- 1Integrative Freshwater Ecology Group, Centre for Advanced Studies of Blanes (CEAB-CSIC), Girona, Spain
- 2Applied and Environmental Geology Group (GAiA), Department of Environmental Sciences, University of Girona, Girona, Spain
- 3School of Geography, Earth and Environmental Sciences, University of Birmingham, Birmingham, United Kingdom
- 4Department of Evolutionary Biology, Ecology and Environmental Sciences, University of Barcelona, Barcelona, Spain
- 5Division of Hydrologic Sciences, Desert Research Institute, Reno, NV, United States
Fine particulate organic matter (FPOM) accumulated in streambeds is a major component of organic matter budgets in headwater streams and greatly affects productivity and metabolism of stream communities. The spatiotemporal distribution of benthic FPOM in the stream, as well as its quantity and quality, depend on inputs from different source types. These can be natural such as soils, streambanks and riparian vegetation, or anthropogenic such as effluents from wastewater treatment plants (WWTP). In addition, stream flow is a key driver of FPOM dynamics, which influences the balance between its transport and accumulation in the streambed. Yet, the link between FPOM dynamics and its effects on stream metabolism is still largely unknown. The aim of this study was to investigate the influence of stream channel hydromorphology on water transport and streambed accumulation of fine particulate matter (FPM) (mineral and organic fractions), FPOM (organic fraction) and its quality (characterized by %OM, %C, %N and the C:N molar ratio). In addition, we quantified the metabolic activity associated with FPM at the habitat scale, and its potential contribution to whole-reach ecosystem respiration using the resazurin-resorufin bioreactive tracer as a proxy for aerobic respiration. We also characterized water transport and metabolic activity with combined additions of hydrological and bioreactive tracers at the reach scale. The study was conducted in the Cànoves stream (Catalonia, NE Spain) downstream of a WWTP that contains three reaches that were hydromorphologically modified using bioengineering techniques. Slower local velocities at the habitat scale increased accumulation of FPM, but did not influence the spatial variability of its quality. Instead, FPM quality declined further downstream from the WWTP. Accumulation of FPM did not increase metabolic activity, but higher %OM of FPM and lower C:N ratios favored the microbial metabolic activity efficiency (normalized by the gram of FPM). Reach-scale metabolic activity was higher in reaches with higher water exchange rate and longer relative travel times, highlighting hydromorphology as an important driver of microbial metabolic activity at the reach-scale. This demonstrates that the interplay of hydrologic exchange and residence time in streambed sediments associated with the microbial metabolic activity of FPOM can ultimately influence reach-scale metabolic activity.
Introduction
The hyporheic zone (HZ) is a region of the streambed sediments that exchanges water, solutes and fine particles with the overlying water column in streams (Boano et al., 2014). In headwater streams, these vertical hydrologic interactions promote the biogeochemical processing of organic matter and nutrients in the HZ, which can substantially contribute to overall surface water quality and whole-reach ecosystem metabolism (Grimm and Fisher, 1984; Brunke and Gonser, 1997; Boulton et al., 2010; Tank et al., 2010; Boano et al., 2014). However, the specific mechanisms and controlling factors underlying these findings are still poorly understood. Most of the microbial metabolic activity in the HZ occurs within the top few centimeters of the streambed sediments where fine particulate matter (FPM; particles from 1 to 1,000 μm) accumulates (Drummond et al., 2014a,b; Knapp et al., 2017). FPM refers to the total composition of material that deposits on the streambed surface, and thus, it includes both inorganic and organic matter fractions. The organic fraction of the FPM (i.e., fine particulate organic matter, FPOM) can consist of fecal particles from shredder invertebrates as well as fragments of organic particles generated both in streams and adjacent soils (Bundschuh and McKie, 2015). Stream FPOM can also derive from anthrogenic point sources such as wastewater treatment plant (WWTP) effluents which usually act as important sources of particles, dissolved organic carbon and nutrients (Marti et al., 2004; Merbt et al., 2014; Bernal et al., 2020; Kelso and Baker, 2020). Although FPOM can be highly processed organic matter; and therefore, expected to be a more recalcitrant OM source than the dissolved organic fraction, FPOM can act both as a colonizing surface for microorganisms and as a carbon source for microbial activity within streams (Hope et al., 1994; Brugger et al., 2001; Gottselig et al., 2014) and eventually can be more labile than dissolved organic matter (Stutter et al., 2007). The quality of FPOM has also been shown to influence microbial processes in aquatic systems (Stelzer et al., 2003), and the enrichment of FPOM with nutrients can increase respiration rates of bacterial colonization (Tant et al., 2013). Therefore, FPM in the streambed, especially the OM fraction, has a high potential to contribute to in-stream heterotrophic activity (i.e., ecosystem respiration), ultimately influencing the balance between gross primary production and respiration at the whole-reach scale.
The spatiotemporal distribution of benthic FPOM in the stream, as well as its quantity and quality, depend on the characteristics of inputs from different contributing sources, whether natural or anthropogenic, and the quantity and quality of FPOM can vary widely - especially from natural sources. In addition, stream flow influences the rate of vertical hydrologic exchange between the surface water, the streambed interface, and the HZ (Boano et al., 2014). The dynamics of FPOM are also influenced by this hydrological exchange, as FPOM will transport with water in and out of the streambed. Therefore, streambed accumulation of FPOM is subjected to an alternance of immobilization and remobilization events that include a wide range of characteristic retention times from surface to subsurface storage areas (Boano et al., 2014; Drummond et al., 2014b). Furthermore, accumulation of FPOM in the streambed can be increased as a result of changes in the hydromorphological configuration of the stream reach, such as the development of pools along the stream reaches (Drummond et al., 2017). This change can increase the hydrologic retention of stream water along a given reach, known as water transient storage, which can increase the interaction time between main stream flow and biogeochemically reactive sediments (Ensign and Doyle, 2005). Therefore, water transient storage not only favors the water residence time and the interaction between solutes and biologically active FPOM in the streambed (Harvey and Fuller, 1998; Lautz and Fanelli, 2008), but can also influence the dynamics between the transport and accumulation of FPOM. In this sense, the hydromorphology of stream reaches can have an effect of boosting stream ecosystem functioning and metabolism (Bencala and Walters, 1983; Fellows et al., 2006), though how the hydromorphological configuration along the stream controls the transport and accumulation of FPOM, and the consequences of these FPOM dynamics on stream metabolic activity at the habitat and whole-reach scales are still largely unknown. Meyer et al. (2005) showed that urban stream standing stocks of FPOM were relatively low due to high and frequent flushing events associated with urban imperviousness and road density. Multidisciplinary studies combining stream hydrology and biogeochemical processes at the surface water-sediment interface, are thus, essential for improving our mechanistic understanding of the connection between FPOM dynamics and stream metabolism (González-Pinzón et al., 2012).
The aim of this study was to investigate the influence of stream channel hydromorphology on water transport and the accumulation of FPM in the streambed and its quality (i.e., organic matter, carbon (C) and nitrogen (N) content). Moreover, we combined local measurements at the habitat scale and slug injections at the reach scale of a widely used hydrometabolic tracer (resazurin) to quantify the metabolic activity associated with FPM, and its potential contribution to whole-reach ecosystem respiration. The study was conducted in three stream reaches that were experimentally manipulated to change their hydromorphological characteristics and produce three reach-scale scenarios with contrasting water residence times and degrees of hydrologic interaction between the water column and the bioactive streambed surface. Based on the differences in hydromorphological characteristics among the 3 experimental reaches, we predicted that (i) quantity of FPM accumulated in streambed sediments would increase with higher water residence time within the reach, (ii) microbial metabolic activity associated with FPM would be influenced by the quantity and quality of FPM, and (iii) the influence of FPM microbial activity on reach-scale ecosystem respiration would vary among the 3 experimental reaches due to differences in water transport and the interaction between surface stream water and streambed sediments, and the influence of FPM would be higher in the reach with higher water residence time.
Materials and Methods
Study Site Description
The study was conducted in Cànoves stream, near the village of Cànoves i Samalús (41°40′50.86″ N, 2°21′23.81″ E, at 300 m above sea level), which is located NE of Barcelona, Spain (Figure 1A). This 3rd order stream is located in a region with a sub humid Mediterranean climate. Annual average rainfall ranges between 700 and 1,000 mm and mainly occurs in spring and autumn, with low precipitation in the summer months. The field sampling and tracer injections were done in a 450 m stream section located 70 m downstream from the effluent input of the WWTP of Cànoves i Samalús municipality (Figure 1B). This WWTP is the first facility within the catchment to deliver effluent water into the stream. Upstream from the WWTP effluent input, the stream has intermittent flow during the summer months, but it has permanent flow year-round downstream of the WWTP due to constant water inputs from the effluent. Therefore, the effluent acts as a source of solutes and particles to the stream.
