- 1Academia Nacional de Ciencias, Córdoba, Argentina
- 2Focal Point, Water Program of the Inter-American Network of Academies of Science, Córdoba, Argentina
River discharge time series, originally recorded to anticipate floods and water scarcity, later became indispensable to design hydroelectric dams. Presently, discharge monitoring aids in detecting climatic and environmental change, because the discharge and quality of river water are functions of many climatic, biological, geological, and topographic variables coexisting in the basin. Climate change is altering the atmospheric precipitation distribution pattern-both, in time and space-as well as the occurrence of extreme climatic events. It is important the global upgrading of river gauging networks to unveil hydrological trends and changing atmospheric patterns. In so doing, discharge monitoring stations–and the resulting time series-may be, as well, invaluable in revealing the role played by significant environmental variables.
Introduction
Rivers are essential features for our planet's habitability. Following the etymology of the term, it appears that in ancient times a river was mostly defined by the channel in which water flows rather than denoting the water it conveys1. This was possibly linked to the fact that rivers were frequently used as natural borders separating different communities, not necessarily coexisting in friendly terms. In any case, the immense significance reached by rivers in the development of humanity became apparent soon in its history. Since early times, rivers were easily reachable sources of freshwater, relatively inexpensive means of transportation, and effortlessly accessible sources of proteins. Unfortunately, these obvious benefits contrasted with disastrous floods or droughts with which, with variable frequency, rivers showed their might. In order to foresee times of hunger, abundance, or catastrophe, Egyptians gauged the Nile River by means of nilometers2, measuring river water height (i.e., a discharge proxy) for millennia (e.g., Eltahir and Wang, 1999 and references therein). These gauging structures continued to be useful until modern times, when the Nile's natural flow was modulated, mainly by the Aswan High Dam.
The human response to such fluvial unpredictability was the building of barrages to prevent devastating floods, to irrigate crops, or to store water to ameliorate foreseeable water scarcity3. The construction of structures across rivers to hold back water led to the development of more truthful methods to approach river flow than measuring river water height (i.e., as in Egypt's nilometers), keeping historical discharge records, so as to fully understand and forecast river discharge variability. Adding to the original objectives, the current worldwide environmental crisis has shown that river discharge monitoring is, as well, a meaningful tool to interpret changes occurring within drainage basins. To meet future global challenges in such context, many countries should modernize to state-of-the-art river flow monitoring networks, employing techniques like acoustic Doppler instrumentation, early-warning systems, remote sensing, real-time data delivery4, or improving rainfall-runoff modeling to assist in the assessment of climate and land use changes (e.g., Anderson et al., 2005). The previous observations turn out to be particularly significant because the existing river flow databases [e.g., Global Runoff Data Center (GRDC), and UNH CSRC Global River Discharge (RivDIS)], generally supply partial images on the current status of discharge monitoring global networks, limiting data to a subset of all the available stations in each country (i.e., those considered significant, usually supplying the system's total discharge), and almost always omitting information on the methodology used to generate the databases.
The target of this policy brief is to show that even though the significance of the initial goals of river flow monitoring still preserve their original worth, the resulting time series are valued means to unveil climate-induced discharge trends and to detect environmental problems within the basin. Europe's recent disastrous flooding took most hydrologists by surprise5, reiterating that climate change is a major challenge for humanity and, hence, it is of utmost importance to build robust data bases so that controlling floods, water scarcity, and preserving water quality are actions that should be based on the most advanced technologies, securing efficient real-time data availability to all parties concerned.
The Significance of Rivers in the Hydrological Cycle
About 2.5 percent of the Earth's water mass (~1.4 109 km3) corresponds to freshwater. Rivers and freshwater lakes – undoubtedly, the most accessible water sources - store ~0.007 percent of the total water6 available for human consumption. Milliman and Farnsworth (2011) calculated a mean riverine global discharge of ~36000 km3 y−1, of which 55 percent is jointly supplied by Asia and Oceania (~11000 km3 y−1) and the Amazon River (~8600 km3 y−1). This skewed discharge distribution is determined by atmospheric precipitations, which are significantly higher in the latitudinal belt comprised between ~20°N and ~20°S (e.g., Dai and Trenberth, 2002; Dai et al., 2009; Milliman and Farnsworth, 2011).
