- 1Office of Science and Engineering Laboratories, Center for Devices and Radiological Health, US Food and Drug Administration, Silver Spring, MD, United States
- 2Office of Product Evaluation, Center for Devices and Radiological Health, US Food and Drug Administration, Silver Spring, MD, United States
Aerosols inadvertently generated by the bubbling of contaminated water in heater cooler devices (HCDs) have been associated with hundreds of patient infections. These aerosols are typically generated by bubbling within the water tanks of HCDs that subsequently escape from the device leading to contamination of the operating room and potentially infect patients undergoing cardiothoracic surgery. Although these infections are now well-reported in literature, very little research has been done to understand the influence of bubbling frequencies, differences in aerosolization across various species of bacteria, sampling methodologies, infectious dose, etc. Here we report on the development of a miniaturized bubbler with a footprint much smaller than HCDs, that will enable academic researchers and HCD developers to investigate these parameters. Using this bubbler, we found that the aerosolization potential of slow growing Mycobacterium chimaera is about one hundred-fold more (p < 0.05) compared to rapid growing M. smegmatis for low inoculum concentrations (≤106 CFU/mL), underscoring the need for using clinically relevant M. chimaera for evaluating the aerosolization potential of nontuberculous mycobacteria (NTM) from HCDs.
Introduction
Heater-cooler devices (HCDs) commonly include water-based heat-exchange technology, used for thermoregulation of patients perioperatively, including cardiothoracic surgeries. While the water does not come into direct contact with the patient during procedures, these devices have been linked to patient infections, many identified as Mycobacterium chimaera, through aerosolization of bacteria from the HCD into the operating theater (Cheng et al., 2016; Falkinham, 2016; Garvey et al., 2016; Chand et al., 2017; Kanamori et al., 2017; U. S. Food and Drug Administration, 2021a). Despite the risk of patient infections, the benefits of thermoregulation during cardiothoracic surgeries necessitate their continued use.
Between January 2010 and October 2020, there have been 1,635 medical device reports (MDRs) submitted to the FDA related to infections and device contamination associated with HCDs from various manufacturers. Of these, 475 MDRs reported patient infections and 1,160 reported device contaminations without known patient infection. Since each MDR can have multiple infections reported, or multiple MDRs can report the same infection, further data analysis was conducted. This revealed that in the MDRs there were at least 300 patient infections and at least 64 patient deaths associated with HCDs. Nontuberculous mycobacteria (NTM) infections are difficult to diagnose and treat due to the late onset of non-specific symptoms, slow rates of bacterial growth and manifestation of disease, and high rates of antimicrobial resistance (Phillips and von Reyn, 2001; Falkinham, 2003; Brown-Elliott et al., 2012; Weeks et al., 2020). The prevalence of infections, and their severity has led to FDA intervention through several safety communications and interactions with manufacturers (U. S. Food and Drug Administration, 2021a,b), and collaboration with the CDC.
Bio-aerosols can be inadvertently generated from contaminated water in HCDs (U. S. Food and Drug Administration, 2021a) potentially leading to infections of patients undergoing cardiothoracic surgery. FDA's detailed investigation has determined this to be a multi-step process—water used in HCDs can become contaminated; the circulating water pumps inside the devices inadvertently create bubbles by cavitation; hydrophobic NTM have the propensity to encapsulate these bubbles in very high concentrations; these bubbles carry the NTM to the top of the air water interface in the water tanks, and then pop to generate smaller aerosols which can leak through vents or crevices in the top of the tank; fans used for cooling the electronics inside the tanks can, through convection, carry NTM laden aerosols toward the operating table and eventually infect patients undergoing cardiothoracic surgery.
Inadvertent patient infections through aerosolization in HCDs has also garnered some attention in the medical community with over 100 publications (Web of science V5.35, Clarivate Inc.) on this topic since the first FDA communication on risks of patient infections in 2015 (U. S. Food and Drug Administration, 2020). While it is now well-known that NTM aerosols from contaminated water in the tanks of HCDs resulted in the patient infections, in vitro aerosolization studies have rarely employed NTM species and bubbling is rarely used to produce such aerosols. Several research questions therefore remain unanswered: are there differences in aerosolization potential across different NTM species (here aerosolization potential is defined as the potential for one unit of NTM to aerosolize for every unit of NTM in solution); what sampling methodologies are the most appropriate for assaying the airborne NTM and how sensitive would the biological assays be; are there differences in the survivability of various NTM species depending on how they aerosolized; how does bubbling compare with other more common laboratory based methods of aerosolization (e.g., atomization). Addressing such questions using actual HCDs would not only be logistically challenging due to the required precautions necessary for work with risk group (RG-2) microorganisms, the logistics of using large volumes (heater-coolers often require 10–14 Liters of fluids), but also time consuming. Getting the answers to most of these questions would require a concerted community effort as pointed out elsewhere (Weeks et al., 2020).
M. smegmatis is often used in academic laboratory settings as a model system to conduct research for M. tuberculosis and other NTMs (Mohan et al., 2015). The objective of this paper is to determine if M. smegmatis can be used to substitute M. chimaera in aerosolization testing by comparing the aerosolization potential of rapid growing, non-pathogenic M. smegmatis with the slow growing opportunistic pathogen M. chimaera that is known to have caused fatal patient infections. To our knowledge, previous studies have not investigated this aspect.
