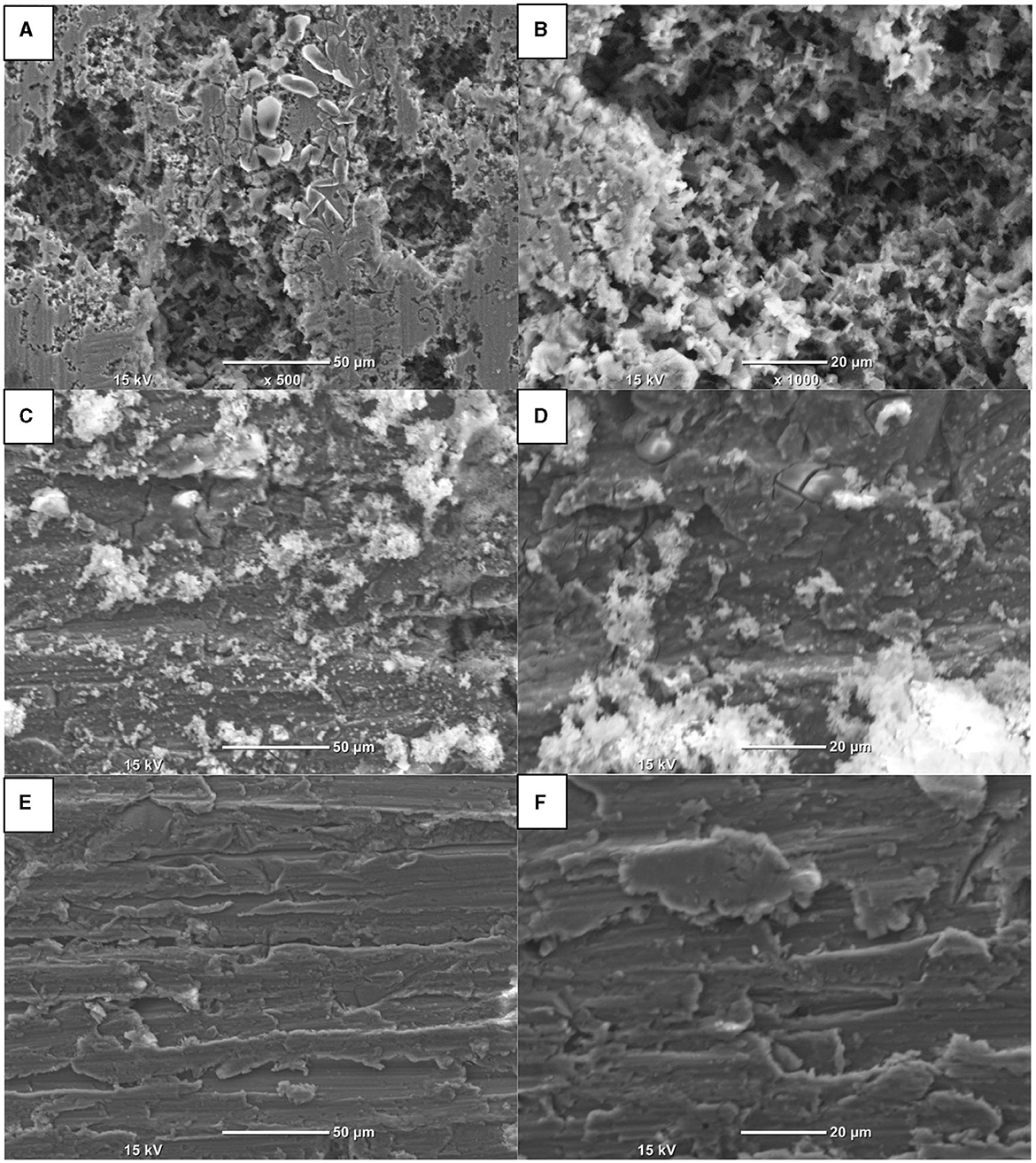
Figure 5. SEM micrographs of aluminum electrodes after galvanostatic electrolysis in (A, B) 0.1 M NaCl, (C, D) 0.05 M Na2SO4, and (E, F) 0.1 M NaH2PO4. Operating conditions: current density j = 2.5 mA/cm2, pH = 7, stirring speed: 400 rpm.
Besides the SEM micrographs, the solids precipitated during the electrolysis at j = 2.5 mA/cm2 for 180 min in 0.1 NaCl were collected, dried and observed directly by transmission electron microscope (TEM). The dried solids were also observed by SEM and analyzed by energy dispersive X-ray (EDS) (see Figures 6, 7). The TEM micrographs shows that the coagulation process starts by formation of nanofibrous materials that agglomerate to form amorphous porous aggregates increasing in size during the galvanostatic electrolysis. SEM results confirmed the results obtained by TEM regarding the size of coagulants. EDX analysis proved that hydroxo-aluminum precipitates are formed during anodic dissolution of Al, which leads to the formation of amorphous porous mixture of Al(OH)3 and Al2O3.