Besides their role as freshwater suppliers, rivers contribute to support dynamic and assorted life-sustaining systems, in the rivers themselves, and in estuaries and the contiguous marine environment. In spite of the ostensible scarcity of freshwater and the almost insignificant relative presence of rivers in the freshwater budget, they supply ~50 per cent of the water humanity has at its disposal to fulfill all its drinking needs, and ~57 per cent of the water used for irrigation (UNESCO WWAP, 2021)7. Unquestionably, these figures put in the correct perspective the importance of freshwater resources for the future of humanity, particularly in a climate change scenario8.
Policy Options and Implications
The topmost factor that might affect river flow in a drainage basin would be a supply of water, thus making weather9 and climate—i.e., briefly described as “the statistics of weather” (Von Storch, 2005)-, important items to consider. Even with adequate water supply, river discharge and runoff might be influenced in a number of ways by the features (i.e., natural or caused by humans) predominant in drainage basins. Rivers, delivering freshwater and the byproducts of continental denudation to world oceans, integrate all the involved exogenous processes at continental scale. The physical characteristics affecting river discharge and runoff, has been traditionally divided in two distinct classes: (a) conditions inherent in the natural landscape, and (b) conditions in which nature has been transformed by the human use of the land. Basin elevation and orientation, topography (shape and slope), geology, and soil type are the more conspicuous natural features. There are few places in the planet where vegetation remains pristine. Deforestation, agriculture or urban developments are the practices having the most general influence on natural drainage, while dam construction and waterway alteration (i.e., channelization) impact on river flow dynamics. Although they are not frequently included as relevant factors intervening in basin's system, rate of change and uncertainty are important in assessing low-(e.g., climatic fluctuations, topography, soil types) and high-frequency (e.g., weather variability, vegetation cover, land use) processes affecting drainage basins as integral units (e.g., Koutsoyiannis, 2013).
Water quantity goes hand in hand with its quality. Unfortunately, many years ago, humankind guiltlessly assumed that rivers were unproblematic means of getting rid of waste. As years passed and human beings progressed in their welfare, recreation was added to the list of benefits rendered by rivers although, concurrently, pollution remains a largely unsolved problem on a worldwide basis (e.g., Issakaa and Ashrafa, 2017: Sasakova et al., 2018). Recently, the water quality scenario in the Americas has been thoroughly surveyed (IANAS, 2019), and international agencies, like UNEP, FAO, and UNESCO, are continuously dealing with this menace, seeking to raise worldwide concern and find innovative solutions10.
Climate, being the most important variable governing riverine flow incorporates, not only the long-term moisture supply, but also the day-to-day weather pattern that governs the timing and total quantity of water delivered to drainage basins by the atmosphere. Included here are storm types, typical duration and intensity of rainfall or snowfall, distribution of precipitation over the drainage basin, and reliability of precipitation over a period of years, all of which are perceived as significantly distorted by climate change. Extended (e.g., longer than 50 years) precipitation or river flow time series frequently exhibit non-stationary properties and past climate may no longer be considered as an accurate guide to present or future climate.
It is clear, then, that floods and droughts are primarily determined by climate. However, floods are frequently affected by physical conditions within the drainage basin, sometimes acting in synergy with rainfall (e.g., increased runoff triggered by deforestation11). On the other hand, efficient management of the vegetation cover may play a favorable role maintaining base flow in streams and rivers during droughts (Olsson et al., in press).
In the late 19th and early 20th centuries, technology added hydroelectric power to the benefits already assumed as supplied by these impressive structures built across rivers to control water flow. Later, around 1960s, dam building was increasingly questioned when environmental awareness began to grow all over the world, showing that dam construction also carried undesirable consequences to the environment. Anyhow, population growth, economic development, the need to close the electricity access breach, and last but not least, the ever-increasing use of fossil fuels—and their ill consequences—promoted a renovated growth in the construction of dams. Over 3,700 major dams (i.e., larger than 1 MW) were in the planning stage or under construction in the last decade and, at the same time, our planet's left over free-flowing large rivers have diminished by ~21% (Zarfi et al., 2014).