Materials and Methods
Selection of NTM Species
Rapid-growing NTM like M. smegmatis grow in 3–5 days compared to 4–6 weeks for slow-growing NTM like M. chimaera. M. smegmatis is classified as a low-Risk Group (RG-1) organism (ATCC, 2022), while M. chimaera is an opportunistic pathogen (RG-2) (DSMZ, 2022). While aerosolization experiments for both require safety precautions, experiments with M. smegmatis pose a lower risk to the experimenter. Thus, choosing M. smegmatis would enable a device manufacturer to evaluate aerosolization risks of their devices in about 1–2 weeks (including growth, inoculation, aerosolization and then enumeration) compared to 8–12 weeks with M. chimaera. However, for HCD-related infections, M. chimaera has been the organism of concern due to the frequency of reported patient infections (European Centre for Disease Prevention Control, 2015; Kohler et al., 2015; Sax et al., 2015). In addition, the higher hydrophobicity (Phillips and von Reyn, 2001) and the larger cell size of M. smegmatis could either result in the individual cells aggregating in the water leaving fewer cells to aerosolize, and/or the large aggregates of M. smegmatis aerosols generated may settle under gravity leaving fewer ones to sample from the air. As a result, during device testing, the extent of aerosolization of M. smegmatis may not reflect the true risk of a device design to aerosolize M. chimaera (Weeks et al., 2020). Therefore, a comparative study was conducted with both organisms where the relative potential of both species was compared.
Strains and Culture Conditions
M. smegmatis (ATCC 19420; type strain) was purchased from the American Type Culture Collection (Manassas, VA). M. chimaera (ND HCU 2016-20-02; isolate from a heater cooler) was received from the Centers for Disease Control and Prevention (CDC; Atlanta, GA). Cultures grown on agar plates were cultured on Middlebrook 7H10 (Remel R453982; M7H10) supplemented with 10% OADC Enrichment Solution (Remel R450605), 0.5% glycerine, and 0.1% Pourite Anti-Bubble (Aurical Company 8500). M7H10 agar plates were incubated at 30°C for 3–7 days for M. smegmatis or 14–28 days for M. chimaera. Liquid cultures of M. smegmatis were prepared by suspending a single colony from 7H10 agar plates in Middlebrook 7H9 (Remel R454012; M7H9) supplemented with 10% OADC, 0.1% Tween 80 (Sigma P1754-500ML) with aeration to stationary phase (7 days) (Islam et al., 2013). Liquid cultures of M. chimaera were prepared by suspending a single colony from 7H10 agar plates in M7H9 supplemented with 10% OADC at 30°C with aeration to stationary phase (~21 days). Liquid cultures were grown in a Innova 44 (New Brunswick) incubator shaker with mechanical aeration (150 RPM).
Aerosolization Inoculum Preparation
Fresh cultures for both M. smegmatis and M. chimaera were prepared by diluting stationary phase cells 1:100 and sub-culturing to an optical density (OD550) of ~1.5. After reaching an OD550 of ~1.5, cultures were centrifuged at 5,000 RPM for 20 min at room temperature. The supernatant was decanted, and cells were washed twice before suspending to an OD550 of 1.0 in sterile deionized (DI) water. Prior to aerosolization experiments, cultures were enumerated by performing 10-fold serial dilutions and plating 100 μl aliquots on MB 7H10 agar plates. M. smegmatis correlated ~2 × 108 colony forming units (CFU)/ml for OD550 of 1.0. M. chimaera correlated ~4 x 108 CFU/ml for OD550 of 1.0. Bacterial suspensions in DI water at OD550 of 1.0 were used to inoculate 100 ml of autoclaved tap water to a final concentration of 105, 106, and 107 CFU/ml. To begin, 100 ml of 105 CFU/ml inoculum was bubbled with increasing flow rates from 0.2 to 12 L/min for 5 min at each flow rate. Samples of the inoculum were taken for enumeration of the inoculum post aerosolization and subsequently adjusted to a final concentration of 106 CFU/ml using water acclimated inoculum at OD550 of 1.0. The 106 CFU/ml inoculum was subjected to increasing flow rates from 0.2 to 12 L/min. Likewise, a sample of the inoculum was taken for enumeration post aerosolization. Finally, the 100 ml inoculum was adjusted to 107 CFU/ml using water acclimated inoculum at OD550 of 1.0. The 107 CFU/ml inoculum was subjected to increasing flow rates from 0.2 to 12 L/min. Following the 12 L/min airflow rate, a sample of the inoculum was taken for enumeration post aerosolization.
Additional experiments with lower concentrations of M. smegmatis were used as part of a feasibility study. We noted that concentrations of 107 CFU/ml were transparent while still being visible to the unaided eye. The 100 ml bacterial suspensions were inoculated into an in-house (FDA) custom-designed bubbler (Figure 1). Bacterial concentrations were also determined from the solution after aerosolization to assess changes to the bacterial population during aerosolization.
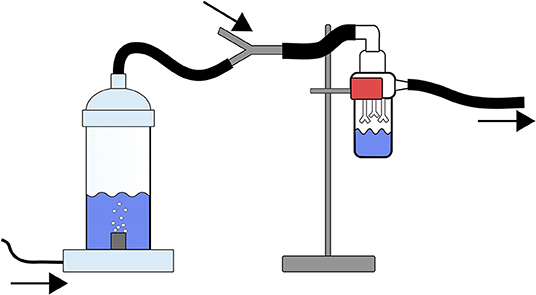
Figure 1. Schematic of the FDA Bubbler along with a biosampler used for characterizing the aerosolization potential of M. smegmatis and M. chimaera. The arrows depict airflow through the apparatus, tubing, and impinger, the airflow as depicted is traveling from left to right in the schematic. The tubing on the left side of the figure is connected to the house air and a mass-flow controller. This mass-flow controller is set to an intended flow rate (e.g., 0.2, 0.5, 2, or 12 L/min). The bacterial solution is placed into the bubbler prior to starting the airflow through the system. The bubbler is connected through tubing to an SKC biosampler (right side of the figure). The SKC biosampler is connected to a mass-flow controller set to 12.5 L/min. The tubing connecting the bubbler to the SKC biosampler is diverted through a y-joint tubing connector which allows for the make up airflow to pass to the SKC Biosampler.