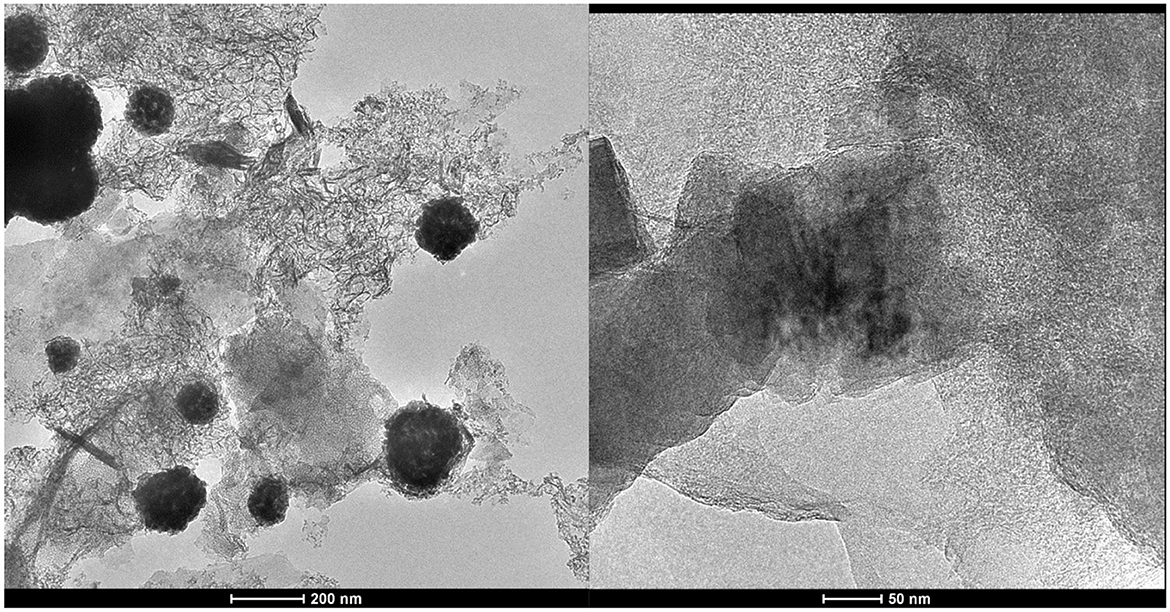
Figure 6. TEM micrographs of aluminum precipitate during galvanostatic electrolysis. Operating conditions: current density j = 2.5 mA/cm2, pH =7, supporting electrolytes: 0.1 M NaCl, stirring speed: 400 rpm.
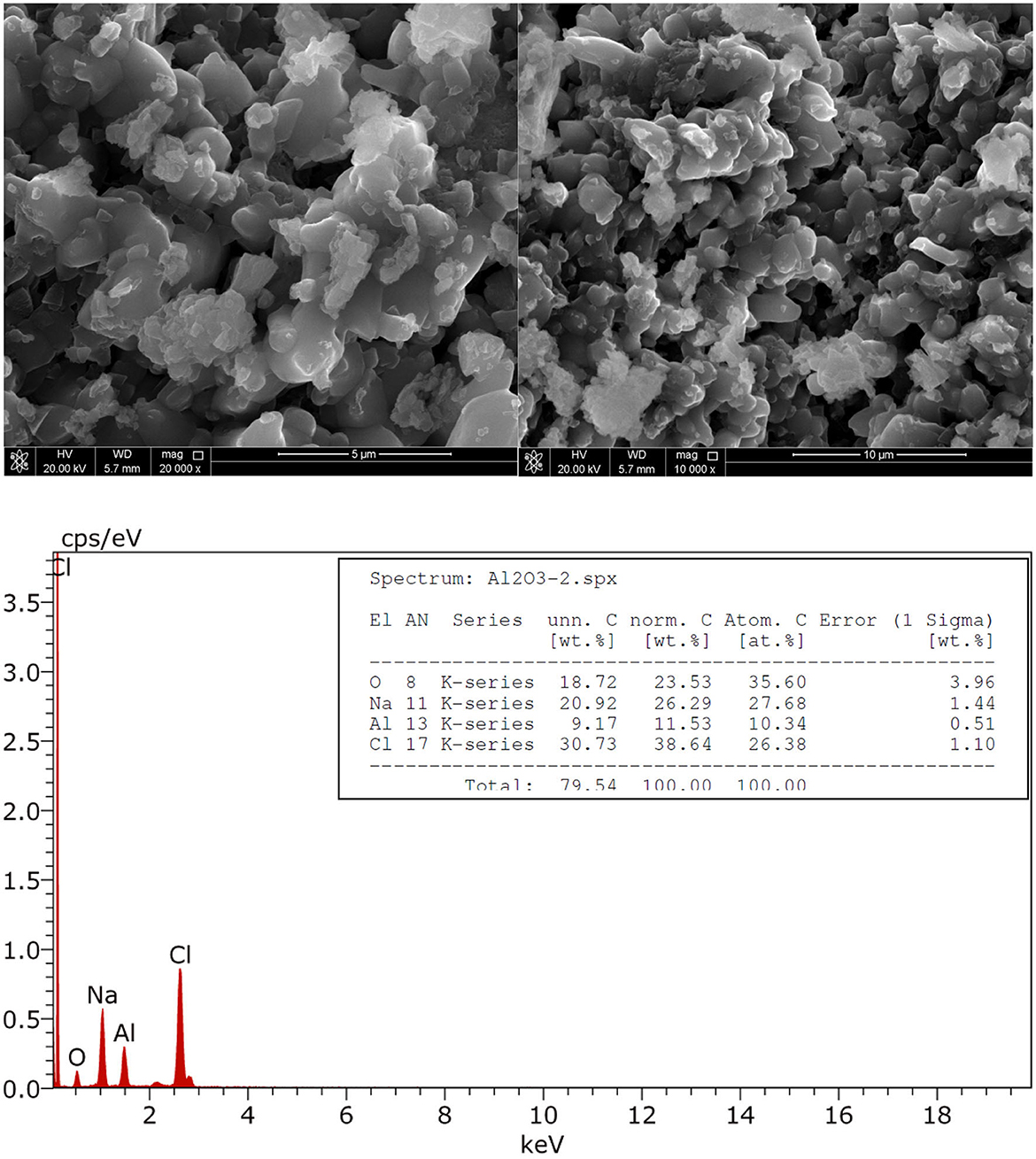
Figure 7. SEM micrographs and EDX spectra of aluminum precipitate during galvanostatic electrolysis. Operating conditions: current density j = 2.5 mA/cm2, pH = 7, supporting electrolytes: 0.1 M NaCl, stirring speed: 400 rpm.
3.2 Removal of KHI by al-electrocoagulation
Electrocoagulation process with sacrificial Al electrodes was selected as a sustainable and economic method for the removal of PVP (KHI) from water. The effects of certain operating conditions were evaluated during treatment of KHI contaminated water by Al-electrocoagulation. Experiments were performed at different initial pH values, different current densities, and different initial TOC contents. The efficiency of the electrocoagulation process in removing KHI from water was evaluated in terms of total organic carbon (TOC). Since the KHI (PVP) is the only organic compound in the medium, the changes of TOC during Al-electrocoagulation experiments reflect the changes in KHI concentration in water. The efficiency of Al-electrocoagulation is governed mainly by specific electric charge consumed and the amount of dissolved aluminum species (Mollah et al., 2001, 2004; Akter et al., 2022). However, these parameters should be kept as low as possible to achieve a low-cost treatment (Cañizares et al., 2007; Sillanpää and Shestakova, 2017; Marmanis et al., 2021; Villalobos-Lara et al., 2021).