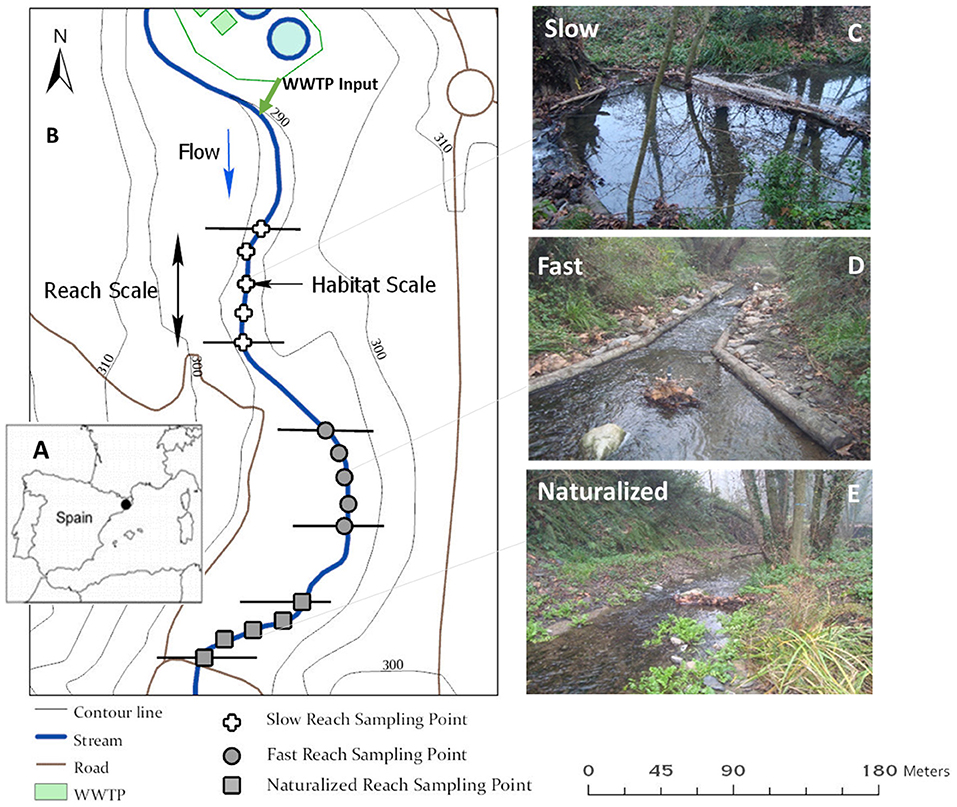
Figure 1. (A) Geographic location of the study site in the NE of Spain. (B) Map of the Cànoves stream 450-m section downstream of the wastewater treatment plant (WWTP) effluent input and the spatial arrangement of the three 75-m reaches, which were experimentally modified to change their hydromorphological characteristics. Different symbols indicate the 5 sampling locations along each reach. (C–E) Pictures of the study reaches showing the bioengineering interventions that resulted in long water residence time and a deep water column (i.e., slow reach; C), short water residence time and a shallow water column (i.e., fast reach; D), and a wide range in water residence times with both deep and shallow water column areas (i.e., naturalized reach; E).
In order to investigate the influence of hydromorphological characteristics of the stream channel on whole-reach FPM dynamics and metabolic activity, the 450 m section downstream of the WWTP input was divided into six ca. 75 m reaches (Figure 1B). Three of those reaches were left without interventions, while the other three reaches were modified using bioengineering techniques. Basically, the technical interventions aimed at modifying water residence time within the reach and the interaction between water column and streambed sediments through changes in stream channel hydromorphological configuration. In the first experimental reach (hereafter, the slow reach), logs were placed transversely in the channel to increase the water residence time while increasing the water column depth relative to the streambed surface area (Figure 1C). In the second experimental reach (hereafter, the fast reach), logs were placed parallel to the riverbanks to act as deflectors, thus increasing the water velocity and turbulence while decreasing the water column depth (Figure 1D). In the third experimental reach (hereafter, the naturalized reach), bioengineering techniques were used to widen the channel, increase the sinuosity, and promote the diversity of streambed habitats. This resulted in a wide range of water residence times within the reach and areas with both increases and decreases in the ratio between the water column depth and the streambed surface area (Figure 1E). The three interspersed reaches without interventions served as buffers and allowed an appropriate assessment of the inputs and outputs from each modified reach.
The bioengineering interventions were done in November 2015. We left the bioengineering structures to settle down and naturalize for about a year. The present study was conducted in May 2017 within a 1-week period. During this period, the mean air temperature showed a steady increase from 15.5 to 20°C. The weather conditions were sunny and the stream baseflow downstream of the WWTP was steady at 13 Ls−1. During the study, the WWTP input contributed to half of the stream flow and the riparian forest along the study section was well-developed, and thus; provided a canopy cover of >75% to the stream channel. The slope of each reach was calculated using the ArcMap 10.2.2 Geographic Information Systems (ArcGIS) program. The Geographic Information System data were obtained online through the Institut Cartogràfic i Geològic de Catalunya.
Field Methods
Habitat-Scale Methods
Samples of FPM accumulated in the streambed sediments (top ~3 cm) were taken from the stream thalweg every 15 m along each study reach (n = 5 samples per reach; Figure 1), following a modified method by Petticrew et al. (2007). Briefly, we placed a 35 cm diameter open-bottom bucket on the streambed to isolate a known area (0.096 m2) and the water column volume above this area. The top 3 cm of sediments were then agitated by hand to resuspend the interstitial FPM into the water volume enclosed in the bucket. We then let the resuspended material settle for 10 s and collected a 2 L sample with a jar. These samples were poured into 250 mL bottles that were taken to the laboratory for further processing (section Characterization of Fine Particulate Matter Accumulated in Streambed Sediments). All samples were immediately placed on ice and covered from sunlight in the field and then kept in the fridge at 4°C until processed. For each FPM sample, the total volume of water isolated within the bucket was calculated using the surface area of the bucket bottom and measuring the water column depth (d) in the bucket. We also measured surface water velocity (v) at each sample location with a handheld current meter and water temperature (T) with a YSI ProODO device.
Reach-Scale Methods
At each of the three bioengineered experimental reaches, a tracer slug injection was conducted using a 2 L solution containing 1 kg of bromide (NaBr) as a conservative tracer and 10 g of resazurin (Raz) as a reactive, metabolic tracer. At each reach, the tracer injection was conducted on the same day prior to the FPM sampling (section Habitat-Scale Methods). Raz is a redox-sensitive phenoxazine dye that loses an oxygen ion irreversibly under reduced conditions to become resorufin (Rru) (Haggerty et al., 2008). The reduction of Raz to Rru, with a color change from purple to pink, is a well-known indicator of the presence of living bacteria, especially aerobic bacteria (Karakashev et al., 2003). Here, we used the Raz-to-Rru transformation along the reach, following Haggerty et al. (2009), as a proxy of the aerobic respiration at the whole reach scale. The tracer solution was injected instantaneously at the head of the reach. At the end of the reach, Br- concentration was measured continuously every 30 s with an ion specific probe (WTW Tetracon 325, model 3310) for 150 min after the injection of the solution to record the breakthrough curve (BTC) passage of the added conservative solute. The BTC was used to estimate the hydraulic characteristics of solute transport, in particular water residence time and hydrologic exchange between surface water and streambed sediment (section Stream Reach Hydraulic Characterization). Moreover, water samples were collected at the end of the reach during the BTC at different time intervals to characterize the Raz slug passage. Water samples were immediately filtered and placed on ice in a dark storage container in the field and transported to the lab to be analyzed for Raz and Rru concentrations within 24 h. The Raz-to-Rru transformation rate was used as a proxy of aerobic respiration (see Characterization of Fine Particulate Matter Accumulated in Streambed Sediments and Whole-Reach Metabolic Activity).
At each experimental reach, we also estimated discharge (Q, in Ls−1) using the velocity-area method (Gordon et al., 2004). For this, we conducted cross-section measurements at each sampling location to measure water depth (d) and water velocity (v) at every 20 cm across the stream wetted width (w). We then averaged the data from the 5 sampling locations along the reach to estimate average d, v, and w. At each reach, Q was the average of the estimations at each sampling location based on transect values of d, v, and w.