At the beginning of the 20th century, the goal behind locating discharge-measuring gauging stations in rivers was the long-term assessment of discharge variability, directly applied to flood and drought management. Building dams previously requires–among many other prerequisites-extended and reliable information on discharge irregularity, focusing on the frequency and volume of floods and the severity of droughts. It follows that the location of such gauging stations along river courses should be determined by suitable sites-from an engineering point of view-for dam construction (e.g., Fell et al., 2018). The current environmental crisis12, however, has added a new perspective to river monitoring: river flow dynamics cast a reliable image on the environmental factors and the changes occurring in the drainage basin. Moreover, lengthy river flow time series allow attaining a historical view on climate change and other environmental modifications. It comes as a logical conclusion, then, that the design of a discharge monitoring network seeking construction of dams may be coincidental or may differ from a network conceived as a tool to gain crucial environmental information. Besides considering technical factors (topographic, hydrological, geological, etc.), which may be common to both, one focuses primarily on the cost-benefit analysis of the forthcoming dam, whereas the other centers in the efficient recording of climatic-environmental information. Relevant examples involving drainage basins may be probing into the effect of a shifting-crop policy, differences in urbanization rate, the impact of selective forest clear-cutting, alterations in river flow not entirely accounted for by meteorological factors, etc.
The Lack of Stationarity in River Discharge Series: the Paraná River Example
The first regular river discharge measurements in England began on the Thames and Lea rivers in the 1880s and, currently, the U.K.'s National River Flow Archive (NRFA) is the focal point for river flow data, supporting hydrological research and water management activities13. The United States Geological Survey (USGS) has been collecting streamflow data for over 100 years, ever since the first streamflow measurements were made in the late 19th century. Although there are a number of discontinued gauging stations, the USGS currently operates about 8,500 streamflow stations, of which more than one-half are telemetered by an earth-satellite-based communications system. Hydrological data are available in real-time for many agencies to conduct water-resources projects and for the National Weather Service (NWS) to forecast floods14. Data from individual stations are frequently used for objectives beyond the original purpose for an individual station, and the undertaking of scientific studies of long-term changes in the hydrologic cycle is among them.
Argentina belongs in the group of countries leading in river discharge data-gathering in South America, since gauging stations began operation early in the 20th century15. Currently, Argentina's national hydrologic network (i.e., Secretaría de Infaestructura y Política Hídrica) operates 339 real-time streamflow gauging stations and 251 conventional ones. Over 200 have ceased operation. There is an uncertain number of additional gauging stations which are operated by other federal (e.g., the Servicio Meteorológico Nacional) or provincial agencies. Data from one of the oldest stations (i.e., Paraná River at Corrientes) was employed to compute the 1904-2003 deseasonalized discharge time series (i.e., data stripped of its seasonal component) and examined by means of the Mann-Kendall trend test (Mann, 1945; Kendall, 1975), finding a significant (p < 0.05) increasing mean trend of ~31 m3 s−1 per year (Pasquini and Depetris, 2007). The seasonal Kendall test (Hirsch et al., 1982) applied to the same 100-year-long series exhibited increasing significant trends (p < 0.001) for the low-water season (i.e., August–November). The same trend test employed in 50-year segments (i.e., 1904–1949, and 1950–2003) showed significant negative trend for the first set (i.e., p < 0.05 in February–March, high discharge months) and a significant (i.e., p < 0.001) positive trend for the second (i.e., August–November) (Pasquini and Depetris, 2007).