The pH of water to be treated is one of the key parameters of electrocoagulation. The pH has an impact on the conductivity of the solution, the dissolution of the electrodes, the speciation of the hydrolysis products of the electrogenerated metal cations, and zeta potential of the colloidal particles. Hydroxo-metal complexes of aluminum cause destabilization of colloids. The most effective coagulating species are formed in acidic, neutral or slightly alkaline. When the pH is highly alkaline, hydrolysis of aluminum forms soluble anionic hydroxo-metal complexes such as Al(OH) ions which significantly reduce the effectiveness of electrocoagulation. The effect of pH on the efficiency of pollutants removal is mainly explained by the nature of metal hydrolysis products. However, the pH varies during electrocoagulation treatment, which makes it difficult to predict the effect of pH on the effectiveness of electrocoagulation and it is necessary to study its effect on the removal of each type of pollutant. Figure 8 presents the effect of initial pH on TOC removal during the treatment of KHI aqueous solution containing 200 mg C/L at 2.5 mA/cm2. As it can be seen, at the beginning of the electrolysis, TOC removal increases with increasing the specific electric charge and then it reaches a plateau after 0.5 Ah/L to stabilize until the end of the experiment. The highest TOC removal (92.6%) was achieved for solution having initial pH values 2.8, 5.4, and 7.0. Lowest TOC removal was obtained at 12. An increase in pH levels during Al-electrocoagulation process are related to OH− ions produced at the cathode electrode and H2 gas released from the cathode. In this pH range, amorphous aluminum hydroxide (Al(OH)3) are formed by the reaction of the electrogenerated Al3+ cations with the hydroxide anions produced by the reduction of water molecules at the cathode. Al(OH)3 is the predominant species in the pH range 5–9 based on the diagram of prevalence of hydroxo-aluminum complexes as a function of pH indicate (Hu et al., 2012; Lin et al., 2014). Accordingly, removal of TOC from aqueous solutions with initial pH 2.8–7.0 is carried out by the adsorption of PVP molecules on the surface of the amorphous hydroxide aluminum species through Van-Der-Waals interactions and hydrogen bonding (Cañizares et al., 2007; Mansouri et al., 2012). It can be argued that the increase of pH was due to the reduction of (excess) H+ ions at the cathode to dihydrogen (H2), which limits the formation of hydroxide and hence hydroxoaluminum species. Many researchers indicate that the removal of organic matter by electrocoagulation at this pH interval occurs due to coagulation by charge neutralization (Mollah et al., 2004; Shahedi et al., 2020; Hendaoui et al., 2021). Dissolved KHI reacts with Al3+ cations generated at the anode to form stable KHI–aluminum complexes (Mollah et al., 2004; Shahedi et al., 2020; Hendaoui et al., 2021).
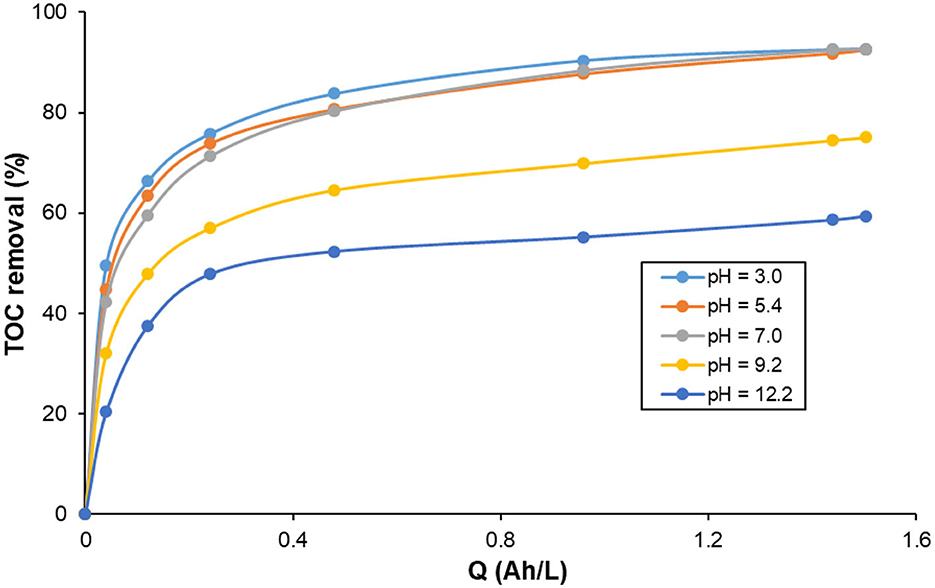
Figure 8. Influence of initial pH on the changes of TOC removal with specific electric charge during the treatment of 200 mg C/L KHI aqueous solutions by electrocoagulation using Al electrodes. Operating conditions: current density: 2.5 mA/cm2; supporting electrolyte 0.1 M NaCl; T = 25 °C.
The effect of the current density on the TOC removal during the treatment of KHI aqueous solutions containing 200 mg C/L in 0.1 M NaCl at pH 7.0 using Al-electrocoagulation process is illustrated in Figure 9. The profile of TOC removal with specific electrical charge is similar for all the current densities: a linear rapid increase with Q for Q < 1 Ah/L, after that the increase of TOC removal is decelerated, and then maintained to an almost constant value at the end of the experiment. The increase of current density from 1.25 mA/cm2 to 2.5 mA/cm2 increased the TOC removal from 71.7% to 92.6%, respectively. However, a further increase in the current density to 5.0 mA/cm2 decreased the TOC removal to 86.6%. It is expected that the increase of the current density produces larger amounts of Al3+ ions within the same period (Faraday's law), which is in accordance with the increase of TOC removal with the increase of the current density from 1.25 to 2.5 mA/cm2. Aluminum hydroxide formation increases with that increase in current density. These aluminum hydroxide flocs ultimately result in higher pollutant removal efficiency. However, at higher current densities than 2.5 mA/cm2, competitive secondary/parasitic reactions consume larger electrical energy, and then the amount of Al3+ ions released from the anode will not increase linearly with Q. This can explain the decrease in TOC removal when the current density increases from 2.5 to 5.0 mA/cm2. A higher current density than 2.5 mA/cm2 is not recommended owing to the high consumption of electrical energy, which increases the costs of Al-electrocoagulation process.
