- 1Department of Biological, Geological and Environmental Sciences, Cleveland State University, Cleveland, OH, United States
- 2Department of Forestry and Natural Resources, University of Kentucky, Lexington, KY, United States
As watersheds are complex systems that are difficult to directly study, the streams that drain them are often sampled to search for watershed “signals.” These signals include the presence and/or abundance of isotopes, types of sediment, organisms (including pathogens), chemical compounds associated with ephemeral biogeochemical processes or anthropogenic impacts, and so on. Just like watersheds can send signals via the streams that drain from them, we present a conceptual analysis that suggests plant canopies (equally complex and hard-to-study systems) may send similar signals via the precipitation that drains down their stems (stemflow). For large, tall, hard-to-access tree canopies, this portion of precipitation may be modest, often <2%; however, stemflow waters, like stream waters, scour a large drainage network which may allow stemflow to pick up various signals from various processes within and surrounding canopies. This paper discusses some of the signals that the canopy environment may impart to stemflow and their relevance to our understanding of vegetated ecosystems. Being a conceptual analysis, some examples have been observed; most are hypothetical. These include signals from on-canopy biogeochemical processes, seasonal epi-faunal activities, pathogenic impacts, and the physiological activities of the canopy itself. Given stemflow's currently limited empirical hydrological, ecological and biogeochemical relevance to date (mostly due to its modest fraction in most forest water cycles), future work on the possible “signals in stemflow” may also motivate more natural scientists and, perhaps some applied researchers, to rigorously monitor this oft-ignored water flux.
Introduction: Water sends “mixed signals”
As water drains over and through landscapes into soils and eventually waterbodies, it interacts with the many components of that landscape. Rainwater, for example, may pass through the forest canopy—a layer containing tree leaves, epiphytes, detritus, canopy soils, and bark—before it continues its path to, over, and through the surface. Snow may take even longer to traverse this same hydrologic flow path from the canopy to the surface (Klamerus-Iwan et al., 2020). Watersheds that contain these flow pathways are complex systems, posing a challenge to scientists interested in understanding how forest ecosystems function and how those functions are impacted by natural and anthropogenic change. Many water states and fluxes within watersheds are challenging to observe, and sometimes currently impossible; thus, virtual experimentation is often required to test and improve theory regarding watershed hydrological processes (Weiler and McDonnell, 2004). However, when these various flow paths converge at a single accessible point, like at the mouth of a stream, this presents an opportunity for scientists to observe much of a watershed's complexity from a single vantage point. In this way, the convergence of flow paths through watersheds can result in streams literally delivering “mixed signals” (conveyed through the chemical, physical and biological properties of water samples) from throughout the watershed to scientists sampling stream discharge.
Signals in stream water have been noticed and interpreted by various interests since long before watershed hydrology was conceptualized. For over 6,000 years, gold flakes suspended within rivers have enabled the discovery of placer deposits that, in turn, supported art and generated wealth in cultures around the world (Boyle, 1979; Yeend and Shawe, 1989). In Tibet around 1330 CE, the Islamic scholar Ibn Battuta reported that during heavy rainfalls, the rivers carried chemical signals from the grasses of that watershed (Ibn-Battuta and Abdallāh, 1829). Leonardo da Vinci noted around 1490 that streams both carried and sorted geological signals (rocks, gravel, sediments, etc.) from their watersheds (in his notes on “The origin of sand in rivers”). Since these early observations, modern scientists have found various other natural and anthropogenic signals in stream waters. The water itself contains isotopic signals which may be used to estimate the time that water took to travel through the system (Sprenger et al., 2019). Insights about how watersheds are affected by agricultural practices may also be found; for example, one may assess the severity of pesticide applications or the extent of biosolid applications via pharmaceutical tracers in stream waters (Metcalfe et al., 2019). Even patterns in the illicit or recreational use of pharmaceuticals in a population, whose waste products enter a watershed, can be identified within the mixed chemical signals of stream waters (Rodayan et al., 2016; Wilkinson et al., 2022). Waste byproducts from various forms of life within a watershed may be identified through microbial source tracking (Jiang et al., 2007; Ballesté et al., 2020), yielding insights into landscape ecology. Waste signals in stream waters may also be useful in understanding the fate and transport of certain waterborne pathogens (Weidhaas et al., 2018). Finally, but certainly not exhaustively, by literally shedding light on stream water samples, the resulting optical properties we observe may metaphorically shed light on the sources and processes operating on organic materials in watersheds (Fellman et al., 2010; Hosen et al., 2021).
Of course, other waters that scour other complex systems before discharging at a single point, may also be capable of carrying mixed signals from those systems. Plant canopies are complex systems that, like watersheds, pose challenges to scientists interested in directly observing specific processes. Tall tree canopies are especially difficult to access, requiring specialized climbing gear and training to safely perform direct personal observations and sampling, or to install automated monitoring technologies (Anderson et al., 2020; Cannon et al., 2021). Scientists without climbing gear and training can use other specialized (and often expensive) gear and training to study canopy ecosystem processes, like remote sensing technology from the ground (i.e., terrestrial lidar) or from the sky via drones or satellites (Zhang et al., 2016; Hanan and Anchang, 2020). Regardless of the technique, the view from climbers and remote sensing both reveal that, like watersheds, the tree canopy environment is spatially and temporally heterogenous (e.g., Merrick et al., 2021), resulting in a diversity of canopy microclimates (Ehbrecht et al., 2019) and microhabitats (Larrieu et al., 2018). During precipitation and condensation events, the heterogeneity of forest canopy structure can form complex water runoff patterns that may drain across/through a diversity of canopy microhabitats (Van Stan et al., 2020b). Like a watershed's soils, branches and bark pore spaces can generate a complex network of heterogenous pores that may act as an accumulator, transporter, substrate, and reactor for draining waters and their suspended and dissolved materials (Ponette-González, 2021) (Figure 1). Some tree canopies, especially in old growth forests, may host an arboreal litter and soil habitat (Gotsch et al., 2016). Within these arboreal soils, on the leaf and bark surfaces, and throughout the various microhabitats, is a wide range of microbes (Koskella, 2020; Looby et al., 2020), flora (Van Stan and Pypker, 2015; Mendieta-Leiva et al., 2020) and fauna (Nadkarni, 1994) (Figure 1). These canopy communities exist at a complex interface that modulates interactions between the atmosphere and the internal physiology of the tree (Van Stan et al., 2020b). Given these properties and processes, the forest canopy is a highly complex and difficult-to-access system that poses substantial challenges to interested researchers.
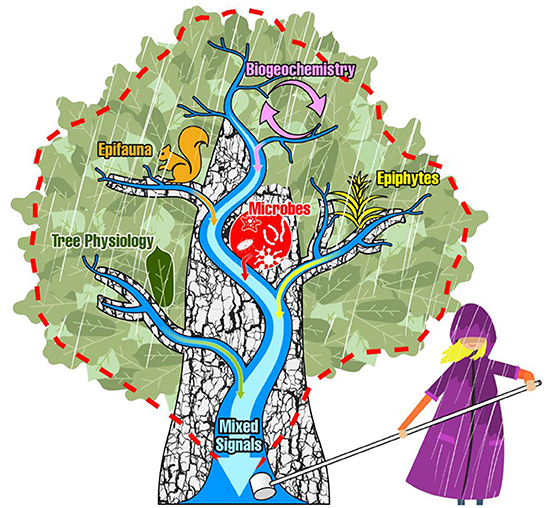
Figure 1. The classical depiction of a dendritic stream network and its watershed boundary (blue streams and red dashed line) is projected over the dendritic branchflow network of a tree canopy to illustrate the conceptual overlap. Here, stemflow at the base of the tree is analogous to the watershed discharge point. Signals are imparted to draining rainwaters from biogeochemical processes, epifauna, epiphytes, microbial communities, and the physiological processes of the tree itself—of which may all be sampled at a single discharge point, in stemflow.
A potential tool for canopy researchers to gain insights to the canopy environment may be stemflow—a water flux resulting from the drainage of precipitation or condensation down individual tree canopies that discharges at a single point at the base of the stem (Sadeghi et al., 2020). As stemflow drains down the tree, it scours canopy surfaces, picking up nutrients, pollutants, particles, and organisms along the way (Van Stan et al., 2021) (Figure 1). A larger portion of precipitation draining through canopies reaches the surface through gaps and by dripping from canopy elements; however, signals within this “throughfall” are not analogous to stream discharge as throughfall can drain and drip through multiple overlapping tree canopies (i.e., through multiple canopy systems). Since throughfall integrates signals from an unknown (or difficult to delineate) area and number of neighboring individual tree canopies, targeting individual canopies of interest through collecting throughfall samples would be very challenging. Spatial patterns of throughfall can also vary across storm conditions, hypothetically due, in part, to variability in canopy saturation and, thereby, variability in the drainage area producing throughfall (Van Stan et al., 2020a). We note that, despite the variable and difficult-to-delineate source area of throughfall, it may still carry signals from the forest canopy in general. In forests where stemflow is exceedingly low and occurs infrequently (several examples may be found in Van Stan and Gordon, 2018), throughfall may be a useful tool for monitoring general forest canopy signals.
Where stemflow is frequently sampleable, it may represent an important monitoring tool for discrete canopies. Sampleable stemflow has been reported from a wide diversity of ecosystems and climates (Sadeghi et al., 2020). The size of a storm generally needed to produce sampleable amounts of stemflow is not particularly large. Most trees in the published literature will produce stemflow after 1–4 mm of rain, i.e., after the bark has been saturated or during fog, condensation, and even rhyme melt (Ney, 1893; Ponette-Gonzalez et al., 2010). Because storms exceeding this magnitude occur frequently across forest types, stemflow is often produced in sampleable quantities in all wooded ecosystems. There have even been studies where large volumes of stemflow were sampled from arid shrublands during small storms (Martinez-Meza and Whitford, 1996).
Stemflow is overwhelmingly derived from precipitation (and condensation) waters draining down a single tree's canopy and, once a storm is large enough to generate stemflow, this flux is consistently delivered to the same location at the surface (i.e., down the stem). Thus, like stream discharge, stemflow is unique in that it may deliver mixed signals from a single complex system of up-gradient communities and processes directly to us at the surface, at a single accessible point. Collecting stemflow may therefore provide a relatively simple and affordable opportunity to make strong inferences into a myriad of ecological and biogeochemical processes that might otherwise be too expensive, too dangerous, or too difficult to be cataloged. To our knowledge, this common theoretical perspective in watershed research has not yet been applied to canopy research. In fact, despite stemflow having been a subject of discussion since the dawn of botanical sciences (in Theophrastus' Historia Plantarum: Van Stan and Friesen, 2020) and since the earliest known national forest hydrological monitoring systems were established (in the Kingdom of Saxony by Krutzsch, 1850; Friesen and Van Stan, 2019), it still appears to be largely overlooked in the natural sciences (Murray et al., 2013; Gutmann, 2020). To inspire discussion of this theoretical perspective, we provide a conceptual analysis of existing observations and hypotheses about stemflow as a carrier of mixed signals from the canopy.