Characterization of Fine Particulate Matter Accumulated in Streambed Sediments
Standing Stock and Organic Matter Content
For each habitat-scale sample, the FPM standing stock was estimated by filtering a known volume (~100–200 mL) of the collected samples through a pre-weighed 0.7 μm glass fiber filter (GF/F, supplied by Whatman, UK). Filters were then dried at 50°C for at least 24 h to reach a constant weight and then weighed to measure the dry mass. The FPM standing stock per unit of streambed area (in gm−2) was estimated as the dry mass in the filter scaled from the volume filtered to the total volume of suspended material in the bucket, and divided by the surface area of the streambed sampled. Filters were then placed in the muffle furnace at 500°C for 5 h and then at 50°C for 24 h before reweighing. The organic matter content of the FPM (%OM) was estimated as the difference in weight between the pre- and post- muffled sample. For each habitat-scale sample, we estimated the standing stock of FPOM per unit of streambed area (in gm−2) based on the FPM standing stock and its %OM. The content of C and N of FPM at each sampling location was estimated as a percentage of dry mass (%C and %N) using a CN analyzer (Thermo Finnigan Flash EA 1112, Waltham, MA, U.S.A.). Content of C and N was also expressed as the molar ratio between the two elements (C:N). Data from the 5 habitat-scale sampling locations within the reach were averaged to provide a representative value for each experimental reach. The %OM, %C and %N, as well as the C:N molar ratio, were used to characterize the quality of FPM. Although higher %OM may not be directly indicative of high quality OM (since it can be recalcitrant), we assume that FPM with a higher fraction of OM can be a better resource for microbial activity.
Microbial Metabolic Activity
Rates of microbial metabolic activity (MMA, h−1) associated with FPM samples were estimated using incubations with the Raz-Rru tracer. We poured a well-mixed 40 mL sub-sample of each collected FPM sample into a 50 mL vial. Vials were kept in a cool, dark place until the start of the incubation. In each vial, we added 400 μL of a Raz solution (0.022 g Raz/L), mixed well, and collected 4 mL aliquots of the mixed solutions at 4 time intervals during a 3 h incubation period (t0 = 15 min, t1 = 60 min, t2 = 105 min, and t3 = 165 min). During the incubation, the vials were shaken every 5 min to ensure homogenous conditions in the mixed solution. The 4 mL aliquots were filtered through a 0.7 μm pore-size glass fiber filter (GF/F, supplied by Whatman, UK). The first 1 mL was discarded, while the remaining 3 mL were placed in a vial and left under dark conditions to avoid light effects on Raz decay (Haggerty et al., 2008).
Immediately prior to Raz and Rru analyses, 0.3 μL of pH 8 buffer solution, generated by mixing 1 molar NaH2PO4·H2O with equal parts of 1 molar NaOH, was added to each sample vial. Raz and Rru concentrations were measured on a spectrofluorometer (Shimadzu/RF-5000) with wavelengths of 602 and 616 nm of excitation and emission for Raz and 571 and 585 nm of excitation and emission for Rru (Haggerty et al., 2008). Fluorescence readings were converted to molar concentrations from a calibration curve (r2 = 0.99) using the same lot of Raz for all experiments. This method was also followed to analyze the Raz and Rru concentrations of the in-stream samples from tracer additions (section Reach-Scale Methods).
The normalized turnover of Raz into Rru [i.e., ln(Rru/Raz + P)] over incubation time was used to estimate the rates of MMA. Values of P indicate the production-decay ratio of Rru, which includes effects of irreversible sorption, photo decay, and any other mass losses of Raz and Rru (Haggerty et al., 2014, Baranov et al., 2016). For the timescale of these incubations, we assumed that Raz decays only to Rru, that Rru is stable, and there are no other mass losses. Therefore, we assumed P = 1 (Haggerty et al., 2014; Baranov et al., 2016; Kurz et al., 2017). Under this assumption, the slope of the linear relationship between ln(Rru/Raz + 1) and incubation time since the spike addition of Raz provides a proxy for aerobic MMA. Rates of MMA were converted to be expressed per gram of FPM (h−1 gFPM−1, hereafter referred to as MMA efficiency) to improve the comparison of values among the collected samples, which varied in FPM content due to differences in streambed standing stocks and water column depth, which influenced the dilution of the resuspended streambed sediments in the overlying surface water. Thus, normalizing the rates of MMA by gram of FPM essentially indicates the MMA efficiency associated with the FPM of each sample.
Calculation of Hydraulic Characteristics and Metabolic Activity at Reach Scale
Stream Reach Hydraulic Characterization
The BTC of Br− recorded at the end of each experimental reach was used to calculate the water transport and transient storage parameters within each reach by using a mobile-immobile model (Drummond et al., 2014a). These methods are briefly described below, and further details are provided in the Supplementary Material. Following the fitting procedure outlined in Drummond et al. (2019), we performed several computational experiments with simulations and parameter sets constrained to match the solute BTCs to assess parameter uncertainty associated with the use of the model. We sampled the parameter space using a Latin Hypercube approach (N = 27,000; e.g., Kelleher et al., 2019). Best fit parameters and associated confidence intervals were calculated as ± the standard deviation of the best 0.05% fits.
The model was used to calculate the mean arrival time (τ, min), and the relative travel time, which equals τ/tpeak, with tpeak the time the conservative tracer BTC reached peak concentration. The greater τ/tpeak, the greater the retention of water within transient storage zones (e.g., streambed sediment or surface pools) relative to the preferential flow path (e.g., surface water). Moreover, the mobile-immobile model also provided four key hydraulic parameters for each reach: (1) reach averaged water velocity (vreach, m s−1), (2) solute dispersion (D, m2 s−1), (3) the rate of solute exchange from the water column to the transient storage (TS) zones, or TS exchange rate (Λ, s−1), and (4) the slope of the power-law residence-time distribution of a solute within the water TS zone, an indication of water/solutes retention time in TS (β, unitless), where values closer to 0 relate to longer residence times of solutes within the water TS zone.
Whole-Reach Metabolic Activity
Fluorescence of Raz and Rru in water grab samples collected during the slug additions was measured following the same method as indicated in Microbial Metabolic Activity. Reach-scale metabolic activity was assessed by the Raz-Rru transformation rate (λraz→rru, h−1), which is a proxy of aerobic respiration (González-Pinzón et al., 2012). In each reach, the Raz–Rru transformation rate was calculated as:
where Minj, raz is the total mass of the Raz injected (g), M0, rru is the zeroth moment for Rru at the downstream end of the reach (g), and τ (s) is the mean arrival time (González-Pinzón and Haggerty, 2013).
Statistical Analysis
Differences in the average standing stock of FPM and FPOM, the %OM and the C:N molar ratio as well as the MMA associated with the FPM between the 3 experimental reaches were tested using habitat-scale values (n = 5) with a Kruskal-Wallis rank sum test. This test was followed with pairwise comparisons, using Dunn's-test for multiple comparisons of independent samples. A p-adjustment with Bonferroni was used to reduce a Type I error inflation that leads to a false positive discovery rate. We used non-parametric tests because the data set was relatively small and data were not normally distributed.
Spearman rank correlations were used to examine the influence of habitat-scale hydromorphological characteristics (i.e., water velocity and water column depth) on the standing stock of FPM and its quality (i.e., %OM, %C, %N, and C:N molar ratios). This test was also used to examine whether the variability in MMA efficiency associated with FPM was related to the standing stocks of FPM and the FPM quality. We used MMA efficiency rather than rates of MMA since the former is normalized by the amount of FPM, which facilitates the comparison among samples collected from different locations and reaches. Statistical analyses were done with R software (version 3.3.1; R Project for Statistical Computing, Vienna, Austria).
Results
Habitat and Reach-Scale Physical and Hydraulic Characteristics
The three experimental reaches had similar stream water temperature, while they showed marked differences in their physical and hydraulic characteristics (Table 1). For instance, the slow reach had the shallowest slope, and the presence of small water dams within this reach lowered the average water velocity at the habitat scale, which was 30 and 65% lower than in the naturalized and fast reaches, respectively. In the slow reach, water column depth was increased by 60–65% and wetted width by 12–18% in comparison to the other two reaches. In contrast, the fast and naturalized reaches showed similar slope, water column depth, and wetted width. Stream discharge increased downstream from the slow to the naturalized reach.