Other important aspects are recurring weather patterns, which change the global atmospheric circulation and affect temperature and precipitation across the globe. A leading example is the broadly known El Niño-Southern Oscillation (ENSO), which involves changes in the water temperature in the Pacific Ocean disturbing worldwide weather (e.g., McPhaden et al., 2020). Early in the study of the Southern Oscillation, its teleconnection with the Paraná River flow was explored by Sir Gilbert Walker's research team in the 1920s, then seeking to forecast India's monsoon (e.g., Depetris et al., 1996 and references therein). The hydrological behavior of the Paraná River drainage basin illustrates the dynamic nature of the linkage between weather patterns and river flow. Harmonic analysis showed that the Paraná River at Corrientes exhibited a significant (p < 0.001) inter-annual oscillation in the ~3–7 years range, which is in phase with ENSO events (Pasquini and Depetris, 2007)16. The impact of ENSO-triggered anomalous hydrology is replicated in many other parts of the world (e.g., McPhaden et al., 2020).
Figure 1 illustrates further the valuable information that the investigation of river flow time series can supply. An updated Paraná River discharge time series shows the increasing trend of the deseasonalized series. In the case of the middle and lower Paraná, its mean monthly discharge increased ~3700 m3 s−1 over the 116-year period. Is ENSO-triggered rainfall a relevant factor in the Paraná's flow increase? It seems to be the case. Figure 1 also includes the variability of the Southern Oscillation Index (SOI) for the same period. Sharply negative SOI departures (i.e., El Niño events) frequently determine high flow, whereas positive departures generally correspond to La Niña occurrences in the Pacific, often determining low discharges. Particularly outstanding is the 1982-83 ENSO which brought about extraordinary flooding accompanied by a significant biogeochemical response (e.g., Depetris and Kempe, 1993; Depetris, 2021). Research has shown that there is unevenness in the hydrologic response of some tributaries feeding the main stem (Depetris and Pasquini, 2008; Pasquini and Depetris, 2010). Increased rainfall in the upper catchments appears to be the main factor determining such hydrological behavior (e.g., Zandonadi et al., 2016, and references therein). However, detailed gauging information from selected sites might reveal, for example, the additional impact of land-use changes. Perhaps the most important conclusion emerging from the Paraná's long discharge time series is that it is not stationary and that discharges recorded during the early decades of the 20th century cannot be used to forecast 21st century river flow17.
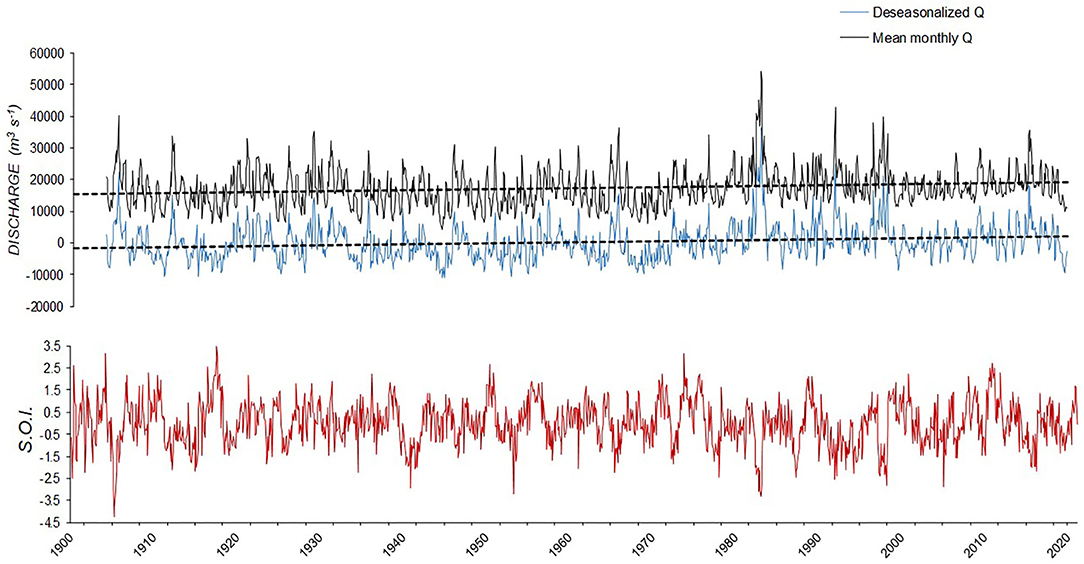
Figure 1. Upper graphs: mean monthly discharge series (Q) of the Paraná River at the Corrientes gauging station (1200 km upstream the mouth) and the corresponding deseasonalized series for the 1904-2020 period. Geometric mean discharge currently reached ~16300 m3s−1. A positive trend (i.e., the slope of ~300 m3s−1per decade showed little change over the last 20 years) resulted in a mean discharge increase of ~3700 m3s−1 over the ~116 year interval. Lower graph: SOI variability19 Notice the coherence between Q and ENSO events, particularly in the 1982-3 exceptional flood.