Signals from canopy life
The canopy of a single tree can support a vibrant community consisting of lifeforms that span all biological kingdoms and most scales, from megafauna—i.e., animals weighing >5 kg (Berzaghi et al., 2018)—and flora (Mendieta-Leiva et al., 2020), to microbes and viruses (Koskella, 2020). The canopy environment can also include aquatic communities, like water-filled leaves and tree holes, called phytotelmata and dendrotelmata, respectively (Kitching, 1971, 2001). Many of the phytotelmata are hosted by the vascular plants residing on canopies (e.g., Zotz et al., 2020), but even some nonvascular vegetation on branches can hold substantial waters during and immediately after storms (Porada et al., 2018). For both types of epiphytic vegetation, the waters they hold can cycle relatively rapidly between storms (Hargis et al., 2019). The same appears true for dendrotelmata (Kitching, 1971; Schmidl et al., 2008). This results in dynamic, highly-localized aquatic habitats that contain their own unique (micro and macro) communities (Yanoviak, 2001; Magyar et al., 2017; Gossner and Petermann, 2022) compared to the communities living in leaf and bark habitats (Koskella, 2020; Magyar et al., 2021). Importantly, these small-scale canopy aquatic communities are ecologically intertwined with the broader terrestrial community. For example, dendrotelmata and phytotelmata can play important roles in the life cycles of terrestrial animals, like insects or amphibians, and in attracting larger fauna, like birds and mammals, to the canopy environment (Wittman, 2000; Delgado-Martínez et al., 2022). As a result, the forest canopy overall is estimated to support ~40% of extant species, of which 10% may be canopy specialists, inspiring natural scientists to describe the forest canopy as the interface where Earth's “biodiversity meets the atmosphere” (Stork et al., 1996; Ozanne et al., 2003). New methods to receive and decipher signals from canopy life—like those that may be found in stemflow—could therefore inform theory and management of broader Earth system processes (species richness patterns, biogenic gas exchange, ecosystem services, etc.). Conceptually, most (if not all) of the organisms living on and within leaves, bark, epiphytes, phytotelmata, and dendrotelmata leave some signal of their presence, activities, and interactions on canopy surfaces to be washed down by stemflow to scientists at the forest floor.
Canopy fauna
Canopy animals include all major biological classes (except for fish for obvious reasons). Signals may be imparted to stemflow from rainwater contacting an animal's external microenvironments (e.g., skin or fur: Kolodny et al., 2019; Rebollar et al., 2020) or from contacting materials released from their internal systems (e.g., from feces or other excretions: Demajo et al., 2011; Vidkjær et al., 2016). Some canopy animals do not exhibit shelter-seeking behaviors during storms and may directly interact with stemflow (Mella et al., 2020; de Albuquerque et al., 2021), providing an opportunity for stemflow to directly transfer signals from these animals to the surface. Excretions may represent the broadest possible signal for animal life (depending on the persistence of excretions during dry periods) as they will remain on canopy surfaces regardless of an animal's shelter-seeking behavior. At present, the only work known to the authors regarding the impact of larger canopy animals on draining precipitation waters has focused on waste products in throughfall (Beard et al., 2002). This case study in Puerto Rico found that the tree frog, Eleutherodactylus coqui, can contribute significant amounts of macronutrient ions to draining rainwaters through waste excretions (Beard et al., 2002). Hypothetically, if these waste-related macronutrient ions are present in stemflow, they may represent signals of the frog species' presence or abundance, and changes in its chemical composition may signal changes in the frogs' diet or digestive health.
A handful of studies on the effect of canopy insect infestations also report that excretions affected rainwater chemistry: significantly elevating nitrogen (N) and carbon (C) concentrations (Stadler and Michalzik, 1998; le Mellec and Michalzik, 2008; Grüning et al., 2017). In one case, the frass itself was present as a particulate organic matter signal in throughfall (le Mellec et al., 2010). Although stemflow was not measured in this study, given the size range of stemflow-suspended particles (Levia et al., 2013) it is plausible that stemflow could also carry signals of insect particulate waste to the surface. The environmental DNA (eDNA) left by canopy fauna on the surfaces of leaves and bark or within canopy aquatic microhabitats may also enter stemflow. Continuing with the tree frog example, the dendrotelmata or phytotelmata in which many tree frogs rely for food, shelter and/or breeding have recently been found to house detectable levels of their eDNA (Barata et al., 2021; Mullin et al., 2022). During large storms, these canopy aquatic microhabitats overflow and drain down tree stems (as discussed in greater detail in the following sections), potentially delivering the eDNA to the surface at the base of the tree stem. In this way, the eDNA in stemflow may signal the presence of rare or elusive species in individual tree canopies.
Smaller canopy fauna may become directly suspended within draining stemflow waters. The visible presence of insects has been considered “contamination” in the past (Ponette-González et al., 2020). Perhaps future work may consider the assemblage of visible arthropods in stemflow bins as a signal of the canopy arthropod community composition. It is plausible that storms can wash off canopy arthropods at various life stages, enabling ecologists to gain insights into the temporal and spatial (i.e., tree-to-tree) dynamics of arthropod life cycles by sampling stemflow. Microscopic canopy animals (microfauna) have generally not been considered contamination in stemflow and, recently, these organisms have received increased attention (Ptatscheck et al., 2018; Guidone et al., 2021). As some of these microfauna are used as biocontrol agents, especially nematodes (Grewal et al., 2005), their presence in stemflow could provide insights into the uniformity and efficacy of biocontrol applications (Ellsbury et al., 1996) or indicate the presence of natural biocontrol agents—as hypothesized in Magyar et al. (2021). Other microfauna can be disease agents in tree canopies where, again, nematodes figure prominently on this list (Carta et al., 2020; Qin et al., 2021). Thus, hypothetically, the presence or abundance of particular types or species of microfauna in stemflow waters may indicate an active (or the potential for) infection in the canopy above. In this case, collecting stemflow after storms may be a simpler, cheaper, passive diagnostic tool for determining which tree canopies are diseased compared to current climbing or drone-based methods. For healthy plant canopies, shifts in the composition of microfauna transported by stemflow could signal changes in the mechanisms that delivered microfauna to the canopy. For example, Guidone et al. (2021) reported substantial changes in the abundance and composition of rotifers, nematodes and flagellate protists when pollen events occurred, indicating that many of these organisms were transported by pollen (or by pollinators). These are just several hypothetical signals in stemflow that may shed insights into the presence, abundance, structure, function, origins, and dynamics of faunal communities in forest canopies.
Canopy flora
Forest canopies can host a diversity of vegetation types across a physiological range of nonvascular to vascular, and a range of adaptations, from living surficially on branches and leaves as obligate or opportunistic epiphytes, to relying on host tree internal resources, as parasites or hemi-parasites. In coastal redwood forests, where tree surfaces can collect airborne particles for >1,000 years, enough soil develops to support even plants that evolved a terrestrial growth form (Enloe et al., 2006). These plants (ferns, shrubs and even trees) can live in pockets of soil as epiphytes (i.e., facultative epiphytes). In places where epiphyte abundance is high, these communities provide additional leaf area that can be colonized by many species of fungi and other taxa (discussed in the next section), further promoting diversity in the system (Nieder et al., 2001). All canopy flora will produce reproductive materials, resulting in a highly diverse set of reproductive signals from pollen, seeds or fruits in vascular plants to soredia (fungal hyphae wrapped around cyanobacteria or algal cells) in lichens. If reproductive signals may be identified and monitored in stemflow, they will yield insights into relatively short, episodic life events for canopy flora. Spatiotemporal dynamics in stemflow-transported reproductive materials may also inform scientists about potential stressors or related ecological processes, as studies have found that diminished reproduction of soredia, for example, can signal excessive deposition of trace metals on lichens (Hauck and Paul, 2005) or provide a proxy for thallus growth of canopy lichens (Gauslaa, 2006).
Since epiphytic lichens and bryophytes lack, or only have primitive, controls over the exchange of water and solutes with their local external environment, they cannot help but also release chemical signals into draining waters like stemflow. Stemflow can become highly concentrated in dissolved organic matter (DOM) compared to other natural waters (Van Stan and Stubbins, 2018), and studies suggest that nonvascular epiphytes leach substantial DOM, possibly contributing signals of their presence and physiological state. Although rarely studied in waters draining from tree canopies, low molecular weight sugars and polyols can be readily available for leaching from nonvascular epiphytes by precipitation (Coxson et al., 1992). Their presence and variability in stemflow could signal a variety of processes and interactions as they are related to various ecophysiological functions, e.g., to cell wall structure and thermal tolerance during extreme desiccation (Crowe and Crowe, 1986). Indeed, tens-to-hundreds of kg ha−1 y−1 of sugars and polyols (and nutrient ions) have been recorded to leach into rainfall after long droughts (Coxson, 1991; Coxson et al., 1992). Many other compounds in stemflow may yield insights into the composition of the nonvascular epiphytic community, including the presence of a specific clade, e.g., some lignans, like megaceratonic and anthoceratonic acids, appear to be specific to hornworts (Asakawa et al., 2013; Commisso et al., 2021). It may be that the chemodiversity of DOM in stemflow can provide insights into the biodiversity of the canopy ecosystem—see discussion of similar hypotheses from first-order streams (Mosher et al., 2015).
Vascular epiphytes may have greater control over their exchange of water and solutes with the external local environment; however, many of these plants depend on physiological systems that are open to the environment. These include families like Bromeliaceae and Nepenthaceae that have developed tank-like leaf structures which can fill with precipitation or condensation, litter, organisms, and the plant's own excretions (Zotz, 2016). These phytotelmata have been reported to overflow under modest rainfall conditions (Benavides-Gordillo et al., 2019; Srivastava et al., 2020), e.g., during storms that exceed ~12 mm (Pereira et al., 2022), and may, therefore, contribute their waters (and associated signals) to stemflow frequently. Phytotelmata may appear modest in volume, ranging from 2 to 5,000 ml capacity tank−1 (Zotz and Thomas, 1999; Zotz et al., 2020); however, tropical tank bromeliads alone have been estimated to store 40,000–50,000 L ha−1 (Fish, 1983; Cogliatti-Carvalho et al., 2010) and to overflow >100 days each year (Pereira et al., 2022).
Phytotelmata overflow may contribute chemical and biological signals to stemflow indicating how these plants affect the canopy nutrient balance, microclimate and ecology. For example, it has recently been hypothesized from throughfall chemistry observations collected immediately beneath tank bromeliads that spill-over waters could be responsible for increased concentrations of not only plant-derived N and P, but Ca from small crustacean inhabitants (Pereira et al., 2022). These chemical signals appear to be large enough to influence soil chemistry patterns at the surface (Pereira et al., 2022). It is plausible that these signals will not only be in throughfall, but also in stemflow, because phytotelma overflows can contribute to the branchflows that become stemflow and many epiphytes reside on tree stems where they directly interact with stemflow (Chen et al., 2019). Phytotelmata support decomposition processes as well, which could supply nutrient (and organismal) signals to stemflow when they overflow (Kitching, 2001). Similarly, parasitic plants living on tree canopies, such as the globally-abundant mistletoe, have been recorded to concentrate nutrients from the canopy and enrich the soils below (Muvengwi et al., 2015). The extent to which this soil chemical influence is related to chemical enrichment of rainwaters by canopies (i.e., chemical signals in stemflow) has not yet been tested; hypothetically, stemflow may carry signals leached from parasitic plants which differ from the leaching of host tree leaves and bark. Thus, there is wide-ranging potential for stemflow signals to enable or complement investigations regarding the presence, community composition, ecophysiology, and nutrient dynamics of vegetation living on tree canopies.