Prior to aerosolization of the lowest inoculum concentration and after disinfection of the apparatus, negative control samples were tested to ensure that no microorganisms were recovered. For this, the apparatus was filled with 100 ml of autoclaved tap water and subjected to 12 L/min airflow for 5 min. The entire 20 ml sample recovered in the SKC biosampler was collected through vacuum filtration on 0.45 μm filters (Millipore) and recovered on M710 agar plates.
Apparatus Disinfection
After all flow rates and concentrations were tested, the apparatus and tubing were disinfected in order to obtain independent replicates. As NTM are known to exhibit high levels of resistance to chlorine-based disinfectants (Taylor et al., 2000), we elected to disinfect these items with a peracetic acid/hydrogen peroxide-based disinfectant/sterilant.
We noted that exposure to a 1% (v/v) Minncare Cold Sterilant (Mar Co. 176-01-002; 4.5% peracetic acid/22% hydrogen peroxide) solution (diluted in DI water) for 10 min was sufficient for M. smegmatis; however, when this was repeated with M. chimaera, we noted significant contamination in control samples after disinfection. Therefore, to disinfect the bubbler between replicates, the bubbler and all tubing were completely submerged in a 5% (v/v) Minncare solution for 30 min. The 5% (v/v) Minncare solution was prepared in facility DI water. After disinfection, each part of the apparatus and tubing were each thoroughly rinsed for 2–3 min in DI water.
Mimicking the Bubbling Process in HCDs
The mechanism of aerosolization has an impact on the quantity of aerosols generated as well as on the survivability and recovery of microorganisms (Heidelberg et al., 1997). While nebulization and humidification are the most popular means of aerosolization, bubbling has rarely been investigated (Weeks et al., 2020). Of the investigations reported, even fewer have used biological species, and have had complex set ups containing peristaltic pumps, multiple flow loops, and other complex parts (Heidelberg et al., 1997; Ulevicius et al., 1997; Maimelis et al., 2005; Simon et al., 2011). Our design has no pumps (Figure 1) and enables flow control through mass flow controllers. Suctioned and pressurized air can be used to create air flow using in-house vacuum and compressed air lines. The inlet and outlet flow rates were controlled using two Alicat mass flow controllers (Alicat Scientific, Tucson, AZ) with ranges of 0–20 LPM, and 0–50 LPM, respectively. The experiment was conducted inside a biosafety cabinet that had been previously disinfected using 70% ethanol. Because the inlet flow was smaller than the outlet flow, a supplementary air flow line was kept open that would suction in the required air.
A miniaturized chamber with dimensions of 51 mm internal diameter (64 mm outer diameter) and 145 mm height (160 mm to bottom of aerator plug-in) was created (Figure 1). An aquarium aerator was added to the air inlet at the bottom of the chamber to assist in bubble diffusion. A small tube would deliver filtered airflow through the bottom of the aerator. Four flow rates, 0.2, 0.5, 2.0, and 12.0 L/min were used through the aerator (16 mm outer diameter) to investigate the impact of bubbling frequency (i.e., mild vs. vigorous bubbling) which was controlled using an Alicat flow controller with a range of 0–20 L/min. At the top of the chamber, a conductive silicon tubing (TSI Inc. Shoreview, MN) would transport the aerosolized NTM to a SKC Biosampler (SKC Inc., PN 225-9595; Figure 1 impinger) that was maintained at 12.5 L/min. The air intake (Figure 1) would vary depending on the difference of flow rate through the aerator and the biosampler. The total footprint of the set up would easily fit into half of a 6' Class II Type 2A biosafety cabinet and is significantly less than the dimensions of a room that would be required to perform aerosolization testing with an actual HCD (Sommerstein et al., 2016). The cabinet was disinfected prior to and at the end of the experiments with 70 % ethanol. Conductive silicon tubing was also used to connect the biosampler with the suction line that hosted the downstream Alicat flow controller. To avoid cross contamination, the tubing was completely disinfected at the end of each experiment (Section Apparatus Disinfection).
Preliminary experiments were performed using a different bubbler design (Supplementary Table S1 and Supplementary Figure S1A). The original design included a square tank and two aerators. While we were able to recover aerosolized bacteria, the square shape resulted in areas where bacterial aggregates would accumulate due to non-uniform aeration of the solution. We also noted that at lower inlet airflows, aeration did not occur evenly through both aerators. We redesigned the bubbler to a smaller volume with a single aerator at the center of the tube. This allowed for even aeration of the solution and decreased dead spaces for bacterial accumulation (Supplementary Table S1).