Concluding Comments and Recommendations
Early in history, barrages were built to prevent the undesirable consequences of both, floods and droughts. Technology devised hydroelectricity around the last quarter of the 19th century and, hence, the regular measurement of river flow began spreading worldwide at propitious sites to gather information which would assist in river discharge forecasting, thus securing continuous power production and water volumes commensurate to demand. The current environmental/climatic crisis, however, has added new challenges -succinctly reviewed below—to the original straightforward goals.
Climate change involves modification of the precipitation distribution patterns and the frequency of occurrence of extreme climatic events, introducing non-stationary properties to river flow series. Thus, it is all the more important to assemble robust hydrological data bases so that the management of rivers for flood control, water scarcity, and maintenance of water quality, can be based on rigorous observations of the frequency and intensity of river discharges. It is important emphasizing here that, Oceania and Africa, for example, account for more than half of the rivers for which Milliman and Farnsworth (2011), in their well-known synthesis, could find no hydrological data. There are, however, numerous problems involved in maintaining worldwide river discharge databases and, although the wide-reaching gauging network has improved significantly since river flow measurement started, it is still far from ideal (e.g., Milliman and Farnsworth, 2011). GRDC, for example, is limited to selected stations, largely defined by participating countries and, furthermore, hydrological data is sometimes incomplete or insufficient, and changes experienced over time in gauging networks may be unreported. GRDC has no information on the instrumentation or methods employed to generate the discharge database18.
Another important aspect to consider is mountain streams. Mountain chains are frequently called the water towers of the world because they often hold the uppermost catchments that supply water to large river systems (e.g., Amazon, Congo, Orinoco). About half of the water used by the world's population originates in mountain chains (Wohl, 2011, and references therein). It follows, then that, under the light of acute climatic modifications, the hydrological scrutiny (i.e., rainfall and stream flow gauging) of such areas must be intensified and optimized, so as to attain sound forecasting capability. The Surface Atmosphere Integrated Field Laboratory (SAIL), high in the Colorado Rockies, is a relevant example of a scientific effort seeking to unveil the causes behind the drought that has gripped the southwestern USA for 2 decades20.
The growth of environmental concern at present has led to a deeper insight into the Earth's “critical zone” (e.g., Richardson, 2020), and resources are now perceived under a different perspective. The water conveyed by rivers, as well as the heterogeneous materials they transport to coastal seas, can supply a wealth of information on the features coexisting and the processes occurring within their drainage basins. Probing into data series allows to investigate hydrologic/environmental high-and low-frequency processes as well as other chemical and physical phenomena.
The recent disastrous floods endured by a significant proportion of the European population, stunned hydrologists and showed that rainfall/streamflow monitoring has never been more important (i.e., investigation suggests that extreme flood events will become more common in Western Europe as the world warms21). This fact tends to get neglected because it is hard to generate support and public understanding for the need to accomplish monitoring on a scale that is significantly larger than the currently reached level. Yet, without such support, it is going to be challenging to devise water management policies based on the modern reality of climate changing over time.
As a final remark, it would be recommended that countries expand and optimize river flow measuring networks, revising the locations of permanent/temporary gauging stations, modernizing measuring methodologies—i.e., hydrology is particularly constrained by the restrictions of in situ sampling (e.g., England, 2005)-, and improving data distribution. The benefits, for example, of real-time data-acquisition, gauging recalibration (e.g., by Doppler acoustic technology), effective use of modeling (e.g., Hidalgo, 2021), the restarting of adequate inoperative stations, will positively impact on our knowledge of the World's hydrology.