Canopy microbial communities
The microbial communities that dwell on and in forests canopies, permanently or temporarily, include viruses, archaea, bacteria, fungi and single-celled eukaryotes (protists). Recent work has shown that many of these types of microbes (bacteria, fungal spores, and protists) can be washed from canopies by stemflow (Teachey et al., 2018; Guidone et al., 2021; Magyar et al., 2021). For host trees, their epiphytes and epifauna, microbiota can engage in various relationships of interest, including mutualism (Mejía et al., 2014), saprotrophism (Song et al., 2017), and pathogenism (Laine et al., 2014), though many, of course, have no known relationship. Parasitic and pathogenic signals in stemflow samples may be useful to assess the extent and severity of microbial disease agents, from viruses (D'Amico and Elkinton, 1995) and bacteria (Griffin and Carson, 2015), to protists, especially a group of particularly effective plant pathogens, oomycetes (Jauss et al., 2021). Viruses can travel in the waters draining through canopies, originating from literal reservoirs of water, like dendrotelmata (Kollars et al., 2019; Diouf et al., 2020), and from cadaverous reservoirs, like putrefying gypsy moths (D'Amico and Elkinton, 1995). In dendrotelmata, the host reservoirs for Zika (mosquitos) and the so-called “cryptic” reservoirs of Ebola (free-living pathogenic amoebae) may both be found (Kollars et al., 2019; Diouf et al., 2020). As these vectors and the tree-holes within which they reside are difficult to discover (being often situated above our heads and hidden within branch confluences), perhaps their signals may be observed in stemflow and thereby, their locations discovered and included in mosquito and amoeba management. Dendrotelmata have been recorded to store >15 L (Gossner et al., 2016); however, the contributions of branchflows and throughfall to these microhabitats during large storms may easily exceed this volume. Indeed, dendrotelmata can overflow and it may be worthwhile to assess whether these hosts (or the viruses themselves) may be washed into stemflow pathways.
On leaves, epiphytic bacterial communities are a reservoir of pathogens (and their antagonists) and it is known that the interception and drainage of rainwater can significantly impact these groups or momentarily enhance some microbes' mobility (Beattie, 2011). It is plausible, then, that stemflow bacterial signals collected throughout the year could yield insights into the dynamics of bacterial pathogens and their antagonists abundance and community composition in the phyllosphere reservoir. Geospatial patterns of fungal canopy pathogens are of increasing interest in regions where climate change is altering precipitation regimes (i.e., Chen et al., 2021), and stemflow monitoring may also be useful for such signals. Although no study applying high-throughput sequencing to examine fungal communities in stemflow has been published, per the authors' knowledge, fungal materials (i.e., asexual spores called “conidia”) have been studied in stemflow for over 50 years (Magyar et al., 2021). These studies report that fungal conidia can be abundant in stemflow: up to billions of conidia ha−1 y−1 (Magyar et al., 2021). It has been recently observed that tree canopies can hold more species of parasitic oomycetes with lifestyles that exclusively feed on living host tissue than in other forest compartments, implying tree canopies may themselves be a major reservoir for parasitic protists (Jauss et al., 2021). As stemflow washes much of the canopy, it may provide an integrated signal of this parasitic protist community and its compositional or functional dynamics.
Canopy microbes also play important roles in securing nutrients for host trees and epiphytes. For example, asymbiotic N fixation by phyllosphere microbial communities can supply as much (or more) N as the detritusphere or rhizosphere (Stewart, 1969; Son, 2001; Moreira et al., 2021) and it can vary substantially in response to disturbances, like fire (Bomfim et al., 2020) and drought (Moreira et al., 2021). A portion of the free-living microbes responsible for this N-fixation may be washed from the canopy by stemflow during storms. If so, stemflow collected from storms that occur before and after disturbances may signal shifts in this community's abundance or community structure. N-cycling functional genes for archaea and bacteria have been observed in throughfall (Watanabe et al., 2016; Guerrieri et al., 2020), suggesting they may also be measurable in stemflow.
Canopies have multiple opportunities for microbially-mediated N retention (Guerrieri et al., 2021), including in canopy soils—a compartment of this ecosystem recently gaining research attention (Gotsch et al., 2016; Tatsumi et al., 2021). Monitoring for known saprotrophic signals in stemflow, for instance, may provide insights into canopy soil processes and how they differ from processes in ground soils (Matson et al., 2014; Looby et al., 2020). Many decomposer communities in canopies can reside within the canopy microrefugia over which stemflow may drain. For example, microbes transported to the surface when litter- and water-filled treeholes overflow may yield insights into processes for these “aquatic islands in the sky” (Petermann and Gossner, 2022). Previous observations of dendrotelmata show significant temporal variability in the microbial communities for saprotrophic (as well as other types of) fungi (Magyar et al., 2017), protists (Yee et al., 2007; Walker et al., 2010), and algae (Ptatscheck and Traunspurger, 2015).
Even where no known relationship exists between a microbe and its canopy environment, its presence and abundance in stemflow may be a signal of interest. In the case of fungal spores, some species may be passing through the canopy ecosystem as part of their life cycle (Sridhar, 2009). Whether microbes are canopy residents with critical biogeochemical or ecological roles, pathogens or parasites waiting for an opportunity to pounce, or simply passers-by on their way between systems, stemflow may supply informative signals from these communities for a range of microbiological research topics.
Signals from biogeochemical processes
Forest canopies are regulatory interfaces between multiple Earth systems, influencing exchange between the soil, atmosphere, biosphere and hydrosphere (Bonan and Doney, 2018). Thus, biogeochemical processes within the forest canopy are relevant to the cycling of gasses (Baldocchi, 2020), particulate matter (Rindy et al., 2019), water (Coenders-Gerrits et al., 2020), and the balance of related sediments—both within and below canopies (Dunkerley, 2020). These biogeochemical processes-of-interest may impart signals to stemflow in dissolved (Van Stan and Gordon, 2018) and particulate form (Levia et al., 2013). Although canopy uptake and release of gasses may not directly impart signals to stemflow, deposition of inorganic compounds (Edgerton et al., 2020) and volatile organic C (especially oxygenated VOCs: Rantala et al., 2015) can alter the chemical composition of draining rainwaters. For example, deposition of VOCs may contribute to the enrichment of stemflow with dissolved organic C (Van Stan and Stubbins, 2018). Inorganic N and S deposition can enrich stemflow as well (Butler and Likens, 1995). Opportunities likely exist for stemflow signals to inform us regarding canopy water balances (isotopically: Allen et al., 2017; Pinos et al., 2022), the trapping and recycling of particulate aerosols (through its abiotic and biotic particulate matter content: Ponette-González, 2021), and the balance of canopy soils, where present (also through its particulate matter content).
The mechanisms driving interception and redistribution of precipitation by canopies is currently poorly understood compared to other components of the water cycle. This is indicated by longstanding disagreements between precipitation partitioning models and experimental data of energy, radiation, and mass balances in canopies (Shuttleworth, 1976; Martin et al., 2013; Lundquist et al., 2021). These models also employ a variety of equations and parameters for the same processes, some of which conflict (as in the case of snow interception) (Gutmann, 2020; Lundquist et al., 2021), and many exclude stemflow from the canopy water balance (Murray et al., 2013; Gutmann, 2020). As rainfall becomes stemflow, the stable isotope composition of this water changes due to several processes which alter the relative abundance of heavy and light isotopes. These fractionation processes leave a “mixed” isotopic signal in stemflow from evaporation, mixing with other canopy waters (like barkwater, dendrotelmata, or phytotelmata), and liquid-vapor exchange (Allen et al., 2017). Thus, stemflow (and throughfall) isotopic signals may aid in improving our understanding of the mechanisms driving evaporation and routing of precipitation in canopies. For example, recent results from within-storm monitoring of stemflow isotopic signals suggest that, since stemflow has longer residence times within the canopy than throughfall, it may yield strong inferences into the role of evaporation in fractionation (Pinos et al., 2022).
Leaf and bark surfaces of tree canopies present large (and aerodynamically rough) areas for atmospheric particulate deposition. As a result, canopies capture substantial amounts of particulate matter from the surrounding regions, and these particulate source regions literally change with the winds (and other factors) in natural (Cayuela et al., 2019) and urban settings (Ponette-González et al., 2022). Thus, the washing out of these trapped aerosols by stemflow in discrete storm events can signal which source area contributed most to canopy-atmosphere interactions before the storm (Teachey et al., 2018; Cayuela et al., 2019). A recent review of bark's role in trapping particulate aerosols suggest that the particulate matter reservoir of the bark (over which stemflow principally drains) can exceed that of leaves (Ponette-González, 2021). The particulate matter scavenged from the atmosphere and stored by bark is diverse, including crustal and anthropogenic particulates (Catinon et al., 2011), metals like iron, lead, copper, and cadmium (Su et al., 2013), fine particulates (<10 μm) from nearby industrial pollution and roadways (Suzuki, 2006), and large particulates up to 100 μm in natural settings (Xu et al., 2019). When stemflow washes over these bark surfaces the resulting particulate load variability across storms may, therefore, signal temporal and spatial variability in various anthropogenic and natural aerosol production/deposition activities.
In several forest ecosystems, tree canopies have been reported to develop soils on their branches, in branch confluences and beneath epiphyte mats, from montane cloud forests in Ecuador (Matson et al., 2014), Costa Rica (Gotsch et al., 2016) and Mexico (Victoriano-Romero et al., 2020) to temperate broadleaf and evergreen forests in Washington, USA (Haristoy et al., 2014). Canopy soil erosion and formation rates within canopies are poorly understood; however, scour by stemflow drainage is likely to erode and transport these soil particles to the surface. Given this, stemflow particulate transport may, hypothetically, inform efforts to understand canopy soil balances. Although canopy soil investigations are scarce, results to date indicate that this is a biogeochemically relevant component of the canopy ecosystem. For example, canopy soils in the Pacific Northwestern USA can represent 20 and 25% of C and N pools compared to the forest floor, respectively (Haristoy et al., 2014). In a forest located in the Ecuadorian Andes, canopy soils were also found to contribute up to 23% of total mineral N production and could be a significant N source for epiphytes (Matson et al., 2014). A survey of canopy soils throughout a mature cloud forest in Veracruz Mexico found that the local soil volume correlated strongly with Mg, Ca, P, and N (Victoriano-Romero et al., 2020). Thus, the stoichiometry of soil particles in stemflow may yield insights into the dynamics of various nutrient stocks in these soils. Since the available evidence currently suggests that various types of epiphytes may rely on canopy soil nutrient stocks (Matson et al., 2014; Gotsch et al., 2016; Victoriano-Romero et al., 2020), the monitoring of stemflow particulate chemistry may signal certain nutrient limitations for members of epiphyte communities. Especially scant observations exist on the particulate matter and isotopic signals in stemflow (Van Stan et al., 2021; Pinos et al., 2022); however, there appears to be significant potential for these kinds of data to yield insights into canopy biogeochemical processes.
Signals from tree physiology
In addition to the various signals that biotic and abiotic elements and processes in the canopy ecosystem can contribute to stemflow, there may be signals from the tree itself. Tree canopies respond to environmental changes, whether caused by natural cycles (like the changing of the seasons), or by natural and anthropogenic stressors (from relatively rapid attacks by pathogens and pests to the more chronic impacts of climate change). Many environmental changes, even those from human activity, are not always obvious to our senses and technologies; however, the plant may respond in ways that contribute detectable signals to the draining precipitation waters that become stemflow. For example, chemical composition and concentrations of stemflow from the same tree can vary substantially across discrete storms (Oka et al., 2021a,b), though the mechanisms that cause this variability are poorly understood (Levia and Germer, 2015). Should this high inter-event variability in stemflow chemistry be due to different signals from the tree itself, these signals may inform scientists about the organism's response to environmental changes. This, of course, may include tree response signals from events that have already occurred, but trees are also known to respond to precursors of major disturbance or mortality events. These precursor responses include changes in leaf chemistry with increasing drought stress (Taylor and Whitelaw, 2001), the release of chemicals in response to pest egg deposition (Hilker and Fatouros, 2015), or herbivory (Holopainen and Blande, 2013). Often, such changes as these are not plainly visible, not easily measurable, or may only manifest obvious/easily measurable signs after significant damages have occurred. Thus, some plant response signals carried by stemflow may not only be useful to observe these changes, but act as early warning indicators for some events. During some attacks, the pest or pathogen organism itself may not be available to stemflow (i.e., being within the plant or secured to the plant). In these cases, the biochemical responses of trees to these attacks can deposit onto canopy surfaces, becoming accessible to stemflow.