Sampling Methodology and Enumeration
The SKC biosampler was filled with 20 ml of M7H9 supplemented with 10% OADC, 0.1% Tween 80, and 0.004% Antifoam B silicone emulsion (JT Baker, B531-05) that prevented froth formation particularly during collection of hydrophobic bacteria. The biosampler was chosen since it is known to be efficient in particle collection particularly compared to using other biosamplers or filters in which survivability decays significantly with increasing collection times (Kesavan et al., 2010; Ferguson et al., 2019). Aerosolized material was collected in the biosampler for 5 min. Impinged solution was transferred into 50 ml conical vials. Impinged solutions were vortexed for 30 s at 1,500 RPM (VWR) and 10-fold serial dilutions were prepared by diluting 1 ml of solution into 9 ml of phosphate buffered saline (Fisher). Samples were either collected by vacuum filtration onto 0.45 μm filters (Millipore) or by spread plating 100 μl onto M7H10 agar plates. Samples were plated as a single replicate at three different dilutions. This was to ensure that countable numbers were obtained for each sample.
Calculations of Aerosolized Particles
Colonies were calculated as the total number of aerosolized organisms by determining the concentration (CFU/ml) multiplied by a total of 20 ml of impinged solution. The average of 5 independent experiments was determined for M. smegmatis and the average of 3 independent experiments was determined for M. chimaera.
Statistical Analysis
Using the Shapiro-Wilks normality test using EXCEL (Microsoft Inc.) no significant departure from normality was found for the aerosol counts for M. smegmatis and M. chimaera at various NTM liquid concentrations as well as bubbler air flow rates. Unpaired student t-test was performed using EXCEL and then checked using Graphpad (Prism Inc.). Statistical significance was determined by setting α to 0.05. For operator-to-operator variability studied with M. smegmatis, the sample sizes for operators 1 and 2 were 3 replicates and 2 replicates, respectively. For subsequent comparison with M. chimaera for aerosolization potential, the data for M. smegmatis for both operators needed to be combined. Thus, for p-values sample sizes used were N = 3 for M. chimaera (performed by operator 2), and N = 5 for M. smegmatis (performed by operators 1 and 2).
Other Considerations
0.1% Tween 80 was added to M7H9 media when culturing M. smegmatis to ensure uniform growth and to reduce the formation of bacterial aggregates. Previous studies have demonstrated that inclusion of Tween 80 or other surfactants can assist in culture uniformity (Le Dantec et al., 2002; Islam et al., 2013). In pilot experiments with M. smegmatis, we noted that at bacterial concentrations >107 CFU/ml in water, bacteria would clump together and form dense bacterial film at the top of the solution (Supplementary Figure S1B). To prevent bacterial aggregation upon recovery, cells were recovered in M7H9 supplemented with 10% OADC, 0.1% Tween 80, and 0.004% Antifoam B. Antifoam B was included as the impinged solution formed significant amounts of foam due to the presence of both 10% OADC and 0.1% Tween 80. Separate experiments showed that the presence of Tween 80 and Antifoam B at these concentrations did not affect bacterial growth (data not shown).
To ensure our set up was safe for operators and it did not have any leaks we conducted a series of tests using M. smegmatis and poly-styrene latex beads (0.5 μm diameter; PSL) and monitored using biological and physical assays. We used BactiSwabs (Remel 12100/12110) to collect samples on the outside of the apparatus and attempted to grow any recovered M. smegmatis that may have been ejected outside of the system during the aerosolization experiments. No M. smegmatis was detected for any swabs outside of the solution or internal air pathway. For experiments with PSL beads (Polysciences Inc.), we used a particle counter (Model 3775, TSI Inc.) to assess whether small particles were leaking from the apparatus. Samples were taken from inside the biosafety cabinet, directly next to the chamber, lid, and tubbing connection joints, as well as directly sampling the number of aerosolized PSL beads being emitted into the tubing. We noted increased particles when we sampled the air from the bubbler without any tubing. However, once tubing was placed particle size and distributions were unchanged from background at all connection joints before, during and after the experiments implying no leaks from the set-up.
Results and Discussion
Aerosolization of M. smegmatis
We performed studies using M. smegmatis and the minimum bacterial concentration required for us to observe and quantify aerosolized bacteria. For this testing, we used bacterial concentrations from 10 to 107 CFU/ml and bubbled with a flow rate of 12 L/min (Supplementary Figure S1E). We noted that bacteria were recovered for all inoculum concentrations (Supplementary Figure S1E); however, quantifiable numbers (30–300 CFUs) of bacteria were only observed for concentrations from 104 to 107 CFU/ml. The number of recovered M. smegmatis colonies were low (average 86 CFU; range 39–156 CFU) for the 104 CFU/ml inoculum. We were concerned that this would lead to non-quantifiable recovery at lower flow rates. However, the recovery of NTM aerosols even with low inoculum concentrations underscores the importance of tight microbial control in the water of reusable HCDs.
Next, we wanted to determine the propensity for M. smegmatis to aerosolize at different air flow rates, and whether this aerosolization was reproducible across multiple operators. To this end, we repeated experiments with inoculum concentrations between 105 and 107 CFU/ml at 4 flow rates (0.2, 0.5, 2, and 12 L/min) in multiple replicates. These replicates were performed by two operators over multiple days. As noted in Supplementary Table S2, there were some variations between operators, however, generally these were not determined to be statistically significant variations.
For M. smegmatis we noted that as we increased inoculum concentrations, the amount of recovered M. smegmatis aerosols increased proportionally (Supplementary Table S2). Additionally, we also noted that as flow rate increased, the number of aerosolized M. smegmatis increased proportionally. Meaning if either inoculum concentration or flow rate increased 10-fold, the number of aerosolized M. smegmatis recovered increased by 10-fold.