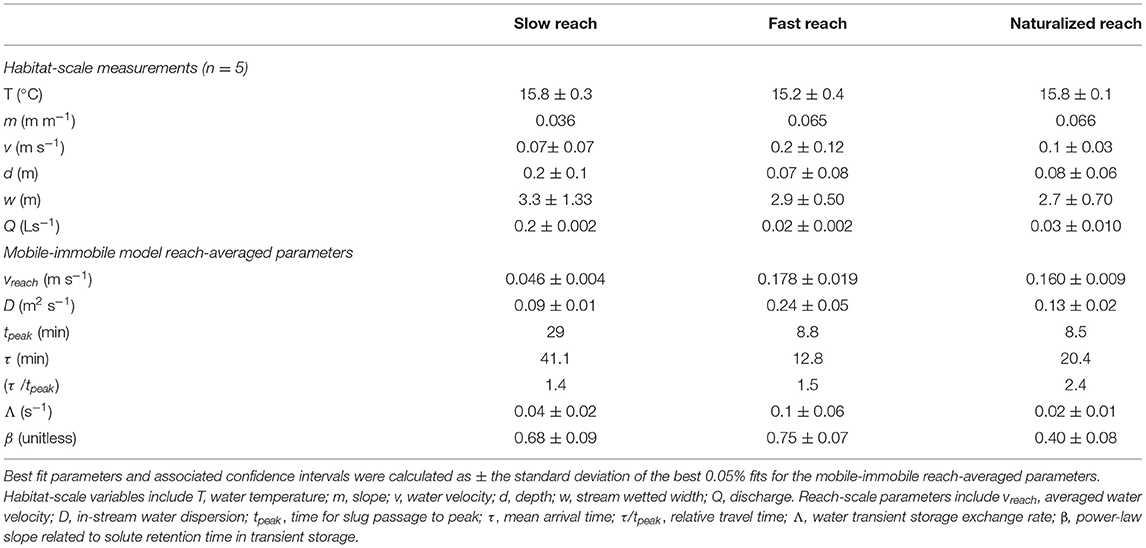
Table 1. Summary of habitat-scale measurements (n = 5, average ± SD and mobile-immobile model reach-averaged parameters (n = 1) that describe the physical and hydraulic characteristics of the three study reaches.
The mobile-immobile model parameters showed differences in solute transport along the three reaches (Table 1). Reach averaged water velocity was lowest in the slow reach and highest in the fast reach, matching the habitat-scale water velocity measurements. Solute dispersion in the reach followed the same pattern among the reaches as water velocity. Solute transport in the water column was similar in the fast and naturalized reaches, as shown by an almost equivalent tpeak, while it was 3 to 4-fold slower in the slow reach (Table 1). The mean arrival time (τ) was between 2 and 3-fold higher in the slow reach compared to the other two reaches, indicating overall slower transport in the slow reach (Table 1). The TS exchange rate (Λ) was ~3 and 7-fold higher in the fast reach than in the slow and naturalized reaches, respectively. The residence time of solutes within the TS zone was longer in the naturalized reach than in the slow and fast reaches as shown by both the highest τ /tpeak and lowest β in the naturalized reach (Table 1).
Characterization of Streambed Fine Particulate Matter Standing Stocks
There were no statistically significant differences in FPM and FPOM standing stocks among the three experimental reaches (Kruskal Wallis comparison test p = 0.2 and p = 0.4, respectively; Figures 2A,B). However, the %OM was 19 and 29% lower in the naturalized reach than in the fast and slow reaches, respectively (Figure 2C). There were no statistically significant differences in the C:N molar ratio among the three reaches (Figure 2D). Yet, the higher median value in the naturalized reach suggests that organic matter was less N enriched compared to the other two reaches.
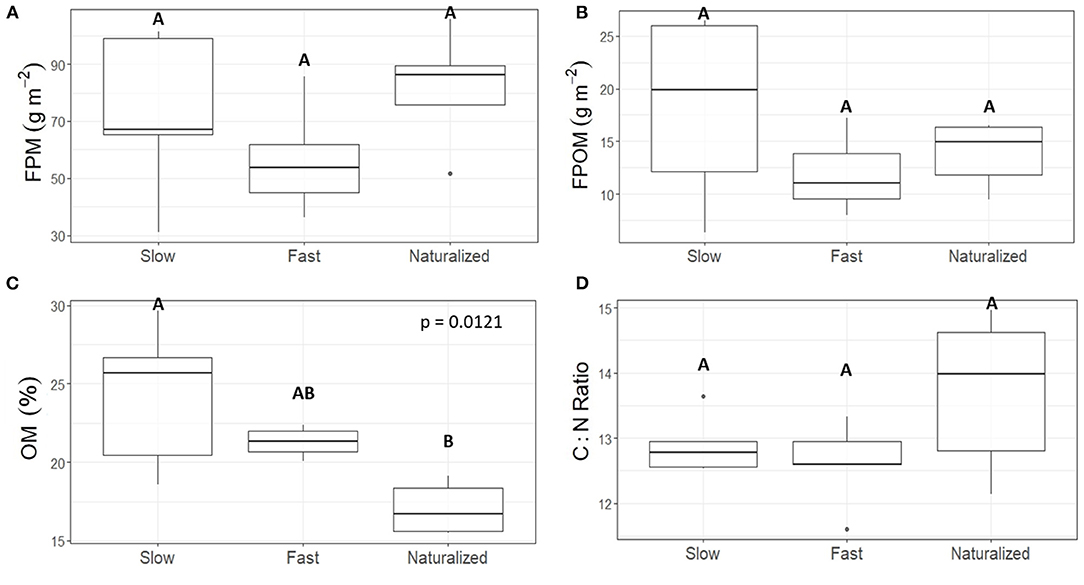
Figure 2. Boxplots showing streambed measurements of (A) fine particulate matter (FPM), (B) fine particulate organic matter (FPOM), (C) percentage organic matter in FPM (%OM), and (D) C:N molar ratio of FPM for each experimental reach. Data come from habitat-scale samples (n = 5) collected at each reach. Results from Kruskal Wallis comparison test among reaches are shown only for statistically significant differences. Same letters indicate no statistical difference among reaches using the Dunn's-test for multiple comparisons.
Water velocity measured at the habitat-scale locations where FPM samples were taken within the reach was not homogenous, with the fast reach showing a wider range in values (Figure 3). Standing stocks of both FPM or FPOM were larger at stream locations with slower velocity and deeper stream depth, and this pattern was consistent regardless of the reach (Figures 3A,B and Supplementary Table 1). There were no statistically significant correlations between %OM and C:N molar ratios of FPM and neither water velocity nor stream depth (Figures 3C,D and Supplementary Table 1).
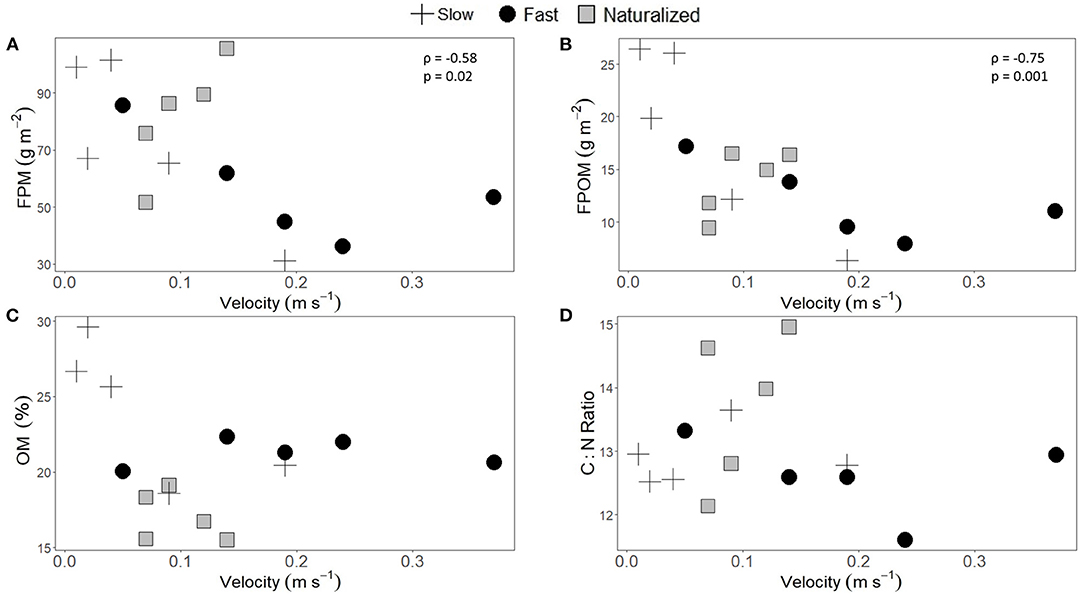
Figure 3. Relationship between habitat-scale measurements of water velocity and streambed standing stocks of (A) fine particulate matter (FPM), (B) fine particulate organic matter (FPOM), (C) percentage organic matter in FPM (% OM), and (D) C:N molar ratios of FPM. Data are from habitat-scale FPM samples (n = 5) collected at the three experimental reaches (crosses, circles, and squares for slow, fast, and naturalized reaches, respectively). Statistical results from Spearman rank correlations (rho, ρ) and the p-value are shown only for statistically significant correlations of the 3 reaches together.