Plants can deploy a diversity of defensive biochemical tactics, many of which leave traces on canopy surfaces that can enter stemflow. In the case of insect herbivores, plant responses may begin soon after eggs are deposited on canopy surfaces (Hilker and Fatouros, 2015). Herbivorous insect eggs are generally secured to the canopy and, thus, unlikely to be transported by stemflow to the surface. But, the canopy itself can respond to these ovideposition events before any eggs hatch by altering leaf chemistry (Blenn et al., 2012), which can include the production of egg-killing (ovicidal) substances (Seino et al., 1996), and by releasing volatile organic compounds (VOCs) to attract egg parasitoids (e.g., Hilker et al., 2005). The studies on leaf chemistry and ovicidal substances focus on leaves from more accessible canopies (rice and Arabadopsis thaliana); however, these are the only studies known to the authors to have examined these processes. These responses may be possible in forest trees as well. The release of parasitoid attractant VOCs has been documented in forest trees and provides an example of how many factors may leave their trace in stemflow. A female phytophagous sawfly [Diprion pini L. (Hymenoptera, Diprionidae)], for example, makes a slit in a pine needle, deposits eggs using her ovipositor and coats them in a secretion. This secretion elicits a release of unique VOCs from the Scots pine (Pinus Sylvestris L.) where ovideposition occurs, which attracts the egg parasitoid Chrysonotomyia ruforum Krausse (Hymenoptera, Eulophidae) to parasitize the eggs and, thereby, prevent future damage to the tree (Hilker et al., 2005). Each of these factors may leave unique residues available for transport by stemflow and provide a signal to scientists at the surface of potential herbivory events. Likewise, there are a variety of secondary metabolites that plants may produce and accumulate for the sake of disease resistance that may be transported by stemflow (Zaynab et al., 2018).
Trees may respond to other environmental changes that appear as subtle to us as a sawfly's ovideposition on pine needles. Being immobile organisms, trees must face a host of climate change-induced disturbances. As ecosystems appear to be more sensitive to plant responses to climate change disturbances (compared to animal responses) (Schleuning et al., 2016), “distress” signals from the forest canopy in stemflow may provide timely insights for intervention that benefit the ecosystem overall. These effects are diverse, and their severity can vary. Many forests are or will be experiencing increased drought frequency and duration (Anderegg et al., 2020; Gazol and Camarero, 2022). Drought can alter canopy phenology in ways that provide stemflow opportunities to pick up signals. In particular, drought-related shifts in leaf chemistry and premature leaf senescence (Taylor and Whitelaw, 2001) may alter stemflow chemistry due to two hypothetical mechanisms. First, leaching of solutes by stemflow may change significantly in response to leaf chemical changes. Canopy leaching rates in a north-eastern US deciduous forest have been observed to increase by an order of magnitude during leaf senescence for multiple solutes, including Mg, K, Ca, and SO4 (Van Stan et al., 2012). Secondly, canopies with dense branching structures may capture senesced leaves (e.g., Nadkarni and Matelson, 1991) that can impart decomposition materials to draining stemflow.
Climate change is also altering precipitation characteristics. For example, many forests are being or will be impacted by hydrological intensification (Gloor et al., 2013; Creed et al., 2015). More intense precipitation rates could not only cause tree physiological responses, but increase the scouring area and efficacy of stemflow by increasing precipitation drainage rates within the canopy. This combination of processes could increase the likelihood or capability for stemflow to take-up signals and carry them in detectable concentrations to the surface. It has long been known that rain droplet impacts can erode the waxy cuticles of leaves and that climate variables are major factors influencing the relationship between cuticular wax development, structure, and its erosion by rainfall (Baker and Hunt, 1986). Stemflow may transport eroded wax structures. The microscopic and chemical analysis of stemflow-transported wax structures may represent signals of cuticular functions, which range from mechanical protection to water exchange (Kerstiens, 1996). Interestingly, in an Arabidopsis study wax composition was found to be a signal itself, where it may influence the number of trichomes and stomata formed in the epidermis (Bird and Gray, 2003). Thus, stemflow-transported wax structures may contain signals about leaf epidermal development. Finally, these climate changes can influence a tree's sensitivity to pests and pathogens. Therefore, signals from the canopy regarding climate change related stressors may also represent early warning signs of infestation or herbivory potential.
Sampling stemflow: A brief beginners guide
To the authors' knowledge, stemflow is not measured as often as other water fluxes in field research, nor is stemflow currently included in core monitoring protocols of national observatory networks. Thus, a brief primer on stemflow sampling methods may be helpful for anyone inspired to search for signals in stemflow. Currently, it is most common to install a thin, flexible gutter (or stemflow “collar”) around an individual plant stem to divert stemflow from the bark surface to a collection bin via gravity (Figure 2a). A thin-channeled collar on smooth bark trees can help reduce the influence of throughfall to negligible proportions. Where the bark is rough, however, a wider-channeled collar is recommended, as a thinner collar may miss the stemflow that drips from bark ridges. Rough bark may require some trimming (of the outer bark) so that the collar may be effectively positioned and sealed (Figure 2b). Take care not to pierce into the living tissue beneath. Collar materials generally consist of vinyl or silicon tubing cut in half, then attached to the stem with nails and sealed with silicon (Figure 2c). Since vinyl tubing tends to stiffen during cold conditions and can curl into itself when it freezes (thereby closing the collar channel) we recommend food-grade silicon tubing over vinyl because it will remain flexible during most cold (even freezing) conditions. To avoid penetrating through the bark with nails, one may wrap weather-proofing foam tape around the stem (which has an adhesive backing) to form the base of the collar channel (Figure 2d). Be sure to leave room for the drainage tube when wrapping the foam tape around the stem – see tubing in Figure 2d. This tape is a useful collar base as it is relatively water-repellant and can be purchased off-the-shelf at various widths to accommodate different bark types (Figure 2e). To form the wall of the channel, and prevent stemflow from running off the side, the outside of the tape can be wrapped with flexible plastic sheeting then sealed with silicon (Figure 2f). While the silicon sets and adheres the plastic to the foam tape, the collar may be held together with zip ties or hose clamps. Of course, each tree has its own eccentricities, resulting in each stemflow collar likely requiring some personal tweak to the design described.
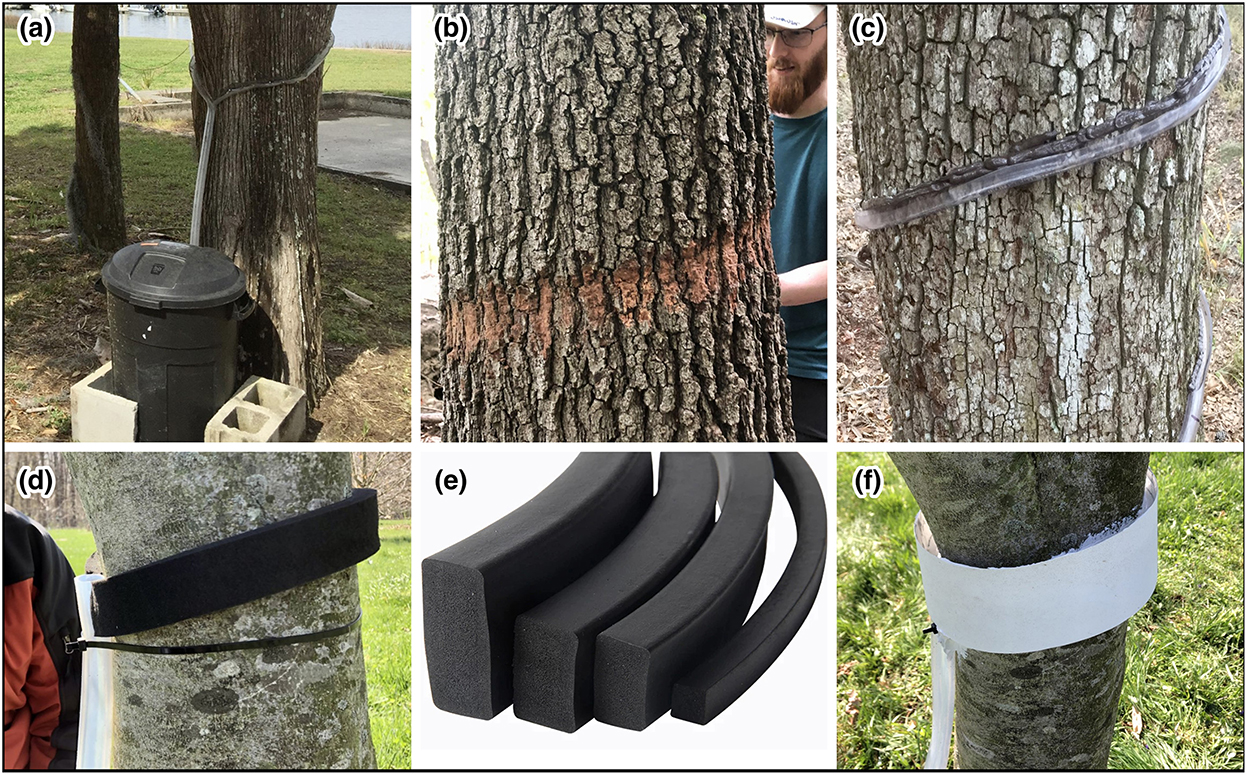
Figure 2. Stemflow may be captured for sampling in many ways. Generally, (a) stemflow is diverted from the bark surface by a collar that drains via gravity to a collection bin. (b) Where bark is thick, the outer bark may require trimming prior to installing a collar. (c) Stemflow collar materials often consist of flexible tubing, cut length-wise, wrapped around the stem, then nailed in place and sealed. (d) To avoid using nails, adhesive-backed foam tape may be used as a collar base, (e) which comes in a variety of widths to accommodate differing bark thicknesses, (f) and a wall of flexible plastic may be used to help direct stemflow to the drainage tube.
Conclusions
Tree canopies are complex systems that are currently difficult and expensive to study directly. However, like difficult-to-study watersheds, tree canopies drain precipitation and condensation to a single discharge point: to the base of their stems via stemflow. This stemflow scours the spatiotemporally heterogeneous canopy environment as it drains from branch tip-to-trunk, then down to the surface. As a result, stemflow may wash-off, leach, or fractionate materials, resulting a variety of signals (in the form of chemical compounds, sediments, organisms, and isotopes) from biogeochemical processes, epi-fauna, epiphytes, microbial communities, disturbances, and the physiological activities of the canopy itself. This conceptual analysis provided published examples and informed hypotheses that suggest, despite stemflow often representing a small fraction of the canopy water balance, the signals it carries may provide important information to progress our understanding of canopy ecosystem processes. If signals in stemflow are found to provide strong inferences into canopy biogeochemistry and ecology, it may also alleviate some socioeconomic barriers-of-entry to various fields of canopy ecosystem science for underserved and underrepresented communities. Since the cost of climbing and remote sensing equipment (including the financial and time costs of specialized training) can be high, monitoring signals in stemflow may prove a more affordable and accessible means of investigating canopy ecosystem processes compared to the standard methods in use today.