Our trends are consistent with studies performed with polystyrene latex beads or E. coli using prior bubbler generators (Simon et al., 2011). Such studies also reported a linear increase in aerosol concentration with increase in air flow rate (Maimelis et al., 2005; Simon et al., 2011).
Surrogate Species Use May Not Be Appropriate
FDA allows for the use of surrogate microorganisms under several circumstances allowing device manufacturers to choose economically viable options in place of the organism of concern. However, in certain circumstances, such as addressing specific safety concerns related to HCDs, the significant inter-species variability in NTM (e.g., size, hydrophobicity) it is difficult to determine if M. smegmatis can be used for aerosolization potential studies in HCDs instead of slow growing M. chimaera (Weeks et al., 2020). To investigate this aspect under controlled circumstances, we performed a direct comparison of the number of aerosols generated by M. chimaera and M. smegmatis by our bubbler for the same bubbling flow rates, and inoculum concentrations.
We noted that M. chimaera exhibited higher numbers of recovered aerosolized bacteria at lower concentrations and flow rates (Figure 2 and Table 1) compared to M. smegmatis. Additionally, with increased inoculum concentration we noted that the number of recovered aerosolized M. chimaera increased, but unlike M. smegmatis, the increase was not proportional to the increase in inoculum. In other words, a 10-fold increase in inoculum did not result in a 10-fold increase in aerosolized M. chimaera. Plotting the amount of aerosolized M. chimaera and M. smegmatis recovered as a function of their concentration in water, also shows differences (Supplementary Figure S2). While M. smegmatis shows a linear increase in aerosolization at both 0.2 and 12 L/min, M. chimaera shows a logarithmic increase, with aerosolization potential reducing with increase in the concentration at low flow rates (Table 1). At higher flow rates, that corresponds to higher rate of bubbling, the aerosolization trends are also linear like M. smegmatis for M. chimaera. The reasoning behind this behavior requires further research.
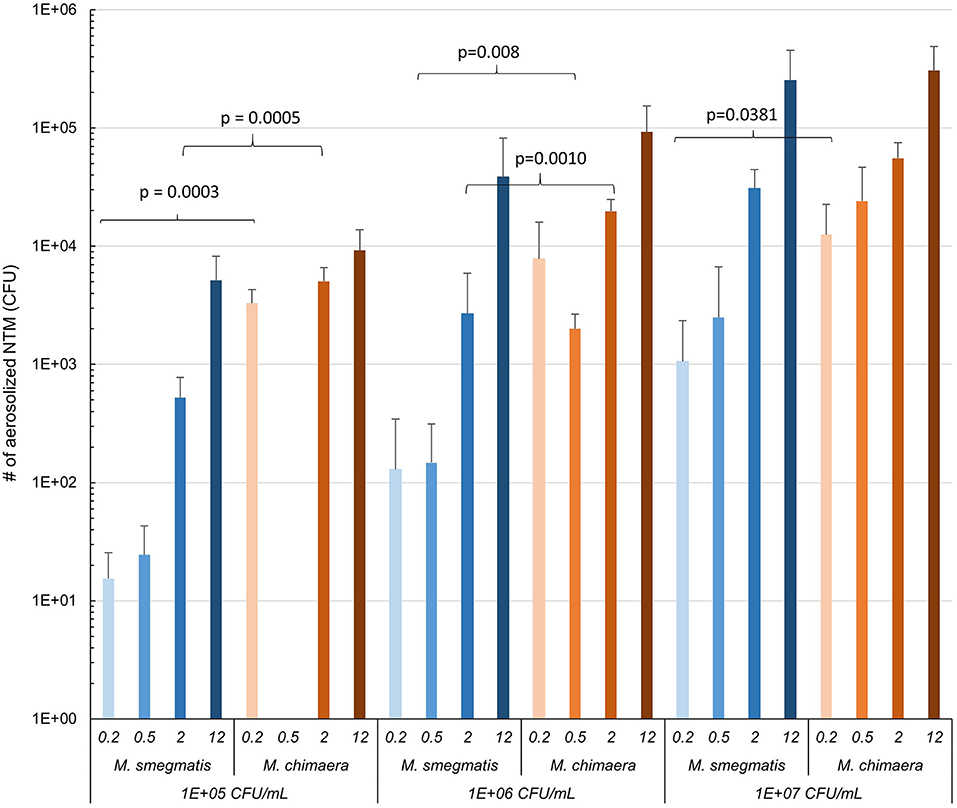
Figure 2. The mean and standard deviation of aerosolized M. smegmatis and M. chimaera as a function of our bubbler flow rate and concentration in liquid. Inter-species statistical comparison is provided in Table 1. For the 105 CFU/mL, Q = 0.5 L/min samples were contaminated and did not grow appropriately, leading to no information acquired for this data point.
To better appreciate the differences in aerosolization between M. chimaera and M. smegmatis, we determined the ratio of aerosols generated for both species with other conditions remaining the same (Table 1). For a flow rate of 0.2 L/min and an inoculum of 105-106 CFU/ml, we observed 100–200 times more M. chimaera recovered than M. smegmatis. Even at high concentrations (107 CFU/ml) we noted 11.9 times greater recovery of aerosolized M. chimaera.
As flow rate and inoculum concentration increased, differences between the two species were not statistically different (Figure 2). At flow rates of 12 L/min, while there were 1.2–2.4 times more aerosolized M. chimaera recovered, this was not determined to be statistically significant (p = 0.1797, p = 0.1887, and p = 0.7195 at 105, 106, and 107 CFU/mL, respectively).