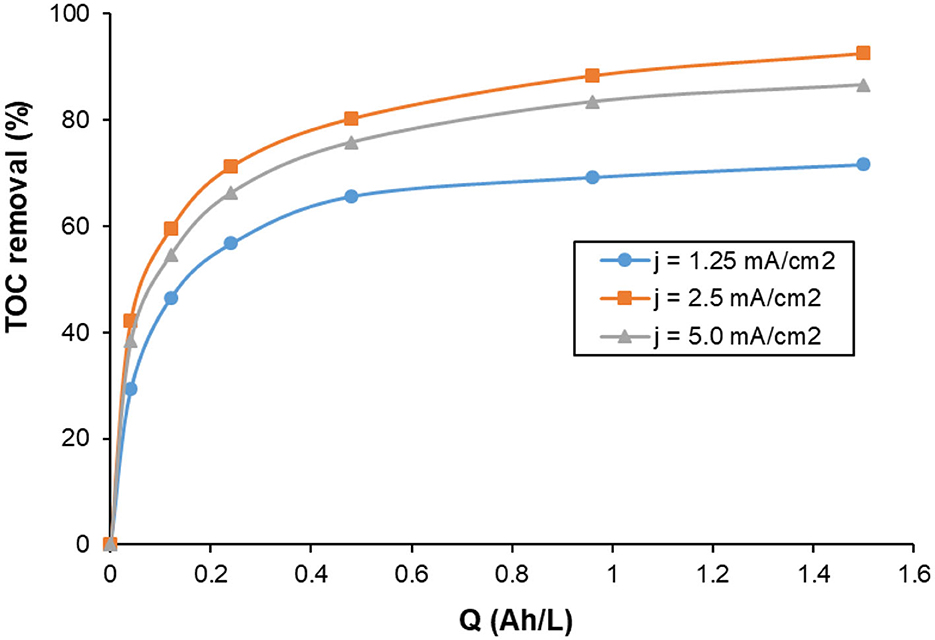
Figure 9. Influence of current density on the changes of TOC with specific electric charge during the treatment of 200 mg/L KHI aqueous solutions by electrocoagulation using Al electrodes. Operating conditions: initial pH = 7.0; supporting electrolyte 0.1 M NaCl; T = 25°C.
Figure 10 presents the effect of initial TOC content on the changes of TOC removal with specific electric charge during the treatment of KHI 0.1 M NaCl aqueous solutions at pH 7.0 by electrocoagulation using Al electrodes at 2.5 mA/cm2. The results showed that the TOC removal decreased with the increase in the initial TOC content. An almost complete TOC removal (99.1%) was obtained with the lowest initial TOC content of 120 mg C/L yielded.
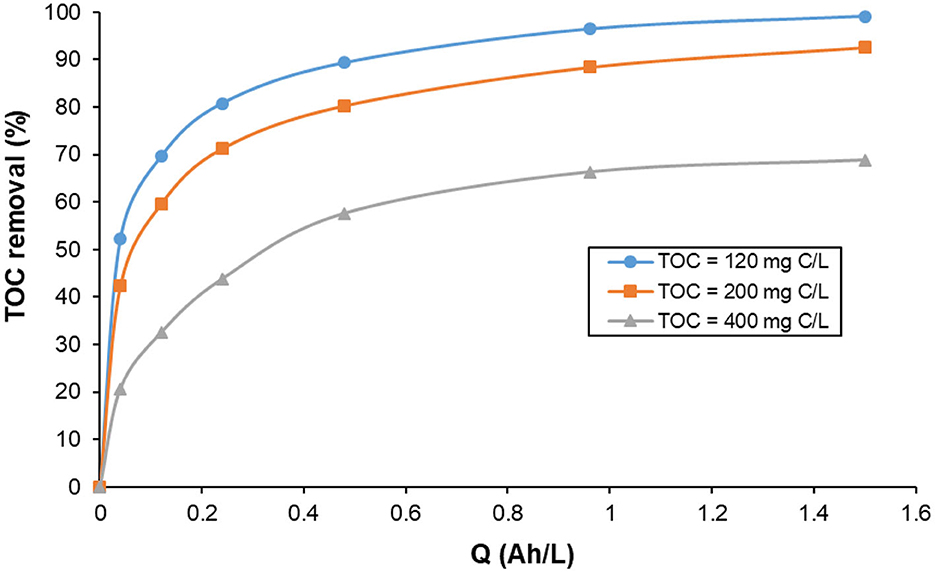
Figure 10. Influence of initial TOC content on the changes of TOC removal with specific electric charge during the treatment of KHI aqueous solutions by electrocoagulation using Al electrodes. Operating conditions: current density: 2.5 mA/cm2; initial pH = 7.0, supporting electrolyte 0.1 M NaCl; T = 25°C.
These results demonstrated the dependence of TOC removal with the amount of Al dissolved during the electrolysis. At a constant current density, the same amount of aluminum is released by anodic dissolution in the aqueous solutions containing different initial TOC content. The capacity of hydroxo-aluminum species to adsorb or bind with PVP molecules is limited by the amount and the form of these species, which is in favor of complete TOC removal for low initial TOC content. This confirms that the mechanism of PVP/KHI removal from water involves mainly adsorption of PVP molecules on the surface of Al(OH)3 flocs and their enmeshment inside the solid coagulants (see Figure 11).