Author contributions
AM conceived the idea during conversations with JV while planning his thesis research. SG and DG expanded on this concept. AM and DG drafted the initial text under the guidance of JV. JV and SG revised the draft text. All authors contributed to the article and approved the submitted version.
Funding
We gratefully acknowledge the support of the US National Science Foundation (EAR-HS #1954538 and #2209775).
Acknowledgments
We thank the following colleagues for insightful conversations on various aspects of the manuscript (alphabetically listed): Drs. David Burke, Valentina Mella, Kevin Mueller, Diana Oviedo-Vargas, and Luke Pangle.
Conflict of interest
The authors declare that the research was conducted in the absence of any commercial or financial relationships that could be construed as a potential conflict of interest.
Publisher's note
All claims expressed in this article are solely those of the authors and do not necessarily represent those of their affiliated organizations, or those of the publisher, the editors and the reviewers. Any product that may be evaluated in this article, or claim that may be made by its manufacturer, is not guaranteed or endorsed by the publisher.
References
Allen, S. T., Keim, R. F., Barnard, H. R., McDonnell, J. J., and Brooks, J. R. (2017). The role of stable isotopes in understanding rainfall interception processes: a review. WREs Water 4, e1187. doi: 10.1002/wat2.1187
Anderegg, W. R. L., Trugman, A. T., Badgley, G., Konings, A. G., and Shaw, J. (2020). Divergent forest sensitivity to repeated extreme droughts. Nat. Clim. Chang. 10, 1091–1095. doi: 10.1038/s41558-020-00919-1
Anderson, D. L., Schulwitz, S., May, M., Hill, G., Koomjian, W., McClure, C. J. W., et al. (2020). Can increased training and awareness take forest research to new heights? Trees For. People 1, 100005. doi: 10.1016/j.tfp.2020.100005
Asakawa, Y., Ludwiczuk, A., and Nagashima, F. (2013). Phytochemical and biological studies of bryophytes. Phytochemistry 91, 52–80. doi: 10.1016/j.phytochem.2012.04.012
Baker, E. A., and Hunt, G. M. (1986). Erosion of waxes from leaf surfaces by simulated rain. New Phytol. 102, 161–173. doi: 10.1111/j.1469-8137.1986.tb00807.x
Baldocchi, D. D. (2020). How eddy covariance flux measurements have contributed to our understanding of Global Change Biology. Glob. Chang. Biol. 26, 242–260. doi: 10.1111/gcb.14807
Ballesté, E., Demeter, K., Masterson, B., Timoneda, N., Sala-Comorera, L., Meijer, W. G., et al. (2020). Implementation and integration of microbial source tracking in a river watershed monitoring plan. Sci. Total Environ. 736, 139573. doi: 10.1016/j.scitotenv.2020.139573
Barata, I., Griffiths, R. A., Fogell, D. J., and Buxton, A. S. (2021). Comparison of eDNA and visual surveys for rare and cryptic bromeliad-dwelling frogs. Herpetol. J. 31, 1–9. doi: 10.33256/hj31.1.19
Beard, K. H., Vogt, K. A., and Kulmatiski, A. (2002). Top-down effects of a terrestrial frog on forest nutrient dynamics. Oecologia 133, 583–593. doi: 10.1007/s00442-002-1071-9
Beattie, G. A. (2011). Water relations in the interaction of foliar bacterial pathogens with plants. Annu. Rev. Phytopathol. 49, 533–555. doi: 10.1146/annurev-phyto-073009-114436
Benavides-Gordillo, S., Farjalla, V. F., González, A. L., and Romero, G. Q. (2019). Changes in rainfall level and litter stoichiometry affect aquatic community and ecosystem processes in bromeliad phytotelmata. Freshw. Biol. 64, 1357–1368. doi: 10.1111/fwb.13310
Berzaghi, F., Verbeeck, H., Nielsen, M. R., Doughty, C. E., Bretagnolle, F., Marchetti, M., et al. (2018). Assessing the role of megafauna in tropical forest ecosystems and biogeochemical cycles - the potential of vegetation models. Ecography 41, 1934–1954. doi: 10.1111/ecog.03309
Bird, S. M., and Gray, J. E. (2003). Signals from the cuticle affect epidermal cell differentiation. New Phytol. 157, 9–23. doi: 10.1046/j.1469-8137.2003.00543.x
Blenn, B., Bandoly, M., Küffner, A., Otte, T., Geiselhardt, S., Fatouros, N. E., et al. (2012). Insect egg deposition induces indirect defense and epicuticular wax changes in Arabidopsis thaliana. J. Chem. Ecol. 38, 882–892. doi: 10.1007/s10886-012-0132-8
Bomfim, B., Silva, L. C. R., Marimon-Júnior, B. H., Marimon, B. S., Doane, T. A., and Horwath, W. R. (2020). Fire affects asymbiotic nitrogen fixation in southern Amazon forests. J. Geophys. Res. Biogeosci. 125. doi: 10.1029/2019JG005383
Bonan, G. B., and Doney, S. C. (2018). Climate, ecosystems, and planetary futures: the challenge to predict life in Earth system models. Science 359, eaam8328. doi: 10.1126/science.aam8328
Boyle, R. W. (1979). The Geochemistry of Gold and its Deposits. Ottawa, ON: Geological Survey of Canada.
Butler, T. J., and Likens, G. E. (1995). A direct comparison of throughfall plus stemflow to estimates of dry and total deposition for sulfur and nitrogen. Atmos. Environ. 29, 1253–1265. doi: 10.1016/1352-2310(94)00339-M
Cannon, C. H., Borchetta, C., Anderson, D. L., Arellano, G., Barker, M., Charron, G., et al. (2021). Extending our scientific reach in arboreal ecosystems for research and management. Front. For. Glob. Change 4, 712165. doi: 10.3389/ffgc.2021.712165
Carta, L. K., Handoo, Z. A., Li, S., Kantor, M., Bauchan, G., McCann, D., et al. (2020). Beech leaf disease symptoms caused by newly recognized nematode subspecies Litylenchus crenatae mccannii (Anguinata) described from Fagus grandifolia in North America. For. Pathol. 50, e12580. doi: 10.1111/efp.12580
Catinon, M., Ayrault, S., Spadini, L., Boudouma, O., Asta, J., Tissut, M., et al. (2011). Tree bark suber-included particles: a long-term accumulation site for elements of atmospheric origin. Atmos. Environ. 45, 1102–1109. doi: 10.1016/j.atmosenv.2010.11.038
Cayuela, C., Levia, D. F., Latron, J., and Llorens, P. (2019). Particulate matter fluxes in a Mediterranean mountain forest: interspecific differences between throughfall and stemflow in oak and pine stands. J. Geophys. Res. Atmos. 124, 5106–5116. doi: 10.1029/2019JD030276
Chen, L., Wang, L., Martin, C. E., and Lin, T. (2019). Mediation of stemflow water and nutrient availabilities by epiphytes growing above other epiphytes in a subtropical forest. Ecohydrology 12. doi: 10.1002/eco.2140
Chen, Q., Hu, H., Yan, Z., Li, C., Nguyen, B. T., Zhu, Y., et al. (2021). Precipitation increases the abundance of fungal plant pathogens in Eucalyptus phyllosphere. Environ. Microbiol. 23, 7688–7700. doi: 10.1111/1462-2920.15728
Coenders-Gerrits, A. M. J., Schilperoort, B., and Jiménez-Rodríguez, C. (2020). “Evaporative processes on vegetation: an inside look,” in Precipitation Partitioning by Vegetation: A Global Synthesis, eds J. T. Van Stan, E. Gutmann, and J. Friesen (Cham: Springer International Publishing), 35–48. doi: 10.1007/978-3-030-29702-2_3
Cogliatti-Carvalho, L., Rocha-Pessôa, T. C., Nunes-Freitas, A. F., and Rocha, C. F. D. (2010). Volume de água armazenado no tanque de bromélias, em restingas da costa brasileira. Acta Bot. Bras. 24, 84–95. doi: 10.1590/S0102-33062010000100009
Commisso, M., Guarino, F., Marchi, L., Muto, A., Piro, A., Degola, F., et al. (2021). Bryo-activities: a review on how bryophytes are contributing to the arsenal of natural bioactive compounds against fungi. Plants 10, 203. doi: 10.3390/plants10020203
Coxson, D. S. (1991). Nutrient release from epiphytic bryophytes in tropical montane rain forest (Guadeloupe). Can. J. Botany 69, 2122–2129. doi: 10.1139/b91-266
Coxson, D. S., McIntyre, D. D., and Vogel, H. J. (1992). Pulse release of sugars and polyols from canopy bryophytes in tropical montane rain forest (Guadeloupe, French West Indies). Biotropica 121–133. doi: 10.2307/2388665
Creed, I. F., Hwang, T., Lutz, B., and Way, D. (2015). Climate warming causes intensification of the hydrological cycle, resulting in changes to the vernal and autumnal windows in a northern temperate forest. Hydrol. Process. 29, 3519–3534. doi: 10.1002/hyp.10450
Crowe, J. H., and Crowe, L. M. (1986). “Stabilization of membranes in anhydrobiotic organisms,” in Membranes, Metabolism and Dry organisms, ed. A. C. Leopold (Ithaca, NY: Comstock Pub. Associate), 188–209.
D'Amico, V., and Elkinton, J. S. (1995). Rainfall effects on transmission of gypsy moth (Lepidoptera: Lymantriidae) nuclear polyhedrosis virus. Environ. Entomol. 24, 1144–1149. doi: 10.1093/ee/24.5.1144
de Albuquerque, N. M., Ruiz-Esparza, J., da Rocha, P. A., Beltrão-Mendes, R., and Ferrari, S. F. (2021). Spontaneous ingestion of water by a free-ranging maned sloth, Bradypus torquatus, in the Ibura National Forest, northeastern Brazil. Behaviour 158, 177–193. doi: 10.1163/1568539X-bja10059
Delgado-Martínez, C. M., Cudney-Valenzuela, S. J., and Mendoza, E. (2022). Camera trapping reveals multispecies use of water-filled tree holes by birds and mammals in a neotropical forest. Biotropica 54, 262–267. doi: 10.1111/btp.13030
Demajo, M. A., Cvetićanin, J., Stoiljkovi,ć, M., Trpkov, D., Andri,ć, V., Onjia, A., et al. (2011). Detection of elements and radioactivity in pellets from long-eared owls (Asio otus) inhabiting the city of Belgrade (Serbia). Chem. Ecol. 27, 393–400. doi: 10.1080/02757540.2011.607440
Diouf, B., Gaye, A., Diagne, C. T., Diallo, M., and Diallo, D. (2020). Zika virus in southeastern Senegal: survival of the vectors and the virus during the dry season. BMC Infect. Dis. 20, 371. doi: 10.1186/s12879-020-05093-5
Dunkerley, D. (2020). “A review of the effects of throughfall and stemflow on soil properties and soil erosion,” in Precipitation Partitioning by Vegetation: A Global Synthesis, eds J. T. Van Stan II, E. D. Gutmann, and J. Friesen (Cham: Springer International Publishing), 183−214. doi: 10.1007/978-3-030-29702-2_12
Edgerton, E. S., Hsu, Y.-M., White, E. M., Fenn, M. E., and Landis, M. S. (2020). Ambient concentrations and total deposition of inorganic sulfur, inorganic nitrogen and base cations in the Athabasca Oil Sands Region. Sci. Total Environ. 706, 134864. doi: 10.1016/j.scitotenv.2019.134864
Ehbrecht, M., Schall, P., Ammer, C., Fischer, M., and Seidel, D. (2019). Effects of structural heterogeneity on the diurnal temperature range in temperate forest ecosystems. For. Ecol. Manage. 432, 860–867. doi: 10.1016/j.foreco.2018.10.008
Ellsbury, M. M., Jackson, J. J., Woodson, W. D., Beck, D. L., and Stange, K. A. (1996). Efficacy, application distribution, and concentration by stemflow of Steinernema carpocapsae (Rhabditida: Steinernematidae) suspensions applied with a lateral-move irrigation system for corn root worm (Coleoptera: Chrysomelidae) control in maize. J. Econ. Entomol. 89, 74–81. doi: 10.1093/jee/89.1.74
Enloe, H. A., Graham, R. C., and Sillett, S. C. (2006). Arboreal histosols in old-growth redwood forest Canopies, Northern California. Soil Sci. Soc. Am. J. 70, 408–418. doi: 10.2136/sssaj2004.0229
Fellman, J. B., Hood, E., and Spencer, R. G. M. (2010). Fluorescence spectroscopy opens new windows into dissolved organic matter dynamics in freshwater ecosystems: a review. Limnol. Oceanogr. 55, 2452–2462. doi: 10.4319/lo.2010.55.6.2452
Fish, D. (1983). “Phytotelmata: flora and fauna.,” in Phytotelmata: Terrestrial Plants as Hosts for Aquatic Insect Communities, eds J. Frank, and L. Lounibos (Medford: Plexus Publishing), 1–27.