While the importance of monitoring has long been recognized, it has assumed today even more standing because of the loss of stationarity and clear evidence that the consequences of climate change are increasingly becoming obvious. Adding the boundary conditions forced by global change to the objectives validating river discharge monitoring implies that gauging station networks may be revised-and, possibly, multiplied and/or relocated-, so as to be useful in assessing recent—or ongoing—environmental impacts.
Author Contributions
The author confirms being the sole contributor of this work and has approved it for publication.
Conflict of Interest
The author declares that the research was conducted in the absence of any commercial or financial relationships that could be construed as a potential conflict of interest.
Publisher's Note
All claims expressed in this article are solely those of the authors and do not necessarily represent those of their affiliated organizations, or those of the publisher, the editors and the reviewers. Any product that may be evaluated in this article, or claim that may be made by its manufacturer, is not guaranteed or endorsed by the publisher.
Acknowledgments
This Policy Brief is one in a series prepared by members and colleagues of the Water Committee of the Inter-American Network of Academies of Science (IANAS). The financial support of the Inter Academy Partnership (IAP) for the realization of this publication is herein acknowledged. The author is grateful to IANAS' Water Program and to its co-Chairs, Henry Vaux and Katherine Vammen, for their valuable assistance in revising the original manuscript. The commentaries of Richard Taylor, Specialty Chief Editor, and two unknown reviewers have been particularly helpful in improving the original manuscript. I am also grateful to Subimal Ghosh, Associate Editor, for the handling of this MS.
Footnotes
1. ^River has its roots in the Latin word riparius (i.e., from ripa, “bank of a river”), and then, from Anglo-Norman rivere, and Old French riviere; rive, French for “shore” or “bank”.
2. ^There are three types of nilometers along the Nile: one is near Cairo, another is in Elephantine Island, at Aswan, and the third kind is a little further north, at the Temple of Kom Ombo.
3. ^Mesopotamians built dams in present-day Jordan (4th century B.C.E.), and Romans, in Spain (1st−2nd century C.E.).
4. ^The U.S. Geological Survey introduced real-time data delivery in 1995.
5. ^https://www.sciencemag.org/news/2021/07/europe-s-deadly-floods-leave-scientists-stunned (accessed August 22, 2021).
6. ^https://www.usgs.gov/special-topic/water-science-school/science/fundamentals-water-cycle? (accessed August 3, 2021).
7. ^According to the FAO, groundwater provides drinking water to at least the remaining 50% of the global population.
8. ^By 2030, the world is projected to face a 40% global water deficit under the business-as-usual climate scenario (UNESCO WWAP, 2021).
9. ^Defined as “the instantaneous state of the atmosphere at a particular location, typically characterized by a range of meteorological variables (or weather elements) such as pressure, temperature, humidity, wind, cloudiness, and precipitation” (Mckendry, 2005, and references therein).
10. ^E.g., https://uneplive.unep.org/media/docs/assessments/unep/wwqa/report/web.pdf (accessed July 30, 2021).
11. ^More than 50% of the Amazonian rainforest (i.e., ~5 106 km2) is in Brazil, where ~19% has been cleared; deforestation decreased after 2004 but forest clearing is rising again (Amigo, 2020).
12. ^https://www.ipcc.ch/library (accessed July 27, 2021).
13. ^https://www.ceh.ac.uk/our-science/projects/national-river-flow-archive (accessed July 7, 2021).
14. ^https://pubs.usgs.gov/fs/2004/3056 (accessed July 7, 2021).
15. ^https://www.argentina.gob.ar/obras-publicas/hidricas/base-de-datos-hidrologica-integrada (accessed June 30, 2021).
16. ^Fourier's squared coherency supported the teleconnection existing between ENSO occurrences and Paraná River flow anomalies.
17. ^Unexpectedly, the Paraná River is currently undergoing the worst drought in over 90 years. The low-discharge trend started in June 2019 and it is still going on.
18. ^Expanding and optimizing global discharge databases is a multifaceted problem and the blueprint for its solution lies beyond the purpose of this policy brief. Extensive international cooperation is, indeed, implicit and should be framed within the current global change crisis.
19. ^http://www.bom.gov.au/climate/influences/graphs/.