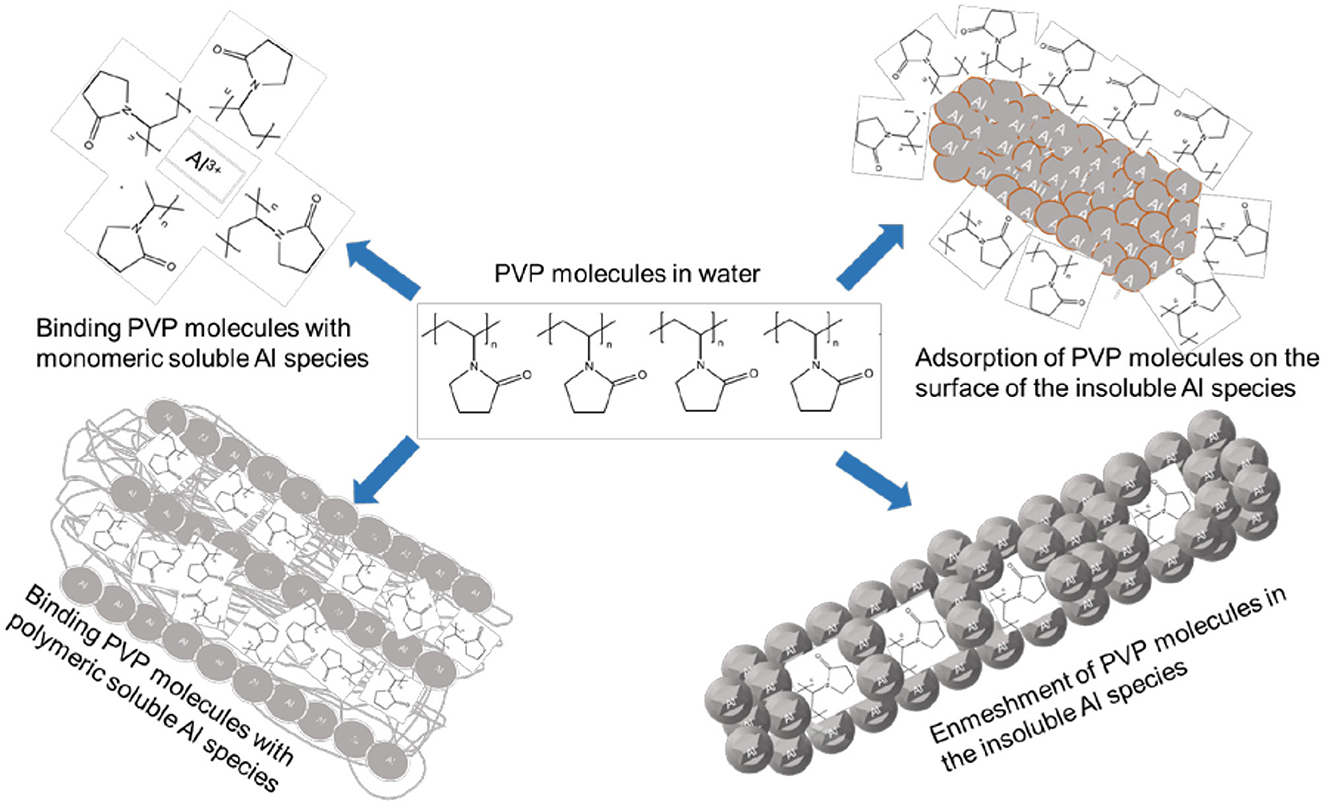
Figure 11. Proposed routes involved in the mechanism of removal of PVCap (KHI) by Fe-electrocoagulation.
4 Conclusion
The main conclusions of this work can be summarized as follows:
- Electrocoagulation was successfully used to remove KHI (polyvinyl pyrrolidone) from water using aluminum electrodes. The high efficiency of this technology is closely related to the amounts of coagulants formed during galvanostatic electrolysis.
- The anodic dissolution of aluminum during galvanostatic electrolysis is mainly affected by the pH conditions and the nature of the supporting electrolyte. During galvanostatic electrolyses using aluminum electrodes, electrochemical and chemical dissolution processes are involved in the generation of aluminum coagulants in solution. Particularly, the pH conditions affected the chemical dissolution of aluminum in solution.
- The dissolution of aluminum by pitting corrosion is accelerated by chloride ions in water. However, a thick passive layer of aluminum hydroxide/phosphate on the aluminum surface, which inhibits further dissolution of aluminum.
- The electrocoagulation process using aluminum electrodes under optimized conditions can eliminate high amounts of TOC content from aqueous solutions of polyvinyl pyrrolidone/KHI.
- The mechanism of removal polyvinyl pyrrolidone (KHI) molecules from water using Al-electrocoagulation involved different routes depending on pH of the medium. These routes include (i) Binding PVP molecules with monomeric soluble Al species, (ii) Binding PVP molecules with polymeric soluble Al species, (iii) Adsorption of PVP molecules on the surface of the insoluble aluminum species, and (iv) Enmeshment of PVP molecules in the insoluble Al species. This study achieved the goal of minimizing the energy requirements in electrochemical coagulation process using Al anodes through the optimization of the experimental conditions.
- The results of this study prove that electrocoagulation using sacrificial Al electrodes is a sustainable technique capable to remove KHI (PVP) from oil and gas-processing wastewaters, recycle the treated water in other industrial applications, and avoid the damage of water reservoirs.
Data availability statement
The raw data supporting the conclusions of this article will be made available by the authors, without undue reservation.
Author contributions
JA-M: Formal analysis, Investigation, Methodology, Writing—original draft. AA: Investigation, Methodology, Writing—original draft. MA: Data curation, Formal analysis, Investigation, Supervision, Writing—original draft. NB: Conceptualization, Data curation, Methodology, Project administration, Resources, Supervision, Validation, Writing—review & editing.
Funding
The author(s) declare that no financial support was received for the research, authorship, and/or publication of this article.
Conflict of interest
The authors declare that the research was conducted in the absence of any commercial or financial relationships that could be construed as a potential conflict of interest.