Friesen, J., and Van Stan, J. T. (2019). Early european observations of precipitation partitioning by vegetation: a synthesis and evaluation of 19th century findings. Geosciences 9, 423. doi: 10.3390/geosciences9100423
Gauslaa, Y. (2006). Trade-off between reproduction and growth in the foliose old forest lichen Lobaria pulmonaria. Basic Appl. Ecol. 7, 455–460. doi: 10.1016/j.baae.2005.12.007
Gazol, A., and Camarero, J. J. (2022). Compound climate events increase tree drought mortality across European forests. Sci. Total Environ. 816, 151604. doi: 10.1016/j.scitotenv.2021.151604
Gloor, M., Brienen, R. J. W., Galbraith, D., Feldpausch, T. R., Schöngart, J., Guyot, J.-L., et al. (2013). Intensification of the Amazon hydrological cycle over the last two decades. Geophys. Res. Lett. 40, 1729–1733. doi: 10.1002/grl.50377
Gossner, M. M., Lade, P., Rohland, A., Sichardt, N., Kahl, T., Bauhus, J., et al. (2016). Effects of management on aquatic tree-hole communities in temperate forests are mediated by detritus amount and water chemistry. J. Anim. Ecol. 85, 213–226. doi: 10.1111/1365-2656.12437
Gossner, M. M., and Petermann, J. S. (2022). Vertical stratification of insect species developing in water-filled tree holes. Front. For. Glob. Change 4, 816570. doi: 10.3389/ffgc.2021.816570
Gotsch, S. G., Nadkarni, N., and Amici, A. (2016). The functional roles of epiphytes and arboreal soils in tropical montane cloud forests. J. Trop. Ecol. 32, 455–468. doi: 10.1017/S026646741600033X
Grewal, P. S., Ehlers, R. U., and Shapiro-Ilan, D. I. (2005). Nematodes as Biocontrol Agents. Oxfordshire, UK: CABI Publishing. doi: 10.1079/9780851990170.0000
Griffin, E. A., and Carson, W. P. (2015). The ecology and natural history of foliar bacteria with a focus on tropical forests and agroecosystems. Bot. Rev. 81, 105–149. doi: 10.1007/s12229-015-9151-9
Grüning, M. M., Simon, J., Rennenberg, H., and l-M-Arnold, A. (2017). Defoliating insect mass outbreak affects soil N fluxes and tree N nutrition in scots pine forests. Front. Plant Sci. 8, 954. doi: 10.3389/fpls.2017.00954
Guerrieri, R., Lecha, L., Mattana, S., Cáliz, J., Casamayor, E. O., Barceló, A., et al. (2020). Partitioning between atmospheric deposition and canopy microbial nitrification into throughfall nitrate fluxes in a Mediterranean forest. J. Ecol. 108, 626–640. doi: 10.1111/1365-2745.13288
Guerrieri, R., Templer, P., and Magnani, F. (2021). Canopy exchange and modification of nitrogen fluxes in forest ecosystems. Curr. For. Rep. 7, 115–137. doi: 10.1007/s40725-021-00141-y
Guidone, M., Gordon, D. A., and Van Stan, J. T. (2021). Living particulate fluxes in throughfall and stemflow during a pollen event. Biogeochemistry 153, 323–330. doi: 10.1007/s10533-021-00787-7
Gutmann, E. D. (2020). “Global modeling of precipitation partitioning by vegetation and their applications,” in Precipitation Partitioning by Vegetation, eds J. T. Van Stan II, E. D. Gutmann, and J. Friesen (Cham: Springer International Publishing), 105–120. doi: 10.1007/978-3-030-29702-2_7
Hanan, N. P., and Anchang, J. Y. (2020). Satellites could soon map every tree on Earth. Nature 587, 42–43. doi: 10.1038/d41586-020-02830-3
Hargis, H., Gotsch, S. G., Porada, P., Moore, G. W., Ferguson, B., Van Stan, J. T., et al. (2019). Arboreal epiphytes in the soil-atmosphere interface: how often are the biggest “Buckets” in the canopy empty? Geosciences 9, 342. doi: 10.3390/geosciences9080342
Haristoy, C. T., Zabowski, D., and Nadkarni, N. (2014). Canopy soils of sitka spruce and bigleaf maple in the Queets River Watershed, Washington. Soil Sci. Soc. Am. J. 78, S118–24. doi: 10.2136/sssaj2013.07.0300nafsc
Hauck, M., and Paul, A. (2005). Manganese as a site factor for epiphytic lichens. Lichenologist 37, 409–423. doi: 10.1017/S0024282905014933
Hilker, M., and Fatouros, N. E. (2015). Plant responses to insect egg deposition. Annu. Rev. Entomol. 60, 493–515. doi: 10.1146/annurev-ento-010814-020620
Hilker, M., Stein, C., Schröder, R., Varama, M., and Mumm, R. (2005). Insect egg deposition induces defence responses in Pinus sylvestris : characterisation of the elicitor. J. Exp. Biol. 208, 1849–1854. doi: 10.1242/jeb.01578
Holopainen, J. K., and Blande, J. D. (2013). Where do herbivore-induced plant volatiles go? Front. Plant Sci. 4, 185. doi: 10.3389/fpls.2013.00185
Hosen, J. D., Allen, G. H., Amatulli, G., Breitmeyer, S., Cohen, M. J., Crump, B. C., et al. (2021). River network travel time is correlated with dissolved organic matter composition in rivers of the contiguous United States. Hydrol. Process. 35, e14124. doi: 10.1002/hyp.14124
Ibn-Battuta, M. I., and Abdallāh, Batuta, I. (1829). The Travels of Ibn Batuta. Oriental translation fund of Gr. Britain and Ireland. Mineola, NY: DOVER PUBLICATIONS, INC.
Jauss, R.-T., Walden, S., Fiore-Donno, A. M., Schaffer, S., Wolf, R., Feng, K., et al. (2021). A parasite's paradise: biotrophic species prevail oomycete community composition in tree canopies. Front. For. Glob. Change 4, 668895. doi: 10.3389/ffgc.2021.668895
Jiang, S. C., Chu, W., Olson, B. H., He, J.-W., Choi, S., Zhang, J., et al. (2007). Microbial source tracking in a small southern California urban watershed indicates wild animals and growth as the source of fecal bacteria. Appl. Microbiol. Biotechnol. 76, 927–934. doi: 10.1007/s00253-007-1047-0
Kerstiens, G. (1996). Signalling across the divide: a wider perspective of cuticular structure—function relationships. Trends Plant Sci. 1, 125–129. doi: 10.1016/S1360-1385(96)90007-2
Kitching, R. L. (1971). An ecological study of water-filled tree-holes and their position in the woodland ecosystem. J. Anim. Ecol. 281–302. doi: 10.2307/3247
Kitching, R. L. (2001). Food webs in phytotelmata: “bottom-up” and “top-down” explanations for community structure. Annu. Rev. Entomol. 46, 729–760. doi: 10.1146/annurev.ento.46.1.729
Klamerus-Iwan, A., Link, T. E., Keim, R. F., and Van Stan II, J. T. (2020). “Storage and routing of precipitation through canopies,” in Precipitation Partitioning by Vegetation, eds J. T. van Stan II, E. D. Gutmann, and J. Friesen (Cham: Springer), 17–34.
Kollars T. M. Jr., Senessie, C., and Sunderland, G. (2019). Indentifying and modeling the distribution of cryptic reservoirs of Ebola virus using artificial intelligence. Afr. J. Clin. Exp. Microbiol. 19, 229–237. doi: 10.4314/ajcem.v19i3.11
Kolodny, O., Weinberg, M., Reshef, L., Harten, L., Hefetz, A., Gophna, U., et al. (2019). Coordinated change at the colony level in fruit bat fur microbiomes through time. Nat Ecol Evol 3, 116–124. doi: 10.1038/s41559-018-0731-z
Krutzsch, H. (1850). Untersuchungen Über die Waldstreu. Forstwirtschaftliches Jahrbuch. Königl.-Sächs. Akademie für Forst- und Landwirthe zu Tharandt. 6, 88–110.
Laine, A.-L., Burdon, J. J., Nemri, A., and Thrall, P. H. (2014). Host ecotype generates evolutionary and epidemiological divergence across a pathogen metapopulation. Proc. R. Soc. B Biol. Sci. 281, 20140522. doi: 10.1098/rspb.2014.0522
Larrieu, L., Paillet, Y., Winter, S., Bütler, R., Kraus, D., Krumm, F., et al. (2018). Tree related microhabitats in temperate and Mediterranean European forests: a hierarchical typology for inventory standardization. Ecol. Indic. 84, 194–207. doi: 10.1016/j.ecolind.2017.08.051
le Mellec, A., Meesenburg, H., and Michalzik, B. (2010). The importance of canopy-derived dissolved and particulate organic matter (DOM and POM)–comparing throughfall solutionfrom broadleaved and coniferous forests. Ann. Sci. For. 67, 411. doi: 10.1051/forest/2009130
le Mellec, A., and Michalzik, B. (2008). Impact of a pine lappet (Dendrolimus pini) mass outbreak on C and N fluxes to the forest floor and soil microbial properties in a Scots pine forest in Germany. Can. J. For. Res. 38, 1829–1841. doi: 10.1139/X08-045
Levia, D. F., and Germer, S. (2015). A review of stemflow generation dynamics and stemflow-environment interactions in forests and shrublands. Rev. Geophys. 53, 673–714. doi: 10.1002/2015RG000479
Levia, D. F., Michalzik, B., Bischoff, S., Näthe, K., Legates, D. R., Gruselle, M.-C., et al. (2013). Measurement and modeling of diameter distributions of particulate matter in terrestrial solutions. Geophys. Res. Lett. 40, 1317–1321. doi: 10.1002/grl.50305
Looby, C. I., Hollenbeck, E. C., and Treseder, K. K. (2020). Fungi in the Canopy: how soil fungi and extracellular enzymes differ between canopy and ground soils. Ecosystems 23, 768–782. doi: 10.1007/s10021-019-00439-w
Lundquist, J. D., Dickerson-Lange, S., Gutmann, E., Jonas, T., Lumbrazo, C., and Reynolds, D. (2021). Snow interception modelling: isolated observations have led to many land surface models lacking appropriate temperature sensitivities. Hydrol. Process. 35. doi: 10.1002/hyp.14274
Magyar, D., Van Stan, J. T., and Sridhar, K. R. (2021). Hypothesis and theory: fungal spores in stemflow and potential bark sources. Front. For. Glob. Change 4, 19. doi: 10.3389/ffgc.2021.623758
Magyar, D., Vass, M., and Oros, G. (2017). Dendrotelmata (water-filled tree holes) as fungal hotspots-a long term study. Cryptogam Mycol. 38, 55–66. doi: 10.7872/crym/v38.iss1.2017.55
Martin, K. A., Van Stan, J. T., Dickerson-Lange, S. E., Lutz, J. A., Berman, J. W., Gersonde, R., et al. (2013). Development and testing of a snow interceptometer to quantify canopy water storage and interception processes in the rain/snow transition zone of the North Cascades, Washington, USA. Water Resour. Res. 49, 3243–3256. doi: 10.1002/wrcr.20271
Martinez-Meza, E., and Whitford, W. G. (1996). Stemflow, throughfall and channelization of stemflow by roots in three Chihuahuan desert shrubs. J. Arid Environ. 32, 271–288.