Raz-to-Rru Transformation at the Habitat and Reach Scales
The MMA efficiency associated with streambed FPM differed among the three experimental reaches, being 2-fold lower in the naturalized reach compared with the fast and slow reaches (Kruskal-Wallis rank sum test, p = 0.018, Table 2). Overall, there was no relationship between MMA efficiency and the standing stocks of either FPM or FPOM (Figures 4A,B). The MMA rates associated with streambed FPM were similar among the three experimental reaches (Table 2) and showed no significant correlations with %OM content nor with elemental content and C:N molar ratios (Supplementary Table 1). In contrast, MMA efficiency was positively correlated to %OM (Spearman rank, p = 0.0001, ρ = 0.89) and negatively correlated to C:N molar ratios (Spearman rank, p = 0.02, ρ = −0.61) (Figure 4). Rates of Raz-to-Rru transformation, as a proxy of the metabolic activity, measured at reach scale were highest in the fast reach and lowest in the slow reach (Table 2). For the three reaches, the magnitude of the overall MMA measurements were between 3 and 9-fold lower at the reach scale than at the habitat scale. Yet, the highest rates occurred in the fast reach and the lowest rates in the slow reach, regardless of whether measurements were done at the habitat scale or at the reach scale. In contrast, the MMA efficiency associated with FPM measured at habitat scale showed higher rates at the slow reach.

Table 2. Microbial metabolic activity (MMA) efficiency associated with fine particulate matter measured at habitat scale in each experimental reach (n = 5, average ± SD), MMA at habitat scale (n = 5, average ± SD) and measurement of resazurin-to-resorrufin transformation rate as a proxy of metabolic activity (i.e., aerobic respiration) at reach scale (n = 1).
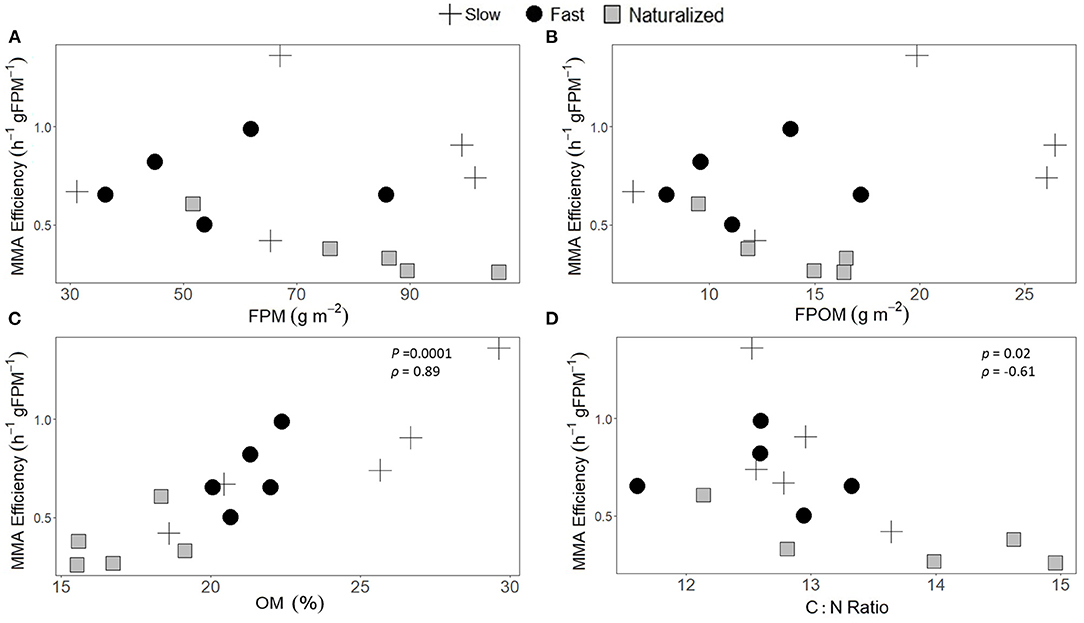
Figure 4. Relationships between microbial metabolic activity (MMA) efficiency associated with (A) fine particulate matter (FPM) accumulated in the streambed and the standing stocks of FPM and (B) fine particulate organic matter (FPOM), as well as (C) the %OM and (D) the C:N ratio of the FPM. Data are from habitat-scale FPM samples (n = 5) collected at the three experimental reaches (crosses, circles, and squares for slow, fast, and naturalized reaches, respectively). Statistical results from Spearman rank correlations (rho, ρ) and the p-value are shown only for statistically significant correlations of the 3 reaches together.
Discussion
This study aimed to investigate the influence of stream hydromorphological characteristics on water transport and the accumulation of FPM in the streambed. In addition, the study examined the variability in quantity, quality, and microbial metabolic activity associated with FPM among three hydromorphological contrasting reaches; and ultimately, what effect these differences had on reach-scale metabolic activity. Our study is one of the few studies to date coupling reach-scale bioreactive transport simultaneously with localized reactivity of the FPM associated with streambed sediments (also see, Knapp et al., 2017). While combining different methodologies at different spatial scales to approach this objective is a challenge in itself, the multidisciplinary character of this study is essential if we are to improve our mechanistic understanding of the connections among stream FPM transport and accumulation, and how it is associated with reach-scale metabolism and nutrient dynamics (González-Pinzón et al., 2012; Ward, 2016). An additional added value of this study is the use of experimentally manipulated reaches to address the objective. The bioengineering interventions were largely successful to produce contrasting hydromorphological characteristics among the three reaches. The three experimental reaches showed differences in many of their hydraulic characteristics measured both at the reach and habitat scales. As a result, the study reaches provided a unique opportunity to not only test the effect of hydromorphological differences on FPM accumulation and distribution at the habitat and reach scales (section Hydromorpohlgoy at the Habitat Scale Control the Accumulation but Not the Quality of Fine Particulate Matter Standing Stocks), but also on the microbial metabolic activity associated with the accumulated FPM. Moreover, we related this activity to reach-scale ecosystem respiration (section Quality and Not Quantity of Streambed FPM Influences Metabolic Activity) to shed light on the role of the activity of benthic FPOM on reach-scale metabolism (section Interaction of In-stream Hydraulics and Streambed Accumulation of FPM Determine Reach-scale Metabolic Activity).
Hydromorphology at the Habitat Scale Control the Accumulation but Not the Quality of Fine Particulate Matter Standing Stocks
On average, accumulation of fine particles (i.e., FPM and its organic matter fraction) in streambed sediments did not vary among the study reaches (Figure 2). However, the wide range of velocities within and across reaches (which was exacerbated by the experimental hydromorphological manipulations) revealed the physical effects of local water velocity on streambed FPM standing stocks. In particular, we found that the spatial variation in accumulation of FPM in the streambed within a reach was negatively related to habitat-scale water velocity. There were also substantial differences in the exchange rate between the surface water and TS zone among study reaches with the highest exchange occurring in the fast reach. In contrast to our expectations, this finding suggests more fine particulate matter transported in and out the streambed in this reach compared to the slow and naturalized reaches. However, this higher exchange in the fast reach was accompanied by lower retention times in the TS zone (lower τ /tpeak and higher β), which likely had an offsetting effect in FPM accumulation in this reach. Overall, our results suggest that not all hydraulic variables equally influenced the accumulation of FPM in the streambed, and that these different effects on FPM accumulation can counterbalance each other. Reach-averaged water velocity, and in particular, the spatial heterogeneity of water velocities within stream reaches was the variable more clearly determining the spatial distribution of FPM. This result is concordant with previous studies showing increased net deposition and accumulation of sediments, detritus, and fine particles in lower velocity zones such as pools and connected lateral cavities (Lemly and Hilderbrand, 2000; Drummond et al., 2017).