Publisher's note
All claims expressed in this article are solely those of the authors and do not necessarily represent those of their affiliated organizations, or those of the publisher, the editors and the reviewers. Any product that may be evaluated in this article, or claim that may be made by its manufacturer, is not guaranteed or endorsed by the publisher.
References
Abdulrazzaq, N. N., Al-Sabbagh, B. H., and Shanshool, H. A. (2021). Coupling of electrocoagulation and microflotation for the removal of textile dyes from aqueous solutions. J. Water Process Eng. 40, 101906. doi: 10.1016/j.jwpe.2020.101906
Acharya, S., Sharma, S. K., and Khandegar, V. (2022). “Electrolyte role in electrocoagulation process for nitrates removal from groundwater,” in Advances in Chemical, Bio and Environmental Engineering. CHEMBIOEN 2021. Environmental Science and Engineering, eds J. K. Ratan, D. Sahu, N. N. Pandhare, and A. Bhavanam (Cham: Springer). doi: 10.1007/978-3-030-96554-9_17
Adham, S., Gharfeh, S., Hussain, A., Minier-Matar, J., and Janson, A. (2014). “Kinetic hydrate inhibitor removal by physical, chemical and biological processes,” in Paper Presented at the Offshore Technology Conference-Asia (Kuala Lumpur). doi: 10.4043/24807-MS
Akter, S., Suhan, M. B. K., and Islam, M. S. (2022). Recent advances and perspective of electrocoagulation in the treatment of wastewater: a review. Environm. Nanotechnol. Monitor. Manage. 17, 100643. doi: 10.1016/j.enmm.2022.100643
AlJaberi, F. Y., Alardhi, S. M., Ahmed, S. A., Salman, A. D., Juzsakova, T., Cretescu, I., et al. (2022). Can electrocoagulation technology be integrated with wastewater treatment systems to improve treatment efficiency? Environ. Res. 214, 113890. doi: 10.1016/j.envres.2022.113890
Al-Qodah, Z., and Al-Shannag, M. (2017). Heavy metal ions removal from wastewater using electrocoagulation processes: a comprehensive review. Separat. Sci. Technol. (Philadelphia). 52, 2649–2676. doi: 10.1080/01496395.2017.1373677
Anderson, R., Tohidi, F., Mozaffar, H., and Tohidi, B. (2016). Kinetic hydrate inhibitor removal from produced waters by solvent extraction. J. Pet. Sci. Eng 145. doi: 10.1016/j.petrol.2016.06.016
Ansari, K., Shrikhande, A., Malik, M. A., Alahmadi, A. A., Alwetaishi, M., Alzaed, A. N., et al. (2022). Optimization and operational analysis of domestic greywater treatment by electrocoagulation filtration using response surface methodology. Sustain 14, 15230. doi: 10.3390/su142215230
Ansari, K., and Shrikhande, A. N. (2022). Impact of different mode of electrode connection on performance of hybrid electrocoagulation unit treating greywater. Lecture Notes in Civil Engineering. 172, 45. doi: 10.1007/978-981-16-4396-5_45
Asaithambi, P., Govindarajan, R., Yesuf, M. B., Selvakumar, P., and Alemayehu, E. (2021). Investigation of direct and alternating current-electrocoagulation process for the treatment of distillery industrial effluent: studies on operating parameters. J. Environ. Chem. Eng 9, 104811. doi: 10.1016/j.jece.2020.104811
Bani-Melhem, K., Al-Kilani, M. R., and Tawalbeh, M. (2023). Evaluation of scrap metallic waste electrode materials for the application in electrocoagulation treatment of wastewater. Chemosphere 310, 136668. doi: 10.1016/j.chemosphere.2022.136668
Bensalah, N. (2011). Removal of natural organic matter from wastewater by electrocoagulation using aluminum electrodes. Qatar Found. Annu. Res. Forum Proc. doi: 10.5339/qfarf.2011.evp17
Biswas, B., and Goel, S. (2022). Electrocoagulation and electrooxidation technologies for pesticide removal from water or wastewater: a review. Chemosphere 302, 134709. doi: 10.1016/j.chemosphere.2022.134709
Cañizares, P., Jiménez, C., Martínez, F., Sáez, C., and Rodrigo, M. A. (2007). Study of the electrocoagulation process using aluminum and iron electrodes. Ind. Eng. Chem. Res. 46, 19. doi: 10.1021/ie070059f
Dehghan, P., Abbasi, M., Mofarahi, M., and Azari, A. (2021). Adsorption of synthetic and real kinetic hydrate inhibitors (KHI) wastewaters on activated carbon: adsorption kinetics, isotherms, and optimized conditions. Sep. Sci. Technol 56, 1821220. doi: 10.1080/01496395.2020.1821220
Ebba, M., Asaithambi, P., and Alemayehu, E. (2021). Investigation on operating parameters and cost using an electrocoagulation process for wastewater treatment. Appl. Water Sci. 11, 11. doi: 10.1007/s13201-021-01517-y
Elkhatib, D., Oyanedel-Craver, V., and Carissimi, E. (2021). Electrocoagulation applied for the removal of microplastics from wastewater treatment facilities. Sep. Purif. Technol. 276, 118877. doi: 10.1016/j.seppur.2021.118877
Ghernaout, D. (2018). Electrocoagulation process: achievements and green perspectives. Colloid Surf. Sci 3, 11. doi: 10.11648/j.css.20180301.11
Ghernaout, D. (2019). Electrocoagulation process for microalgal biotechnology-a review. Rev. Appl. Eng 3, 85–94. doi: 10.11648/j.ae.20190302.12
Golpour, M., and Pakizeh, M. (2017). Development of a new nanofiltration membrane for removal of kinetic hydrate inhibitor from water. Sep. Purif. Technol. 183, 11. doi: 10.1016/j.seppur.2017.04.011