Matson, A. L., Corre, M. D., and Veldkamp, E. (2014). Nitrogen cycling in canopy soils of tropical montane forests responds rapidly to indirect N and P fertilization. Glob. Chang. Biol. 20, 3802–3813. doi: 10.1111/gcb.12668
Mejía, L. C., Herre, E. A., Sparks, J. P., Winter, K., García, M. N., van Bael, S. A., et al. (2014). Pervasive effects of a dominant foliar endophytic fungus on host genetic and phenotypic expression in a tropical tree. Front. Microbiol. 5, 479. doi: 10.3389/fmicb.2014.00479
Mella, V. S. A., Orr, C., Hall, L., Velasco, S., and Madani, G. (2020). An insight into natural koala drinking behaviour. Ethology 126, 858–863. doi: 10.1111/eth.13032
Mendieta-Leiva, G., Porada, P., and Bader, M. Y. (2020). “Interactions of epiphytes with precipitation partitioning,” in Precipitation Partitioning of Vegetation: A Global Synthesis, eds J. T. Van Stan II, E. D. Gutmann, and J. Friesen (Cham: Springer International Publishing), 133–146. doi: 10.1007/978-3-030-29702-2_9
Merrick, T., Pau, S., Detto, M., Broadbent, E. N., Bohlman, S. A., Still, C. J., et al. (2021). Unveiling spatial and temporal heterogeneity of a tropical forest canopy using high-resolution NIRv, FCVI, and NIRvrad from UAS observations. Biogeosciences 18, 6077–6091. doi: 10.5194/bg-18-6077-2021
Metcalfe, C. D., Helm, P., Paterson, G., Kaltenecker, G., Murray, C., Nowierski, M., et al. (2019). Pesticides related to land use in watersheds of the Great Lakes basin. Sci. Total Environ. 648, 681–692. doi: 10.1016/j.scitotenv.2018.08.169
Moreira, J. C. F., Brum, M., de Almeida, L. C., Barrera-Berdugo, S., de Souza, A., de Camargo, A., et al. (2021). Asymbiotic nitrogen fixation in the phyllosphere of the Amazon forest: changing nitrogen cycle paradigms. Sci. Total Environ. 773, 145066. doi: 10.1016/j.scitotenv.2021.145066
Mosher, J. J., Kaplan, L. A., Podgorski, D. C., McKenna, A. M., and Marshall, A. G. (2015). Longitudinal shifts in dissolved organic matter chemogeography and chemodiversity within headwater streams: a river continuum reprise. Biogeochemistry 124, 371–385. doi: 10.1007/s10533-015-0103-6
Mullin, K. E., Barata, I. M., Dawson, J., and Orozco-terWengel, P. (2022). First extraction of eDNA from tree hole water to detect tree frogs: a simple field method piloted in Madagascar. Conserv. Genet. Resour. 14, 99–107. doi: 10.1007/s12686-021-01245-0
Murray, S. J., Watson, I. M., and Prentice, I. C. (2013). The use of dynamic global vegetation models for simulating hydrology and the potential integration of satellite observations. Prog. Phys. Geogr. 37, 63–97. doi: 10.1177/0309133312460072
Muvengwi, J., Ndagurwa, H. G. T., and Nyenda, T. (2015). Enhanced soil nutrient concentrations beneath-canopy of savanna trees infected by mistletoes in a southern African savanna. J. Arid Environ. 116, 25–28. doi: 10.1016/j.jaridenv.2015.01.017
Nadkarni, N. M. (1994). Diversity of species and interactions in the upper tree canopy of forest ecosystems. Am. Zool. 34, 70–78. doi: 10.1093/icb/34.1.70
Nadkarni, N. M., and Matelson, T. J. (1991). Fine litter dynamics within the tree canopy of a tropical cloud forest. Ecology 72, 2071–2082. doi: 10.2307/1941560
Nieder, J., Prosperí, J., and Michaloud, G. (2001). Epiphytes and their contribution to canopy diversity. Plant Ecol. 153, 51–63. doi: 10.1023/A:1017517119305
Oka, A., Takahashi, J., Endoh, Y., and Seino, T. (2021a). Bark effects on stemflow chemistry in a japanese temperate forest I. The role of bark surface morphology. Front. For. Glob. Change 4, 654375. doi: 10.3389/ffgc.2021.654375
Oka, A., Takahashi, J., Endoh, Y., and Seino, T. (2021b). Bark effects on stemflow chemistry in a japanese temperate forest II. The role of bark anatomical features. Front. For. Glob. Change 4, 657850. doi: 10.3389/ffgc.2021.657850
Ozanne, C. M. P., Anhuf, D., Boulter, S. L., Keller, M., Kitching, R. L., K?rner, C., et al. (2003). Biodiversity meets the atmosphere: a global view of forest canopies. Science 301, 183–186. doi: 10.1126/science.1084507
Pereira, T. A., Vieira, S. A., Oliveira, R. S., Antiqueira, P. A. P., Migliorini, G. H., Romero, G. Q., et al. (2022). Local drivers of heterogeneity in a tropical forest: epiphytic tank bromeliads affect the availability of soil resources and conditions and indirectly affect the structure of seedling communities. Oecologia 199, 205–215. doi: 10.1007/s00442-022-05179-8
Petermann, J. S., and Gossner, M. M. (2022). Aquatic islands in the sky: 100 years of research on water-filled tree holes. Ecol. Evol. 12, e9206. doi: 10.1002/ece3.9206
Pinos, J., Llorens, P., and Latron, J. (2022). High-resolution temporal dynamics of intra-storm isotopic composition of stemflow and throughfall in a Mediterranean Scots pine forest. Hydrol. Process. 36. doi: 10.1002/hyp.14641
Ponette-González, A. G. (2021). Accumulator, transporter, substrate, and reactor: multidimensional perspectives and approaches to the study of bark. Front. For. Glob. Change 4, 716557. doi: 10.3389/ffgc.2021.716557
Ponette-González, A. G., Chen, D., Elderbrock, E., Rindy, J. E., Barrett, T. E., Luce, B. W., et al. (2022). Urban edge trees: urban form and meteorology drive elemental carbon deposition to canopies and soils. Environ. Pollut. 314, 120197. doi: 10.1016/j.envpol.2022.120197
Ponette-González, A. G., Van Stan, J. T., and Magyar, D. (2020). “Things seen and unseen in throughfall and stemflow,” in Precipitation Partitioning by Vegetation: A Global Synthesis, eds J. T. Van Stan II, E. D. Gutmann, and J. Friesen (Cham: Springer International Publishing), 71−88. doi: 10.1007/978-3-030-29702-2_5
Ponette-Gonzalez, A. G., Weathers, K. C., and Curran, L. M. (2010). Water inputs across a tropical montane landscape in Veracruz, Mexico: synergistic effects of land cover, rain and fog seasonality, and interannual precipitation variability. Glob. Chang. Biol. 16, 946–963. doi: 10.1111/j.1365-2486.2009.01985.x
Porada, P., Van Stan, J. T., and Kleidon, A. (2018). Significant contribution of non-vascular vegetation to global rainfall interception. Nat. Geosci. 11, 563. doi: 10.1038/s41561-018-0176-7
Ptatscheck, C., Milne, P. C., and Traunspurger, W. (2018). Is stemflow a vector for the transport of small metazoans from tree surfaces down to soil? BMC Ecol. 18, 43. doi: 10.1186/s12898-018-0198-4
Ptatscheck, C., and Traunspurger, W. (2015). Meio- and macrofaunal communities in artificial water-filled tree holes: effects of seasonality, physical and chemical parameters, and availability of food resources. PLoS ONE 10, e0133447. doi: 10.1371/journal.pone.0133447
Qin, J., Wang, B., Wu, Y., Lu, Q., and Zhu, H. (2021). Identifying pine wood nematode disease using UAV images and deep learning algorithms. Remote Sens. 13, 162. doi: 10.3390/rs13020162
Rantala, P., Aalto, J., Taipale, R., Ruuskanen, T. M., and Rinne, J. (2015). Annual cycle of volatile organic compound exchange between a boreal pine forest and the atmosphere. Biogeosciences 12, 5753–5770. doi: 10.5194/bg-12-5753-2015
Rebollar, E. A., Martínez-Ugalde, E., and Orta, A. H. (2020). The amphibian skin microbiome and its protective role against chytridiomycosis. Herpetologica 76, 167. doi: 10.1655/0018-0831-76.2.167
Rindy, J. E., Ponette-Gonz?lez, A. G., Barrett, T. E., Sheesley, R. J., and Weathers, K. C. (2019). Urban trees are sinks for soot: elemental carbon accumulation by two widespread oak species. Environ. Sci. Technol. 53, 10092–10101. doi: 10.1021/acs.est.9b02844
Rodayan, A., Afana, S., Segura, P. A., Sultana, T., Metcalfe, C. D., Yargeau, V., et al. (2016). Linking drugs of abuse in wastewater to contamination of surface and drinking water. Environ. Toxicol. Chem. 35, 843–849. doi: 10.1002/etc.3085
Sadeghi, S. M. M., Gordon, A. G., and Van Stan, J. T. (2020). “A global synthesis of throughfall and stemflow hydrometeorology,” in Precipitation Partitioning by Vegetation: A Global Synthesis, eds J. T. Van Stan II, E. D. Gutmann, and J. Friesen (Cham: Springer International Publishing), p. 49–70. doi: 10.1007/978-3-030-29702-2_4
Schleuning, M., Fründ, J., Schweiger, O., Welk, E., Albrecht, J., Albrecht, M., et al. (2016). Ecological networks are more sensitive to plant than to animal extinction under climate change. Nat. Commun. 7, 13965. doi: 10.1038/ncomms13965
Schmidl, J., Sulzer, P., and Kitching, R. L. (2008). The insect assemblage in water filled tree-holes in a European temperate deciduous forest: community composition reflects structural, trophic and physicochemical factors. Hydrobiologia 598, 285–303. doi: 10.1007/s10750-007-9163-5
Seino, Y., Suzuki, Y., and Sogawa, K. (1996). An ovicidal substance produced by rice plants in response to oviposition by the whitebacked planthopper, Sogatella furcifera (HORVATH) (Homoptera: Delphacidae). Appl. Entomol. Zool. 31, 467–473. doi: 10.1303/aez.31.467
Shuttleworth, W. J. (1976). Experimental evidence for the failure of the Penman-Monteith equation in partially wet conditions. Boundary Layer Meteorol. 10, 91–94. doi: 10.1007/BF00218726
Son, Y. (2001). Non-symbiotic nitrogen fixation in forest ecosystems. Ecol. Res. 16, 183–196. doi: 10.1046/j.1440-1703.2001.00385.x
Song, Z., Kennedy, P. G., Liew, F. J., and Schilling, J. S. (2017). Fungal endophytes as priority colonizers initiating wood decomposition. Funct. Ecol. 31, 407–418. doi: 10.1111/1365-2435.12735
Sprenger, M., Stumpp, C., Weiler, M., Aeschbach, W., Allen, S. T., Benettin, P., et al. (2019). The demographics of water: a review of water ages in the critical zone. Rev. Geophys. 57, 800–834. doi: 10.1029/2018RG000633
Sridhar, K. F. (2009). “Fungi in the tree canopy: an appraisal,” in Applied Mycology (Wallingford: CABI), 73–91. doi: 10.1079/9781845935344.0073
Srivastava, D. S., Céréghino, R., Trzcinski, M. K., MacDonald, A. A. M., Marino, N. A. C., Mercado, D. A., et al. (2020). Ecological response to altered rainfall differs across the Neotropics. Ecology 101, e02984. doi: 10.1002/ecy.2984
Stadler, B., and Michalzik, B. (1998). Aphid infested Norway spruce are “hot spots” in throughfall carbon chemistry in coniferous forests. Can. J. For. Res. 28, 1717–1722. doi: 10.1139/x98-153
Stewart, W. D. P. (1969). Biological and ecological aspects of nitrogen fixation by free-living micro-organisms. Proc. R. Soc. Lond. B. Biol. Sci. 172, 367–388. doi: 10.1098/rspb.1969.0027
Stork, N. E., Adis, J., and Didham, R. K. (1996). Canopy Arthropods. New York, NY: Chapman and Hall.