While low water velocity favored the accumulation of FPM on the streambed, it did not influence the spatial variability of the quality of FPM within each reach (i.e., %OM and C:N). Instead, we found a decline in %OM of FPM along the study section (including the three study reaches), which was accompanied by an increase in the molar C:N ratio, suggesting a decrease in the quality of FPM (i.e., lower N content relative to C content) from the slow reach to the naturalized reach. This declining pattern in the downstream direction from the WWTP effluent input, together with the similar FPM standing stocks measured at the three reaches, imply two main findings. First, that FPM either supplied by or produced downstream of the WWTP point source was biogeochemically processed along the stream; and second, that FPM was transported and redistributed along each stream reach; otherwise, FPM standing stocks would have also shown a longitudinal decline. Our results are concordant with the idea that FPM accumulation in the streambed is subjected to an alternance of immobilization and remobilization events, which ultimately determines their residence times within streams (Boano et al., 2014; Drummond et al., 2014b). Moreover, our results support that streambed sediments are hot spots of biogeochemical reactions (Jones and Holmes, 1996, Lautz and Fanelli, 2008), and that microbes developed on FPM from either allochthonous or autochthonous sources use this substrate as a carbon source, therefore contributing to a decline in its relative N content (Webster et al., 2009). These results show that while physical controls (such as velocity) influence FPM accumulation, in-stream biogeochemical processing can control the quality of FPM standing stocks.
Quality and Not Quantity of Streambed FPM Influences Metabolic Activity
The FPM standing stocks alone poorly explained the observed variability of the MMA associated with FPM at the habitat scale. In fact, we found no correlation between MMA, as both rates and efficiency, and the amount of FPM or FPOM (Figure 4B and Supplementary Table 2). Our results do not support previous findings relating FPOM quantity to increased respiration, which is usually attributed to FPOM acting as a nutrient-rich substrate for bacterial colonization (Grossart and Ploug, 2001; Mendoza-Lera and Datry, 2017; Mendoza-Lera et al., 2017). In contrast, our results showed that MMA efficiency increased as the quality of FPM increased (i.e. higher %OM and lower C:N ratio) (Figure 4). While the specific sources, composition, and portion of dead vs. alive organic matter in FPOM were unknown in our case, the fact that MMA efficiency increased with decreasing C:N ratio suggests that the FPOM in the Cànoves stream was of relatively high quality. This idea is supported by the low C:N ratios measured at the Cànoves stream (from 12:1 to 15:1), which are substantially lower than those reported for total organic matter in rivers worldwide (from 30:1 to 60:1) (Bauer et al., 2013). In urban streams, FPOM is usually a mixture of terrestrial, autochthonous and wastewater effluent sources, the latter source contributing >50% when hydrological dilution is low (Kelso and Baker, 2020). Therefore, and given that the study reaches were downstream of a WWTP effluent, we propose that this was an important source of FPOM in the Cànoves stream. However, FPOM sourced by WWTP effluents does not necessarily need to be of high quality. For instance, Wang et al. (2018) showed that FPM inputs from a WWTP effluent had a high proportion of humic-like compounds compared to the receiving stream, and that the bioavailability of this material was relatively low. There is also the possibility that high nutrient inputs from the WWTP effluent lead to increased biomass and the production of N-enriched and highly bioavailable FPOM in the Cànoves stream. The increased N content, together with the high %OM (from 15 to 30% of the FPOM), likely contributed to sustained high respiration rates by heterotrophs associated with FPM in streambed sediments, as indicated by the high Raz-to-Rru transformation measured at the habitat scale. This finding supports previous studies showing that streambed FPM availability, in this case FPM quality, can greatly influence the productivity and metabolic activity of stream communities (Webster and Meyer, 1997; Crenshaw et al., 2002; Allan and Castillo, 2007; Nogaro et al., 2007).
Interaction of In-stream Hydraulics and Streambed Accumulation of FPM Determine Reach-Scale Metabolic Activity
Our results indicate mismatches in Raz-to-Rru transformation rates between the habitat and reach scales. First, aerobic metabolic rates associated with FPM accumulated in streambed (MMA) were several fold higher than those measured at the reach scale. Second, patterns of Raz-to-Rru transformation across the three stream reaches did not hold between the two spatial scales. For instance, streambed sediments from the slow reach showed the highest MMA efficiency rates (0.82 h−1gFPM−1) but the lowest reach-scale Raz-to-Rru transformation rates (0.09 h−1). These differences can be at least partially explained by the different approaches used. Metabolic activity from localized sampling of the top few centimeters of the HZ accounts for aerobic respiration at the surface water-sediment interface, while whole-reach injections of hydrometabolic tracers represents overall metabolic activity by stream heterotrophs including not only bacteria in sediment FPM, but also in other streambed substrate and also secondary producers (Harvey et al., 2013; Peipoch et al., 2016). Moreover, while the former approach informs about the potential microbial metabolic activity associated with hot spots of biogeochemical reactivity in the streambed (i.e., FPM accumulation), the latter approach is related to actual aerobic respiration rates at reach scale, which were far below MMA efficiency rates at habitat scale. These results suggest streambed sediments can act as hot spots of microbial metabolic activity within the stream, and yet, can have a relatively small influence on whole-reach aerobic respiration. This disconnection between observational scales can be explained by the different degree in connectivity between the water column and the streambed sediments among the different hydromorphologically modified reaches, or in other words, by a different relationship between hydrological and functional connectivity at the habitat and reach scales in the different study reaches.
The slow reach was the one showing the highest rates of microbial metabolic activity associated with FPM in streambed sediments. With slow water column velocities behind the placement of the transversal logs, fine particle standing stocks accumulate in greater quantity, as more of the fine particles come out of suspension from gravitational settling and accumulate on the streambed. The smaller particle size increases surface area for attachment of microbes and subsequent development of biofilms (Bott and Kaplan, 1985; Kondratieff and Simons, 1985). This fact, combined with the nutrient rich fine particles sourced from the WWTP, can explain the higher microbial activity per gram of FPM within the slow reach. However, this reach showed the slowest water transport (i.e., short hydrologic retention times) and a moderate water exchange rate with the transient storage areas, compared to the other reaches. Moreover, the slow reach showed the shallowest slope, low water velocities and deep average depth, which resulted in an increased accumulation of sediments but also in a lower stream turbulence, and more homogenous streambed topography. Therefore, despite the high potential for microbial respiration exhibited by FPM streambed sediments from this reach, we concluded that the laminar flow and limited hydrologic exchange between the water column and the hyporheic zone offset the influence of streambed FPM metabolic activity on aerobic heterotrophic respiration at the whole-reach scale.
In contrast to the slow reach, the fast reach showed higher velocity and steeper slope. In line with previous studies, our results indicate that these physical features contributed to increase the water exchange rate between the water column and transient storage areas (Boano et al., 2014). Higher water exchange rates likely foster the transfer of nutrients from the water column to metabolically active zones, thereby increasing respiration rates, and ultimately aerobic metabolic rates at the whole-reach scale. The same pattern was exhibited by the naturalized reach, for which FPM streambed sediments showed the lowest potential for microbial activity, but whole-reach metabolic rates were still higher than those for the slow reach. With higher heterogeneity of flow paths and more transient storage areas, the naturalized reach had the greatest retention time within transient storage and the greatest relative travel time. These hydrological features suggest more interaction between water column solutes and streambed FPM with biologically active areas than in the slow reach, which is supported by the higher whole-reach metabolic activity rates measured in the naturalized reach.
Our results highlight that stream hydromorphology can strongly control hydrological connectivity between free flowing water and transient storage zones, which influences the dynamics of accumulation and resuspension of streambed FPM and with that, can have a large impact on microbial activity at the habitat and reach scales. Previous studies have shown that the amount of streambed organic matter (such as FPOM) influences nutrient uptake in streams (Mulholland et al., 1985; Webster et al., 2000). In addition, Meyer et al. (2005) suggested that increasing the supply and retention of particulate organic matter in the stream should enhance nutrient uptake capacity and metabolic activity. With this in mind, the bioengineering experimental designs aimed to modify water residence times and hydrologic exchange between water column and streambed to better understand the influence of hydromorphology on overall stream functioning. The results of this study provides insights on how transient storage zones do not equally contribute in terms of metabolic activity at reach scale. Instead, the contribution of metabolic activity associated to FPM to reach-scale metabolism depends on the combined influence of hydraulic properties and quality of the FPM standing stocks. These results can have important implications within the context of stream restoration since induced hydromorphological changes can modify the stream's metabolic activity; and ultimately the in-stream capacity to attenuate organic matter and nutrient loads.