Hendaoui, K., Trabelsi-Ayadi, M., and Ayari, F. (2021). Optimization and mechanisms analysis of indigo dye removal using continuous electrocoagulation. Chinese J. Chem. Eng 29, 65. doi: 10.1016/j.cjche.2020.07.065
Hu, C., Liu, H., Chen, G., and Qu, J. (2012). Effect of aluminum speciation on arsenic removal during coagulation process. Sep. Purif. Technol. 86, 17. doi: 10.1016/j.seppur.2011.10.017
Hu, Y., Zhou, L., Zhu, J., and Gao, J. (2023). Efficient removal of polyamide particles from wastewater by electrocoagulation. J. Water Process Eng. 51, 103417. doi: 10.1016/j.jwpe.2022.103417
Idusuyi, N., Ajide, O. O., Abu, R., Okewole, O. A., and Ibiyemi, O. O. (2022). Low cost electrocoagulation process for treatment of contaminated water using aluminium electrodes from recycled cans. Mater. Today Proc. 56, 352. doi: 10.1016/j.matpr.2021.10.352
Jovanović, T., Velinov, N., Petrović, M., Najdanović, S., Bojić, D., Radović, M., et al. (2021). Mechanism of the electrocoagulation process and its application for treatment of wastewater: a review. Adv. Technol 10, 1. doi: 10.5937/savteh2101063J
Khadir, A., Negarestani, M., and Motamedi, M. (2021). Optimization of an electrocoagulation unit for purification of ibuprofen from drinking water: effect of conditions and linear/non-linear isotherm study. Sep. Sci. Technol 56, 1770795. doi: 10.1080/01496395.2020.1770795
Li, B., Lu, Y. Y., Li, Y., and Le, Y. (2022). A review of natural gas hydrate formation with amino acids. J. Marine Sci. Eng. 10, 1134. doi: 10.3390/jmse10081134
Li, Z., Liao, K., Qin, H. B., Chen, J., Ren, L., Li, F., et al. (2019). The gas-adsorption mechanism of kinetic hydrate inhibitors. AIChE J. 65, 16681. doi: 10.1002/aic.16681
Lim, V. W. S., Metaxas, P. J., Stanwix, P. L., Johns, M. L., Haandrikman, G., Crosby, D., et al. (2020). Gas hydrate formation probability and growth rate as a function of kinetic hydrate inhibitor (KHI) concentration. Chem. Eng. J. 388, 124177. doi: 10.1016/j.cej.2020.124177
Lin, J. L., Huang, C., Dempsey, B., and Hu, J. Y. (2014). Fate of hydrolyzed al species in humic acid coagulation. Water Res. 56, 4. doi: 10.1016/j.watres.2014.03.004
Lu, J., Zhuo, Q., Ren, X., Qiu, Y., Li, Y., Chen, Z., et al. (2022). Treatment of wastewater from adhesive-producing industries by electrocoagulation and electrochemical oxidation. Process Saf. Environ. Prot. 157, 35. doi: 10.1016/j.psep.2021.10.035
Lucakova, S., Branyikova, I., Kovacikova, S., Pivokonsky, M., Filipenska, M., Branyik, T., et al. (2021). Electrocoagulation reduces harvesting costs for microalgae. Bioresour. Technol. 323, 124606. doi: 10.1016/j.biortech.2020.124606
Mamelkina, M. A., Vasilyev, F., Tuunila, R., Sillanpää, M., and Häkkinen, A. (2019). Investigation of the parameters affecting the treatment of mining waters by electrocoagulation. J. Water Process Eng. 32, 100929. doi: 10.1016/j.jwpe.2019.100929
Mansouri, K., Elsaid, K., Bedoui, A., Bensalah, N., and Abdel-Wahab, A. (2011a). Application of electrochemically dissolved iron in the removal of tannic acid from water. Chem. Eng. J. 172, 970–976. doi: 10.1016/j.cej.2011.07.009
Mansouri, K., Hannachi, A., Abdel-Wahab, A., and Bensalah, N. (2012). Electrochemically dissolved aluminum coagulants for the removal of natural organic matter from synthetic and real industrial wastewaters. Ind. Eng. Chem. Res. 44, 4178–4185. doi: 10.1021/ie202188m
Mansouri, K., Ibrik, K., Bensalah, N., and Abdel-Wahab, A. (2011b). Anodic dissolution of pure aluminum during electrocoagulation process: influence of supporting electrolyte, initial PH, and current density. Ind. Eng. Chem. Res. 50, 13362–13372. doi: 10.1021/ie201206d
Marmanis, D., Thysiadou, A., Diamantis, V., Christoforidis, A., and Dermentzis, K. (2021). Performance of electrocoagulation processes for the removal of COD and ammonia from high salinity landfill-leachate using iron or aluminum electrodes. J. Eng. Sci. Technol. Rev 14, 14. doi: 10.25103/jestr.144.14
Medina-Collana, J. T., Reyna-Mendoza, G. E., Montaño-Pisfil, J. A., Rosales-Huamani, J. A., Franco-Gonzales, E. J., Córdova García, X., et al. (2023). Evaluation of the performance of the electrocoagulation process for the removal of water hardness. Sustain 15, 590. doi: 10.3390/su15010590
Mollah, M. Y. A., Morkovsky, P., Gomes, J. A. G., Kesmez, M., Parga, J., Cocke, D. L., et al. (2004). Fundamentals, present and future perspectives of electrocoagulation. J. Hazard. Mater 114, 1–3. doi: 10.1016/j.jhazmat.2004.08.009
Mollah, M. Y. A., Schennach, R., Parga, J. R., and Cocke, D. L. (2001). Electrocoagulation (EC)- science and applications. J. Hazard. Mater 84, 5. doi: 10.1016/S0304-3894(01)00176-5
Morales-Figueroa, A., Teutli-Sequeira, E. A., Linares-Hernández, I., Martínez-Miranda, V., García-Morales, M. A., Roa-Morales, G., et al. (2022). Optimization of the electrocoagulation process with aluminum electrodes for rainwater treatment. Front. Environ. Sci. 10, 860011. doi: 10.3389/fenvs.2022.860011
Mozaffar, H., Larsen, T., Henderson, C., Deshmukh, S., Anderson, R., Hoopanah, M., et al. (2022). Multiple Recovery and Re-Use of Commercial Kinetic Hydrate Inhibitors from Produced Water and Rich Glycol.