Su, P., Granholm, K., Pranovich, A., Harju, L., Holmbom, B., Ivaska, A., et al. (2013). Sorption of metal ions from aqueous solution to spruce bark. Wood Sci. Technol. 47, 1083–1097. doi: 10.1007/s00226-013-0562-7
Suzuki, K. (2006). Characterisation of airborne particulates and associated trace metals deposited on tree bark by ICP-OES, ICP-MS, SEM-EDX and laser ablation ICP-MS. Atmos. Environ. 40, 2626–2634. doi: 10.1016/j.atmosenv.2005.12.022
Tatsumi, C., Azuma, W. A., Ogawa, Y., and Komada, N. (2021). Nitrogen availability and microbial communities of canopy soils in a large Cercidiphyllum japonicum tree of a cool-temperate old growth forest. Microb. Ecol. 82, 919–931. doi: 10.1007/s00248-021-01707-w
Taylor, J. E., and Whitelaw, C. A. (2001). Signals in abscission. New Phytol. 151, 323–340. doi: 10.1046/j.0028-646x.2001.00194.x
Teachey, M. E., Pound, P. T., Ottesen, E. A., and Van Stan, J. T. (2018). Bacterial community composition of throughfall and stemflow. Front. For. Glob. Change 1, 7. doi: 10.3389/ffgc.2018.00007
Van Stan, J. T., and Friesen, J. (2020). “Precipitation partitioning, or to the surface and back again: historical overview of the first process in the terrestrial hydrologic pathway,” in Precipitation Partitioning by Vegetation: A Global Synthesis, eds J. T. Van Stan II, E. D. Gutmann, and J. Friesen (Cham: Springer International Publishing), 1–16. doi: 10.1007/978-3-030-29702-2_1
Van Stan, J. T., and Gordon, D. A. (2018). Mini-review: stemflow as a resource limitation to near-stem soils. Front. Plant Sci. 9, 248. doi: 10.3389/fpls.2018.00248
Van Stan, J. T., Hildebrandt, A., Friesen, J., Metzger, J. C., and Yankine, S. A. (2020a). Spatial variablity and temporal stability of local net precipitation patterns,” in Precipitation Partitioning by Vegetation: A Global Synthesis, eds J. T. Van Stan II, E. D. Gutmann, and J. Friesen (Cham: Springer International Publishing), 89–104. doi: 10.1007/978-3-030-29702-2_6
Van Stan, J. T., Morris, C. E., Aung, K., Kuzyakov, Y., Magyar, D., Rebollar, E. A., et al. (2020b). “Precipitation partitioning - hydrologic highways between microbial communities of the plant microbiome?” in Precipitation Partitioning by Vegetation: A Global Synthesis, eds J. T. Van Stan II, E. D. Gutmann, and J. Friesen (Cham: Springer International Publishing), 229–252. doi: 10.1007/978-3-030-29702-2_14
Van Stan, J. T., Ponette-González, A. G., Swanson, T., and Weathers, K. C. (2021). Throughfall and stemflow are major hydrologic highways for particulate traffic through tree canopies. Front. Ecol. Environ. 19, 2360. doi: 10.1002/fee.2360
Van Stan, J. T., and Pypker, T. G. (2015). A review and evaluation of forest canopy epiphyte roles in the partitioning and chemical alteration of precipitation. Sci. Total Environ. 536, 813–824. doi: 10.1016/j.scitotenv.2015.07.134
Van Stan, J. T., and Stubbins, A. (2018). Tree-DOM: dissolved organic matter in throughfall and stemflow. Limnol Oceanogr. Lett. 3, 199–214. doi: 10.1002/lol2.10059
Van Stan, J. T. Jr., Levia, D. F., Inamdar, S. P., Lepori-Bui, M., and Mitchell, M. J. (2012). The effects of phenoseason and storm characteristics on throughfall solute washoff and leaching dynamics from a temperate deciduous forest canopy. Sci. Total Environ. 430, 48–58. doi: 10.1016/j.scitotenv.2012.04.060
Victoriano-Romero, E., García-Franco, J. G., Mehltreter, K., Valencia-Díaz, S., Toledo-Hernández, V. H., and Flores-Palacios, A. (2020). Epiphyte associations and canopy soil volume: nutrient capital and factors influencing soil retention in the canopy. Plant Biol. 22, 541–552. doi: 10.1111/plb.13080
Vidkjær, N. H., Wollenweber, B., Jensen, K.-,M. V., Ambus, P. L., Offenberg, J., and Fomsgaard, I. S. (2016). Urea in weaver ant feces: quantification and investigation of the uptake and translocation of urea in Coffea arabica. J. Plant Growth Regul. 35, 803–814. doi: 10.1007/s00344-016-9586-1
Walker, E., Kaufman, M., and Merritt, R. (2010). An acute trophic cascade among microorganisms in the tree hole ecosystem following removal of omnivorous mosquito larvae. Community Ecol. 11, 171–178. doi: 10.1556/ComEc.11.2010.2.5
Watanabe, K., Kohzu, A., Suda, W., Yamamura, S., Takamatsu, T., Takenaka, A., et al. (2016). Microbial nitrification in throughfall of a Japanese cedar associated with archaea from the tree canopy. Springerplus 5, 1596. doi: 10.1186/s40064-016-3286-y
Weidhaas, J., Anderson, A., and Jamal, R. (2018). Elucidating waterborne pathogen presence and aiding source apportionment in an impaired stream. Appl. Environ. Microbiol. 84, e02510-17. doi: 10.1128/AEM.02510-17
Weiler, M., and McDonnell, J. (2004). Virtual experiments: a new approach for improving process conceptualization in hillslope hydrology. J. Hydrol. 285, 3–18. doi: 10.1016/S0022-1694(03)00271-3
Wilkinson, J. L., Boxall, A. B. A., Kolpin, D. W., Leung, K. M. Y., Lai, R. W. S., Galbán-Malagón, C., et al. (2022). Pharmaceutical pollution of the world's rivers. Proc. Nat. Acad. Sci. 119. doi: 10.1073/pnas.2113947119
Wittman, P. K. (2000). The animal community associated with canopy bromeliads of the lowland Peruvian Amazon rain forest. Selbyana 21, 48–51. doi: 10.2307/41760052
Xu, X., Yu, X., Mo, L., Xu, Y., Bao, L., Lun, X., et al. (2019). Atmospheric particulate matter accumulation on trees: a comparison of boles, branches and leaves. J. Clean. Prod. 226, 349–356. doi: 10.1016/j.jclepro.2019.04.072
Yanoviak, S. P. (2001). The macrofauna of water-filled tree holes on Barro Colorado Island, Panama1. Biotropica 33, 110–120. doi: 10.1646/0006-3606(2001)033[0110:TMOWFT]2.0.CO;2
Yee, D. A., Yee, S. H., Kneitel, J. M., and Juliano, S. A. (2007). Richness–productivity relationships between trophic levels in a detritus-based system: significance of abundance and trophic linkage. Oecologia 154, 377–385. doi: 10.1007/s00442-007-0837-5
Yeend, W., and Shawe, D. R. (1989). “Gold in placer deposits,” in Geology and the Resources of Gold in the United States, eds D. R. Shawe, R. P. Ashley, and L. M. H. Carter (Reston, VA: United States Geological Survey), G1–G13.
Zaynab, M., Fatima, M., Abbas, S., Sharif, Y., Umair, M., Zafar, M. H., et al. (2018). Role of secondary metabolites in plant defense against pathogens. Microb. Pathog. 124, 198–202. doi: 10.1016/j.micpath.2018.08.034
Zhang, J., Hu, J., Lian, J., Fan, Z., Ouyang, X., Ye, W., et al. (2016). Seeing the forest from drones: testing the potential of lightweight drones as a tool for long-term forest monitoring. Biol. Conserv. 198, 60–69. doi: 10.1016/j.biocon.2016.03.027
Zotz, G. (ed.)., (2016). “Functional anatomy and morphology,” in Plants on Plants–The Biology of Vascular Epiphytes (Cham: Springer), 67–93. doi: 10.1007/978-3-319-39237-0_4
Zotz, G., Leja, M., Aguilar-Cruz, Y., and Einzmann, H. J. R. (2020). How much water is in the tank? An allometric analysis with 205 bromeliad species. Flora 264, 151557. doi: 10.1016/j.flora.2020.151557
Keywords: precipitation partitioning, canopy water balance, stemflow, forest, interception, rainfall, fog, epiphytes
Citation: Mabrouk AI, Gordon DA, Gotsch SG and Van Stan JT II (2022) Conceptual analysis: What signals might plant canopies send via stemflow? Front. Water 4:1075732. doi: 10.3389/frwa.2022.1075732
Received: 20 October 2022; Accepted: 24 November 2022;
Published: 20 December 2022.
Edited by:
Ty Ferre, University of Arizona, United StatesReviewed by:
Brady Adams Flinchum, CSIRO Land and Water, AustraliaYafeng Zhang, Northwest Institute of Eco-Environment and Resources (CAS), China
Chuan Yuan, Zhejiang Agriculture and Forestry University, China
Copyright © 2022 Mabrouk, Gordon, Gotsch and Van Stan. This is an open-access article distributed under the terms of the Creative Commons Attribution License (CC BY). The use, distribution or reproduction in other forums is permitted, provided the original author(s) and the copyright owner(s) are credited and that the original publication in this journal is cited, in accordance with accepted academic practice. No use, distribution or reproduction is permitted which does not comply with these terms.
*Correspondence: John T. Van Stan II, professor.vanstan@gmail.com