At low inoculum concentrations of ≤ 106 CFU/mL, the relative potential of aerosolization in M. chimaera is 100–200-fold more than M. smegmatis. There could be two reasons behind this: (a) M. smegmatis tends to create large aggregates in solution (suggesting increased hydrophobicity) resulting in fewer free M. smegmatis in the solution that encapsulate each bubble as they rise. As these bubbles burst at the air water interface there are fewer M. smegmatis aerosols generated; (b) the relatively larger size of M. smegmatis (Vijay et al., 2017) compared to M. chimaera (SecureAire, 2022), and hence may be more prone to settling (as settling scales with mass of an aerosol, and mass scales with diameter for cylindrical shaped aerosols) and thus fewer get transported to the biosampler. While our studies did not directly measure hydrophobicity of the organisms, we noted significantly higher bacterial “foam” clumping at the air/water interface correlating (Supplementary Figure S1C) to decreased turbidity in solution for M. smegmatis than M. chimaera at high concentrations (i.e., above 107 CFU/ml). Additionally, M. smegmatis water acclimatized concentrated stock solutions (OD550 of 1.0) left unagitated was prone to aggregation.
For a fixed inoculum concentration, as bubbling flow rate increases (qualitatively characterized based on videos), the relative differences in aerosolization of M. chimaera and M. smegmatis also decreased. This is probably because more vigorous bubbling at the higher flow rates causes the aggregates for M. smegmatis to break up, increasing the concentration of free M. smegmatis in the solution which then get aerosolized in larger numbers.
As the inoculum concentration becomes high (107 CFU/mL), the difference in aerosolization potential appears to become statistically indistinguishable between the two species. This is probably because the increased concentration potentially leads to more aggregation of M. chimaera leaving fewer free in the solution to aerosolize. We noted that concentrations of 107 CFU/ml were transparent but visibly cloudy. It is not likely that such high concentrations (>107 CFU/ml) of NTM to be seen in devices as manufacturer's instructions for use direct hospitals to clean and disinfect HCDs prior to the water being visibly contaminated and FDA recommends the use of sterile or 0.22 μm filtered water to fill the device (U. S. Food and Drug Administration, 2021b). Such instructions and recommendations should reduce the potential for situations of uncontrolled microbial growth to emerge within the devices. Additional studies would further clarify the role of M. chimaera inoculum concentration on the aerosolization potential.
Limitations
Studies were performed only with two species and more strains will help better delineate the impact of various characteristics such as hydrophobicity, size etc. We did find some inter-operator differences and further interlaboratory studies may be desirable. It should also be pointed out since we did not directly perform experiments with actual heater cooler devices, extrapolation of our findings or trends to heater coolers used in clinical settings should be performed with caution.
Implications and Future Research
The intent of this research was not to perform exhaustive analysis and characterization of NTM aerosolization or characterization of the bubbler developed, rather to demonstrate the capabilities through proof of principle experiments in a controlled laboratory setting with minimal footprint requirements. Based on our results we suggest that use of surrogates, especially M. smegmatis in place of clinically relevant M. chimaera is not appropriate for aerosolization testing of HCDs. In addition, medical device manufacturers can use the bubbler design proposed here, as well as our sample methodology for validation studies before assessing the extent of aerosolization of M. chimaera from their HCDs. We also hope that academic investigators will engage in answering the existing scientific gaps (Weeks et al., 2020). In addition, determination of infection doses, and quantitative risk assessment models developed based on retrospective analysis of the infection patterns and bioburden (Haas et al., 2014; Chen et al., 2021) would help assess future risks of infection to patients.
Data Availability Statement
Additional information including videos, preliminary design and data analysis are included in the Supplementary Material. Further inquiries can be directed to the corresponding authors.
Author Contributions
All authors listed have made a substantial, direct, and intellectual contribution to the work and approved it for publication.
Funding
DW and NA were supported by an appointment to the Research Participation Program at the Center for Devices and Radiological Health administered by the Oak Ridge Institute for Science and Engineering through an interagency agreement between the U.S. Department of Energy and the U.S. Food and Drug Administration. This project was funded in part through the CDRH Critical Path Initiative.
Author Disclaimer
The mention of commercial products, their sources, or their use reported herein is not to be construed as either an actual or implied endorsement of such products by the Department of Health and Human Services. The findings and conclusions in this article have not been formally disseminated by the U.S. Food and Drug Administration and should not be construed to represent any Agency determination or policy.
Conflict of Interest
The authors declare that the research was conducted in the absence of any commercial or financial relationships that could be construed as a potential conflict of interest.
Publisher's Note
All claims expressed in this article are solely those of the authors and do not necessarily represent those of their affiliated organizations, or those of the publisher, the editors and the reviewers. Any product that may be evaluated in this article, or claim that may be made by its manufacturer, is not guaranteed or endorsed by the publisher.
Acknowledgments
We would like to thank CDRH's Medical Countermeasures staff particularly Dr. Suzanne Schwartz, Dr. Aftin Ross, and Ms. Julia Marders. We would like to thank CDC's epidemiology staff for helpful guidance and for M. chimaera strains, specifically Dr. Heather Moulton-Meissner and Commander Bryan Christensen. We thank CDRH's Mr. Randy Bidinger for creating the bubbler.