20. ^https://www.science.org/news/2021/08/high-colorado-rockies-scientists-launch-search-causes-western-water-woes?utm_campaign=news_daily_2021-08-24&et_rid=742695301&et_cid=3895875 (accessed September 3, 2021).
21. ^https://www.nature.com/articles/d41586-021-02330-y?utm_source=Nature+Briefing&utm_campaign=144d67f668-briefing-dy-20210826&utm_medium=email&utm_term=0_c9dfd39373-144d67f668-44601189 (accessed August 30, 2021).
References
Anderson, M. G., and McDonnell, J. J., (eds.). (2005). Encyclopedia of Hydrological Sciences, Vol. 5, Chichester, SXW: Wiley and Sons. doi: 10.1002/0470848944
Dai, A., Qian, T., Trenberth, K. E., and Milliman, J. D. (2009). Changes in continental freshwater discharge from 1948 to 2004. J. Clim. 22, 2773–2792. doi: 10.1175/2008JCLI2592.1
Dai, A., and Trenberth, K. E. (2002). Estimates of freshwater discharge from continents: latitudinal and seasonal variations. J. Hydrometeor. 3, 660-687. doi: 10.1175/1525-7541(2002)003<0660:EOFDFC>2.0.CO
Depetris, P. J. (2021). Revisiting the effect of extreme flooding on the chemistry of a large floodplain river: the example of Argentina's middle Paraná. Env. Earth Sci. 80:54. doi: 10.1007/s12665-020-09309-4
Depetris, P. J., and Kempe, S. (1993). Carbon dynamics and sources in the Paraná River. Limn. Oceanogr. 387, 382–395. doi: 10.4319/lo.1993.38.2.0382
Depetris, P. J., Kempe, S., Latif, M., and Mook, W. G. (1996). ENSO-controlled flooding in the Paraná River (1904-1991). Naturwissen. 83, 127–129. doi: 10.1007/BF01142177
Depetris, P. J., and Pasquini, A. I. (2008). Riverine flow and lake level variability in Southern South America. EOS 89, 254–255. doi: 10.1029/2008EO280002
Eltahir, E. A. B., and Wang, G. (1999). Nilometers, El Nino, and climate variability. Geophys. Res. Let. 26, 489–492. doi: 10.1029/1999GL900013
England, A. W. (2005). “Sensor principles and remote sensing techniques,” in Encyclopedia of Hydrological Sciences, eds M. G. Anderson (Chichester, SXW: Wiley and Sons), 673–695. doi: 10.1002/0470848944.hsa050
Fell, R., Stapledon, D., Bell, G., and Foster, M. (2018). Geotechnical Engineering of Dams, 2nd edn. London: CRC Press.
Hidalgo, H. G. (2021). Climate variability and change in Central America: what does it mean for water managers. Front. Water 2:1. doi: 10.3389/frwa.2020.632739
Hirsch, R. M., Slack, J. R., and Smith, R. A. (1982). Techniques of trend analysis for monthly water quality data. Water Res. 20, 107–121. doi: 10.1029/WR018i001p00107
IANAS (2019). Water Quality in the Americas. Risks and Opportunities, 624. Available online at: https://ianas.org/wp-content/uploads/2020/07/02-Water-quality-INGLES.pdf (accessed July 19, 2021).