Data Availability Statement
The datasets presented in this study can be found in online repositories. The names of the repository/repositories and accession number(s) can be found below: https://doi.org/10.6084/m9.figshare.14528790.v1.
Author Contributions
JD, EM, and WM: study conception and design. WM, JD, EM, MT, and MR: data collection. WM, JD, EM, and FS: analysis and interpretation of results. WM and JD: draft manuscript preparation. EM and SB: revising the manuscript critically for important intellectual content. All authors reviewed the results and approved the final version of the manuscript.
Funding
This project was supported by a Marie Curie Individual Fellowship (833702 - MICROPATH) and an NSF EAR-Postdoctoral Fellowship to JD and project MEDSOUL (CGL2014-59977-C3-2-R) from the Spanish Office for Research (MINECO).
Conflict of Interest
The authors declare that the research was conducted in the absence of any commercial or financial relationships that could be construed as a potential conflict of interest.
Acknowledgments
We thank Thalia Lazo Campos for her help with the lab analysis.
Supplementary Material
The Supplementary Material for this article can be found online at: https://www.frontiersin.org/articles/10.3389/frwa.2021.682905/full#supplementary-material
References
Allan, J. D., and Castillo, M. (2007). Stream Ecology: Structure and Function of Running Waters. Dordrecht: Springer.
Baranov, V., Lewandowski, J., Romeijn, P., Singer, G., and Krause, S. (2016). Effects of bioirrigation of non-biting midges (Diptera: Chironomidae) on lake sediment respiration. Sci. Rep. 6:27329. doi: 10.1038/srep27329
Bauer, J., Cai, W., Raymond, P., Bianchi, T. S., Hopkinson, C. S., and Regnier, P. A. (2013). The changing carbon cycle of the coastal ocean. Nature 504, 61–70. doi: 10.1038/nature12857
Bencala, K., and Walters, R. (1983). Simulation of solute transport in a mountain pool-and-riffle stream: a transient storage model. Water. Resour. Res. 19, 718–724. doi: 10.1029/WR019i003p00718
Bernal, S., Drummond, J., Castelar, S., Gacia, E., Ribot, M., and Martí, E. (2020). Wastewater treatment plant effluent inputs induce large biogeochemical changes during low flows in an intermittent stream but small changes in day-night patterns. Sci. Tot. Environ. 714:136733. doi: 10.1016/j.scitotenv.2020.136733
Boano, F., Harvey, J. W., Marion, A., Packman, A. I., Revelli, R., Ridolfi, L., et al. (2014). Hyporheic flow and transport processes: mechanisms, models and biogeochemical implications. Rev. Geophys. 52, 603–679. doi: 10.1002/2012RG000417
Bott, T. L., and Kaplan, L. A. (1985). Bacterial biomass, metabolic state, and activity in stream sediments: relations to environmental variables and multiple assay comparisons. Appl. Environ. Microbiol. 50, 508–522. doi: 10.1128/AEM.50.2.508-522.1985
Boulton, A., Thibault, D., Kasahara, T., Mutz, M., and Stanford, J. (2010). Ecology and management of the hyporheic zone: Stream-groundwater interactions of running waters and their floodplains. J. North Am. Benthol. Soc. 29, 26–40. doi: 10.1899/08-017.1
Brugger, A., Wett, B., Kolar, I., Reitner, B., and Herndl, G. J. (2001). Immobilization and bacterial utilization of dissolved organic carbon entering the riparian zone of the alpine Enns River, Austria. Aquat. Microb. Ecol. 24, 129–142. doi: 10.3354/ame024129
Brunke, M., and Gonser, T. (1997). The ecological significance of exchange processes between rivers and groundwater. Freshw. Biol. 37, 1–33. doi: 10.1046/j.1365-2427.1997.00143.x
Bundschuh, M., and McKie, B. G. (2015). An ecological and ecotoxicological perspective on fine particulate organic matter in streams. Freshw. Biol. 61, 2063–2074. doi: 10.1111/fwb.12608
Crenshaw, C. L., Valett, H. M., and Webster, J. R. (2002). Effects of augmentation of coarse particulate organic matter on metabolism and nutrient retention in hyporheic sediments. Freshw. Biol. 47, 1820–1831. doi: 10.1046/j.1365-2427.2002.00928.x
Drummond, J., Schmadel, N., Kelleher, C., Packman, A., and Ward, A. (2019). Improving predictions of fine particle immobilization in streams. Geophys. Res. Lett. 46, 1–9. doi: 10.1029/2019GL085849
Drummond, J. D., Aubeneau, A. F., and Packman, A. I. (2014a). Stochastic modeling of fine particulate organic carbon dynamics in rivers. Water Resour. Res. 50, 4341–4356. doi: 10.1002/2013WR014665
Drummond, J. D., Davies-Colley, R. J., Stott, R., Sukias, J. P., Nagels, J. W., Sharp, A., et al. (2014b). Retention and remobilization dynamics of fine particles and microorganisms in pastoral streams. Water Res. 66, 459–472. doi: 10.1016/j.watres.2014.08.025
Drummond, J. D., Larsen, L., Gonzalez-Pinzon, G. R., Packman, A. I., and Harvey, J. W. (2017). Fine particle retention within stream storage areas at base flow and in response to a storm event. Water Resour. Res. 53:2016WR020202. doi: 10.1002/2016WR020202
Ensign, S. H., and Doyle, M. W. (2005). In-channel transient storage and associated nutrient retention: evidence from experimental manipulations. Limnol. Oceanograp. 6:1740. doi: 10.4319/lo.2005.50.6.1740
Fellows, C. S., Valett, H. M., Dahm, C. N., Mulholland, P., and Thomas, S. (2006). Coupling nutrient uptake and energy flow in headwater streams. Ecosystems 9, 788–804. doi: 10.1007/s10021-006-0005-5
González-Pinzón, R., and Haggerty, R. (2013). An efficient method to estimate processing rates in streams. Water Resour. Res. 49, 6096–6099. doi: 10.1002/wrcr.20446
González-Pinzón, R., Haggerty, R., and Myrold, D. D. (2012). Measuring aerobic respiration in stream ecosystems using the resazurin-resorufin system. J. Geophys. Res. 117:G00N06. doi: 10.1029/2012JG001965
Gordon, N. D., McMahon, T. A., Finlayson, B. L., Gippel, C. J., and Nathan, R. J. (2004). Stream Hydrology: An Introduction for Ecologists, 2nd Edn. London: Wiley.