Supplementary Material
The Supplementary Material for this article can be found online at: https://www.frontiersin.org/articles/10.3389/frwa.2022.902872/full#supplementary-material
References
ATCC (2022). Mycobacterium smegmatis (Trevisan) Lehmann and Neumann. ATCC Available online at: https://www.atcc.org/products/19420 (accessed May 18, 2022).
Brown-Elliott, B. A., Nash, K. A., and Wallace, R. J. Jr. (2012). Antimicrobial suscepticbility testing, drug resistance mechanisms, and therapy of infections with nontuberculous mycobacteria. Clin. Microbiol. Rev. 25, 545–582. doi,: 10.1128/C. M. R.05030-11
Chand, M., Lamagni, T., Kranzer, K., Hedge, J., Moore, G., Parks, S., et al. (2017). Insidious risk of severe Mycobacterium chimaera infection in cardiac surgery patients. Clin. Infect. Dis. 64, 335–342. doi: 10.1093/cid/ciw754
Chen, Y.-H., Yan, C., Yang, Y.-F., and Ma, J.-X. (2021). Quantitative microbial risk assessment and sensitivity analysis for workers exposed to pathogenic bacterial bioaerols under various aeration modes in two wastewater treatment plans. Sci. Total Environ. 755, 142615. doi: 10.1016/j.scitotenv.2020.142615
Cheng, A. C., Stewardson, A. J., Mitchell, B. G., Collignon, P., Johnson, P. D., and Stuart, R. L. (2016). Mycobacterial infections due to contaminated heater cooler units used in cardiac bypass: an approach for infection control practitioners. Infect. Dis. Health 21, 154–161. doi: 10.1016/j.idh.2016.10.002
DSMZ (2022). German Collection of Microorganisms and Cell Cultures GmbH: Mycobacterium intraceullare subsp. chimaera. Available online at: https://www.dsmz.de/collection/catalogue/details/culture/DSM-44623 (accessed May 18, 2022).
European Centre for Disease Prevention Control (2015). Invasive cardiovascular infection by Mycobacterium chimaera potentially associated with heater-cooler units used during cardiac surgery. Available online at: https://www.ecdc.europa.eu/en/publications-data/invasive-cardiovascular-infection-mycobacterium-chimaera-potentially-associated (accessed August 20, 2021).
Falkinham, J. O. (2003). The changing pattern of nontuberculous mycobacterial disease. Can. J. Infect. Dis. 14, 281–286. doi: 10.1155/2003/323058
Falkinham, J. O. III. (2016). Current epidemiologic trends of the Nontuberculous Mycobacteria (NTM). Curr. Environ. Health Rep. 3, 161–167. doi: 10.1007/s40572-016-0086-z,
Ferguson, R. M. W., Garcia.-Alcega, S., Coulon, F., Dubrell, A. J., Whitby, C., and Colbeck, I. (2019). Bioaerosol biomonitoring: sampling optimization for molecular microbial ecology. Mol. Ecol. Resour. 19, 672–690. doi: 10.1111/1755-0998.13002
Garvey, M. I., Ashford, R., Bradley, C. W., Bradley, C. R., Martin, T. A., Walker, J., et al. (2016). Decontamination of heater-cooler units associated with contamination by atypical mycobacteria. J. Hosp. Infect. 93, 229–234. doi: 10.1016/j.jhin.2016.02.007
Haas, C. N., Rose, J. B., and Gerba, C. P. (2014). Quantitative Microbial Risk Assessment, 2nd Edn. Hoboken, NJ: Wiley. doi: 10.1002/9781118910030
Heidelberg, J. F., Shahamat, M., Levin, M., Rahman, I., Stelma, G., Grim, C., et al. (1997). Effect of aerosolization on culturability and viability of gram-negative bacteria. Appl. Environ. Micobiol. 63, 3585–3588. doi: 10.1128/aem.63.9.3585-3588.1997
Islam, M. S., Larimer, C., Ojha, A., and Nettleship, I. (2013). Antimycobacterial efficacy of silver nanoparticles as deposited on porous membrane filters. Materiaas Sci. Eng. C 33, 4575–4581. doi: 10.1016/j.msec.2013.07.013
Kanamori, H., Weber, D. J., and Rutala, W. A. (2017). Healthcare-associated Mycobacterium chimaera transmission and infection prevention challenges: role of heater-cooler units as a water source in cardiac surgery. Clin. Infect. Dis. 64, 343–346. doi: 10.1093/cid/ciw755
Kesavan, J., Schepers, D., and McFarland, A. R. (2010). Sampling and retention efficiencies of batch-type liquid-based bioaerosol samplers. Aeroosl Sci. Technol. 44, 817–829. doi: 10.1080/02786826.2010.497513
Kohler, P., Kuster, S. P., Bloemberg, G., Schulthess, B., Frank, M., Tanner, F. C., et al. (2015). Healthcare-associated prosthetic heart valve, aortic vascular graft, and disseminated Mycobacterium chimaera infections subsequent to open heart surgery Eur. Heart J. 36, 2745–2753. doi: 10.1093/eurheartj/ehv342
Le Dantec, C., Duguet, J. P., Montiel, A., Dumoutier, N., Dubrou, S., and Vincent, V. (2002). Chlorine disinfection of atypical mycobacteria isolated from a water distribution system. Appl. Environ. Micobiol. 68, 1025–1032. doi: 10.1128/AEM.68.3.1025-1032.2002
Maimelis, G., Berry, D., An, H. R., Yao, M., DeVoe, K., Fennell, D. E., et al. (2005). Design and performance of a single-pass bubbling bioaerosol generator. Atmos. Environ. 39, 3521–3533. doi: 10.1016/j.atmosenv.2005.02.043
Mohan, A., Padiapu, J., Baloni, P., and Chandra, N. (2015). Complete genome squences of a mycobactrium smegmatis laboratory strain (MC2 155) and isoniazid-resistant (4XR1/R2) mutant strains. Genome Announc. 3, e01520–e01514. doi: 10.1128/genomeA.01520-14
Phillips, M. S., and von Reyn, C. F. (2001). Nosocomial infections due to nontuberculous mycobacteria. Clin. Infect. Dis. 33, 1363–1374. doi: 10.1086/323126
Sax, H., Blomberg, G., Hasse, B., Sommerstein, R., Kohler, P., Achermann, Y., et al. (2015). Prolonged outbreak of Mycobacterium chimaera infection after open-chest heart surgery. Clin. Infect. Dis. 61, 67–75. doi: 10.1093/cid/civ198
SecureAire (2022). Particle Control Technology Combats Aerosolized Transmission of Mycobacterium chimaera from Heater-Cooler Units during Cardiac Surgery. Healthcare series: Hospital Acquired Infections – Mycobacterium chimaera. Available online at: https://secureaire.com/wpcontent/uploads/2021/07/Aerosolized-Transmission-of-Mycobacterium-and-Particle-Control-Technology-White-Paper.pdf (accessed May 26, 2022).