Orescanin, V., Kollar, R., Nad, K., Halkijevic, I., Kuspilic, M., Findri Gustek, S., et al. (2014). Removal of arsenic, phosphates and ammonia from well water using electrochemical/chemical methods and advanced oxidation: a pilot plant approach. J. Environ. Sci. Heal. - Part A Toxic/Hazardous Subst. Environ. Eng. 49, 1007–14. doi: 10.1080/10934529.2014.894843
Othmani, A., Kadier, A., Singh, R., Igwegbe, C. A., Bouzid, M., Aquatar, M. O., et al. (2022). A comprehensive review on green perspectives of electrocoagulation integrated with advanced processes for effective pollutants removal from water environment. Environ. Res 215. doi: 10.1016/j.envres.2022.114294
Rebai, N., Hadjadj, A., Benmounah, A., Berrouk, A. S., and Boualleg, S. M. (2019). Prediction of natural gas hydrates formation using a combination of thermodynamic and neural network modeling. J. Pet. Sci. Eng. 182, 106270. doi: 10.1016/j.petrol.2019.106270
Rodriguez, J., Stopi,ć, S., Krause, G., and Friedrich, B. (2007). Feasibility assessment of electrocoagulation towards a new sustainable wastewater treatment. Environ. Sci. Pollut. Res 14, 424. doi: 10.1065/espr2007.05.424
Romani, M., Espinoza-Quinõnes, F. R., Módenes, A. N., and Borba, C. E. (2020). new insights into the improvement of electrocoagulation performance on the basis of a time-integrated performance index: the pivotal role of electrical conductivity. J. Environ. Chem. Eng. 8, 103902. doi: 10.1016/j.jece.2020.103902
Rookesh, T., Samaei, M. R., Yousefinejad, S., Hashemi, H., Derakhshan, Z., Abbasi, F., et al. (2022). Investigating the electrocoagulation treatment of landfill leachate by iron/graphite electrodes: process parameters and efficacy assessment. Water (Switzerland) 14, 205. doi: 10.3390/w14020205
Saberi, A., Alamdari, A., Rasoolzadeh, A., and Mohammadi, A. H. (2021). Insights into kinetic inhibition effects of MEG, PVP, and L-tyrosine aqueous solutions on natural gas hydrate formation. Pet. Sci 18, 515. doi: 10.1007/s12182-020-00515-0
Sayani, J. K. S., Pedapati, S. R., and Lal, B. (2020). Phase behavior study on gas hydrates formation in gas dominant multiphase pipelines with crude oil and high CO2 mixed gas. Sci. Rep 10, 6. doi: 10.1038/s41598-020-71509-6
Shahedi, A., Darban, A. K., Taghipour, F., and Jamshidi-Zanjani, A. (2020). A review on industrial wastewater treatment via electrocoagulation processes. Curr. Opini. Electrochem. 22, 154–169. doi: 10.1016/j.coelec.2020.05.009
Sillanpää, M., and Shestakova, M. (2017). Electrochemical Water Treatment Methods: Fundamentals, Methods and Full Scale Applications. Elsevier.
Tchamango, S. R., Kamdoum, O., Donfack, D., and Babale, D. (2018). Comparison of electrocoagulation and chemical coagulation processes in the treatment of an effluent of a textile factory. J. Appl. Sci. Environ. Manag. 21, 17. doi: 10.4314/jasem.v21i7.17
Trompette, J. L., and Lahitte, J. F. (2021). Effects of some ion-specific properties in the electrocoagulation process with aluminum electrodes. Colloids surfaces a physicochem. Eng. Asp 629, 127507. doi: 10.1016/j.colsurfa.2021.127507
Veluswamy, H. P., and Linga, P. (2021). Natural gas hydrate formation using saline/seawater for gas storage application. Energy and Fuels 35, 7. doi: 10.1021/acs.energyfuels.1c00399
Villalobos-Lara, A. D., Álvarez, F., Gamiño-Arroyo, Z., Navarro, R., Peralta-Hernández, J. M., Fuentes, R., et al. (2021). Electrocoagulation treatment of industrial tannery wastewater employing a modified rotating cylinder electrode reactor. Chemosphere 264, 128491. doi: 10.1016/j.chemosphere.2020.128491
Wang, Y., Fan, S., and Lang, X. (2019). Reviews of gas hydrate inhibitors in gas-dominant pipelines and application of kinetic hydrate inhibitors in China. Chin. J. Chem. Eng. 29, 2118–2132. doi: 10.1016/j.cjche.2019.02.023
Xu, L. Q., Liu, S. L., Xu, X. J., and Cao, G. Z. (2017). Passivation Mechanism of Electrocoagulation Process in Removing Heavy Metals from Wastewater. Waltham: Xiandai Huagong/Modern Chemical Industry.
Keywords: electrocoagulation, sacrificial electrodes, potentiodynamic polarization, anodic dissolution, kinetic hydrate inhibitor
Citation: Al-Marri JS, Abouedwan AB, Ahmad MI and Bensalah N (2023) Electrocoagulation using aluminum electrodes as a sustainable and economic method for the removal of kinetic hydrate inhibitor (polyvinyl pyrrolidone) from produced wastewaters. Front. Water 5:1305347. doi: 10.3389/frwa.2023.1305347
Received: 01 October 2023; Accepted: 27 November 2023;
Published: 14 December 2023.
Edited by:
Amin Mojiri, Hiroshima University, JapanReviewed by:
Khalid Ansari, Yeshwantrao Chavan College of Engineering (YCCE), IndiaNediljka Vukojević Medvidović, University of Split, Croatia
Copyright © 2023 Al-Marri, Abouedwan, Ahmad and Bensalah. This is an open-access article distributed under the terms of the Creative Commons Attribution License (CC BY). The use, distribution or reproduction in other forums is permitted, provided the original author(s) and the copyright owner(s) are credited and that the original publication in this journal is cited, in accordance with accepted academic practice. No use, distribution or reproduction is permitted which does not comply with these terms.
*Correspondence: Nasr Bensalah, bmFzci5iZW5zYWxhaCYjeDAwMDQwO3F1LmVkdS5xYQ==