Issakaa, S., and Ashrafa, M. A. (2017). Impact of soil erosion and degradation on water quality: a review. Geol. Ecol. Land. 1:2017. doi: 10.1080/24749508.2017.1301053
Koutsoyiannis, D. (2013). Hydrology and change. Hydrol. Sci. J. 58, 1177–1197. doi: 10.1080/02626667.2013.804626
Mann, H. B. (1945). Non-parametric tests against trend. Econometrica 13, 245–259. doi: 10.2307/1907187
Mckendry, I. G. (2005). “Weather patterns and weather types,” in Encyclopedia of Hydrological Sciences, ed M. G. Anderson (Chichester, SXW: Wiley and Sons), 401–411. doi: 10.1002/0470848944.hsa028
McPhaden, M. J., Santoso, A., and Cai, W, . (eds). (2020). El niño southern oscillation in a changing climate. Washington, DC: Wiley. doi: 10.1002/9781119548164
Milliman, J. D., and Farnsworth, K. L. (2011). River Discharge to the Coastal Ocean. A Global Synthesis. Cambridge: Cambridge University Press. doi: 10.1017/CBO9780511781247
Olsson, L., Barbosa, H., Bhadwal, S., Cowie, A., Delusca, K., and Flores-Renteria, D. (in press). “Land Degradation,” in Climate Change Land: an IPCC Special Report on Climate Change Desertification, Land Degradation, Sustainable Land Management, Food Security, Greenhouse Gas Fluxes in Terrestrial Ecosystems, eds P. R. Shukla, J. Skea, E. Calvo Buendia, V. Masson-Delmotte, H.-O. Pörtner, D. C. Roberts, P. Zhai, R. Slade, S. Connors, R. van Diemen, M. Ferrat, E. Haughey, S. Luz, S. Neogi, M. Pathak, J. Petzold, J. Portugal Pereira, P. Vyas, E. Huntley, K. Kissick, M. Belkacemi, J. Malley. Available online at: https://www.ipcc.ch/srccl/ (accessed July 19, 2021).
Pasquini, A. I., and Depetris, P. J. (2007). Discharge trends and flow dynamics of south American rivers draining the southern Atlantic seaboard: an overview. J. Hydrol. 333, 385–399. doi: 10.1016/j.jhydrol.2006.09.005
Pasquini, A. I., and Depetris, P. J. (2010). ENSO-triggered exceptional flooding in the Paraná River: where is the excess water coming from? J. Hydrol. 383, 186–193. doi: 10.1016/j.jhydrol.2009.12.035
Richardson, J. (2020). “Critical Zone”, in Encyclopaedia of Geochemistry, ed W. M. White (Berlin: Springer), 326–330.
Sasakova, N., Gregova, G, Takacova, D., Mojzisova, J., Papajova, I., Venglovsky, J., et al. (2018). Pollution of surface and ground water by sources related to agricultural activities. Front. Sustain. Food Syst. 2:42. doi: 10.3389/fsufs.2018.00042
UNESCO WWAP (2021). The United Nations World Water Development Report 2021: Valuing Water. UNESCO World Water Assessment Program. https://unesdoc.unesco.org/ark:/48223/pf0000375724 (accessed July 19, 2021).
Von Storch, H. (2005). “Models of global and regional climate,” in Encyclopedia of Hydrological Sciences, ed M. G. Anderson (Chichester, SXW: Wiley and Sons), 477–490. doi: 10.1002/0470848944.hsa035
Wohl, E. (2011). Mountain Rivers Revisited. Washington, DC: American Geophysical Union. doi: 10.1029/WM019
Zandonadi, L., Acquaotta, F., Fratianni, S., and Zavattini, J. A. (2016). Changes in precipitation extremes (Paraná River basin). Theor. Appl. Climat. 123, 741–756. doi: 10.1007/s00704-015-1391-4
Keywords: streamflow, river monitoring, ENSO effect, discharge trend, drainage basin, Paraná river, climatic effect
Citation: Depetris PJ (2021) The Importance of Monitoring River Water Discharge. Front. Water 3:745912. doi: 10.3389/frwa.2021.745912
Received: 22 July 2021; Accepted: 09 September 2021;
Published: 26 October 2021.
Edited by:
Subimal Ghosh, Indian Institute of Technology Bombay, IndiaReviewed by:
Anamitra Saha, Massachusetts Institute of Technology, United StatesJisha Joseph, Indian Institute of Technology Bombay, India
Copyright © 2021 Depetris. This is an open-access article distributed under the terms of the Creative Commons Attribution License (CC BY). The use, distribution or reproduction in other forums is permitted, provided the original author(s) and the copyright owner(s) are credited and that the original publication in this journal is cited, in accordance with accepted academic practice. No use, distribution or reproduction is permitted which does not comply with these terms.
*Correspondence: P. J. Depetris, cGVkcm8uZGVwZXRyaXMmI3gwMDA0MDtnbWFpbC5jb20=