Simon, X., Duquenne, P., Koehler, V., Piernot, C., Coulais, C., and Faure, M. (2011). Aerosolisation of Escherichia coli and associated endotoxin using an improved bubbling bioaerosol generator. J. Aerosol Sci. 42, 517–531. doi: 10.1016/j.jaerosci.2011.05.002
Sommerstein, R., Ruegg, C., Kohler, P., Bloemberg, G., Kuster, S. P., and Sax, H. (2016). Transmission of Mycobacterium chimaera from heater-cooler units during cardiac surgery despite an ultraclean air ventilation system. Emerg. Infect. Dis. 22, 1008–1013. doi: 10.3201/eid2206.160045
Taylor, R. H., Falkinham, J. O., Norton, C. D., and LeChevallier, M. W. (2000). Chlorine, cholarmine, cholrine dioxide, and ozon susceptibility of Mycobacterium avium. Appl. Environ. Microbiol. 66, 1702–1705. doi: 10.1128/AEM.66.4.1702-1705.2000
Ulevicius, V., Willeke, K., Grinshpun, S. A., Donnelly, J., Lin, X., and Mainelis, G. (1997). Aerosolization of particles from a bubbling liquid: characteristics and generator development. Aerosol Sci. Technol. 26, 175–190. doi: 10.1080/02786829708965423
U. S. Food Drug Administration (2020). Nontuberculous Mycobacterium Infections Associated with Heater-Cooler Devices: FDA Safety Communication. Available online at: http://wayback.archive-it.org/7993/20170722215713/https:/www.fda.gov/MedicalDevices/Safety/AlertsandNotices/ucm466963.htm (accessed August 20, 2020).
U. S. Food Drug Administration (2021a). FDA's Ongoing Evaluations and Continued Monitoring of Reports of Nontuberculous Mycobacteria Infections Associated with Heater-Cooler Devices. Available online at: https://www.fda.gov/medical-devices/what-heater-cooler-device/fdas-ongoing-evaluation-and-continued-monitoring-reports-nontuberculous-mycobacteria-infections (accessed July 8, 2021).
U. S. Food Drug Administration (2021b). Recommendations for the Use of Any Heater Cooler Device. Available online at: https://www.fda.gov/medical-devices/what-heater-cooler-device/recommendations-use-any-heater-cooler-device (accessed July 8, 2021).
Vijay, S., Nair, R. R., Sharan, D., Jakkala, K., Mukkayan, M., Swaminath, S., et al. (2017). Mycobacterial cultures contain cell size and density specific sub-populations of cells with significant differential susceptibility to antibiotics, oxidative and nitrite stress. Front. Microbiol. 8, 463. doi: 10.3389/fmicb.2017.00463
Keywords: heater cooler devices, Mycobacterium chimaera, NTM, bioaerosols, Mycobacterium smegmatis, hospital associated infections
Citation: Guha S, Wolloscheck D, Abdali N, Wentz C, Gillette N, Bauer KA and Weeks JW (2022) A Methodology for Investigating Aerosolization of Nontuberculous Mycobacteria From Contaminated Heater Cooler Devices. Front. Water 4:902872. doi: 10.3389/frwa.2022.902872
Received: 23 March 2022; Accepted: 30 May 2022;
Published: 27 June 2022.
Edited by:
Nhamo Chaukura, Sol Plaatje University, South AfricaReviewed by:
Cheng Yan, China University of Geosciences Wuhan, ChinaTodd Primm, Sam Houston State University, United States
Copyright © 2022 Guha, Wolloscheck, Abdali, Wentz, Gillette, Bauer and Weeks. This is an open-access article distributed under the terms of the Creative Commons Attribution License (CC BY). The use, distribution or reproduction in other forums is permitted, provided the original author(s) and the copyright owner(s) are credited and that the original publication in this journal is cited, in accordance with accepted academic practice. No use, distribution or reproduction is permitted which does not comply with these terms.
*Correspondence: Suvajyoti Guha, suvajyoti.guha@fda.hhs.gov; Jon W. Weeks, jon.weeks@fda.hhs.gov