Gottselig, N., Bol, R., Nischwitz, V., Vereecken, H., Amelung, W., and Klumpp, E. (2014). Distribution of phosphorus-containing fine colloids and nanoparticles in stream water of a forest catchment. Vadose Zone J. 13, 1–11. doi: 10.2136/vzj2014.01.0005
Grimm, N. B., and Fisher, S. G. (1984). Exchange between interstitial and surface water: implications for stream metabolism and nutrient cycling. Hydrobiologia 111, 219–228. doi: 10.1007/BF00007202
Grossart, H. P., and Ploug, H. (2001). Microbial degradation of organic carbon and nitrogen on diatom aggregates. Limnol. Oceanograph. 2:267. doi: 10.4319/lo.2001.46.2.0267
Haggerty, R., Argerich, A., and Marti, E. (2008). Development of a “smart” tracer for the assessment of microbiological activity and sediment-water interaction in natural waters: the resazurin-resorufin system. Water Resour. Res. 44:W00D01. doi: 10.1029/2007WR006670
Haggerty, R., Marti, E., Argerich, A., von Schiller, D., and Grimm, N. B. (2009). Resazurin as a “smart” tracer for quantifying metabolically active transient storage in stream ecosystems. J. Geophys. Res. 114:G03014. doi: 10.1029/2008JG000942
Haggerty, R., Ribot, M., Singer, G. A., Martí, E., Argerich, A., Agell, G., et al. (2014). Ecosystem respiration increases with biofilm growth and bed forms: flume measurements with resazurin. J. Geophys. Res. Biogeosci. 119, 2220–2230. doi: 10.1002/2013JG002498
Harvey, J. W., Böhlke, J. K., Voytek, M. A., Scott, D., and Tobias, C. R. (2013). Hyporheic zone denitrification: controls on effective reaction depth and contribution to whole-stream mass balance. Water Resour. Res. 49, 6298–6316. doi: 10.1002/wrcr.20492
Harvey, J. W., and Fuller, C. F. (1998). Effect of enhanced manganese oxi- dation in the hyporheic zone on basin-scale geochemical mass balance. Water Resour. Res. 34, 623–636. doi: 10.1029/97WR03606
Hope, D., Billett, M. F., and Cresser, M. S. (1994). A review of the export of carbon in river water: fluxes and processes. Environ. Pollut. 84, 301–324. doi: 10.1016/0269-7491(94)90142-2
Jones, J. B., and Holmes, R. M. (1996). Surface-subsurface interactions in stream ecosystems. Trends Ecol. Evol. 11, 239–242. doi: 10.1016/0169-5347(96)10013-6
Karakashev, D., Galabova, D., and Simeonov, I. (2003). A simplet and rapid test for differentiation of aerobic from anaerobic bacteria. World J. Microbiol. Biotechnol. 19, 233–238. doi: 10.1023/A:1023674315047
Kelleher, C., Ward, A., Knapp, J. L. A., Blaen, P. J., Kurz, M. J., Drummond, J. D., et al. (2019). Exploring tracer information and model framework trade-offs to improve estimation of stream transient storage processes. Water Resour. Res. 55, 3481–3501. doi: 10.1029/2018WR023585
Kelso, J. E., and Baker, M. A. (2020). Organic matter is a mixture of terrestrial, autochthonous, and wastewater effluent in an urban river. Front. Environ. Sci. 7:202. doi: 10.3389/fenvs.2019.00202
Knapp, J. L. A., González-Pinzón, R., Drummond, J. D., Larsen, L. G., Cirpka, O. A., and Harvey, J. W. (2017). Tracer-based characterization of hyporheic exchange and benthic biolayers in streams. Water Resour. Res. 53:2016WR019393. doi: 10.1002/2016WR019393
Kondratieff, P. F., and Simons, G. M. (1985). Microbial colonization of seston and free bacteria in an impounded river. Hydrobiologia 128, 127–133 doi: 10.1007/BF00008732
Kurz, M. J., Drummond, J. D., Martí, E., Zarnetske, J. P., Lee-Cullin, J., Klaar, M. J., et al. (2017). Impacts of water level on metabolism and transient storage in vegetated lowland rivers: insights from a mesocosm study. J. Geophys. Res. Biogeosci. 122, 628–644. doi: 10.1002/2016JG003695
Lautz, L. K., and Fanelli, R. M. (2008). Seasonal biogeochemical hotspots in the streambed around restoration structures. Biogeochemistry 91, 85–104. doi: 10.1007/s10533-008-9235-2
Lemly, A. D., and Hilderbrand, R. H. (2000). Influence of large woody debris on stream insect communities and benthic detritus. Hydrobiologia 421, 179–185. doi: 10.1023/A:1003904130002
Marti, E., Aumatell, J., Godé, L., Poch, M., and Sabater, F. (2004). Nutrient retention efficiency in streams receiving inputs from wastewater treatment plants. J. Environ. Qual. 33:285. doi: 10.2134/jeq2004.2850
Mendoza-Lera, C., and Datry, T. (2017). Relating hydraulic conductivity and hyporheic zone biogeochemical processing to conserve and restore river ecosystem services. Sci. Tot. Environ. 579, 1815–1821. doi: 10.1016/j.scitotenv.2016.11.166
Mendoza-Lera, C., Frossard, A., Knie, M., Federlein, L. L., Gessner, M. O., and Mutz, M. (2017). Importance of advective mass transfer and sediment surface area for streambed microbial communities. Freshw. Biol. 62, 133–145. doi: 10.1111/fwb.12856
Merbt, S. N., Auguet, J.-C., Blesa, A., Martí, E., and Casamayor, E. O. (2014). Wastewater treatment plant effluents change abundance and composition of ammonia-oxidizing microorganisms in mediterranean urban stream biofilms. Microb. Ecol. 69, 66–74. doi: 10.1007/s00248-014-0464-8
Meyer, J. L., Paul, M. J., and Taulbee, W. K. (2005). Stream ecosystem function in urbanizing landscapes. J. North Am. Benthol. Soc. 24:602612. doi: 10.1899/04-021.1
Mulholland, P. J., Newbold, J. D., Elwood, J. W., Ferrin, L. A., and Webster, J. R. (1985). Phosphorus spiralling in a woodland stream: seasonal variations. Ecology 66, 1012–1023. doi: 10.2307/1940562
Nogaro, G., Mermillod-Blondin, F., Montuelle, B., Boisson, J., Bedell, J., Ohannessian, A., et al. (2007). Influence of a stormwater sediment deposit on microbial and biogeochemical processes in infiltration porous media. Sci. Tot. Environ. 377, 334–48. doi: 10.1016/j.scitotenv.2007.01.093
Peipoch, M., Gacia, E., Bastias, E., Serra, A., Proia, L., Ribot, M., et al. (2016). Small-scale heterogeneity of microbial N uptake in streams and its implications at the ecosystem level. Ecology 97, 1329–1344. doi: 10.1890/15-1210.1
Petticrew, E. L., Krein, A., and Walling, D. E. (2007). Evaluating fine sediment mobilization and storage in a gravel-bed river using controlled reservoir releases. Hydrol. Process. 21, 198–210. doi: 10.1002/hyp.6183
Stelzer, R. S., Heffernan, J., and Likens, G. E. (2003). The influence of dissolved nutrients and particulate organic matter quality on microbial respiration and biomass in a forest stream. Freshw. Biol. 48, 1925–1937. doi: 10.1046/j.1365-2427.2003.01141.x
Stutter, M. I., Langan, S. J., and Demars, B. O. (2007). River sediments provide a link between catchment pressures and ecological status in a mixed land use Scottish River system. Water Res. 41, 2803–2815. doi: 10.1016/j.watres.2007.03.006
Tank, J. L., Rosi-Marshall, E. J., Griffiths, N. A., Entrekin, S. A., and Stephen, M. L. (2010). A review of allochthonous organic matter dynamics and metabolism in streams. J. North Am. Benthol. Soc. 29, 118–146. doi: 10.1899/08-170.1
Tant, C. J., Rosemond, A. D., and First, M. R. (2013). Stream nutrient enrichment has a greater effect on coarse than on fine benthic organic matter. Freshw. Sci. 32, 1111–1121. doi: 10.1899/12-049.1
Wang, Y., Hu, Y., Yang, C., Wang, Q., and Jiang, D. (2018). Variations of DOM quantity and compositions along WWTPs-river-lake continuum: implications for watershed environmental management. Chemosphere 218, 468–476. doi: 10.1016/j.chemosphere.2018.11.037
Ward, A. S. (2016). The evolution and state of interdisciplinary hyporheic research. Water 3, 83–103. doi: 10.1002/wat2.1120
Webster, J. R., and Meyer, J. L. (1997). Organic matter budgets for streams: a synthesis. J. North Am. Benthol. Soc. 16, 141–161. doi: 10.2307/1468247
Webster, J. R., Newbold, J. D., Thomas, S. A., Valett, H. M., and Mulholland, P. J. (2009). Nutrient uptake and mineralization during leaf decay in streams – a model simulation. Int. Rev. Hydrobiol. 94, 372–390. doi: 10.1002/iroh.200811158
Webster, J. R., Tank, J. L., Wallace, J. B., Meyer, J. L., Eggert, S. L., Ehrman, T. P, et al. (2000). Effects of litter exclusion and wood removal on phosphorus and nitrogen retention in a forest stream. Verhandlungen der Internationalen Vereinigung fur theoretische und angewandte Limnologie 27, 1337–1340. doi: 10.1080/03680770.1998.11901453
Keywords: fine particle standing stocks, aerobic respiration, streambed, hydromorphology, stream metabolism, organic matter, hydrologic exchange, catchment
Citation: Meredith W, Drummond J, Bernal S, Tobella M, Ribot M, Schumer R, Sabater F and Martí E (2021) Hydromorphologic Control of Streambed Fine Particle Standing Stocks Influences In-stream Aerobic Respiration. Front. Water 3:682905. doi: 10.3389/frwa.2021.682905
Received: 19 March 2021; Accepted: 20 May 2021;
Published: 21 June 2021.
Edited by:
Dipankar Dwivedi, Lawrence Berkeley National Laboratory, United StatesReviewed by:
Asghar Azizian, Imam Khomeini International University, IranDavid W. P. Manning, University of Nebraska at Omaha, United States
Copyright © 2021 Meredith, Drummond, Bernal, Tobella, Ribot, Schumer, Sabater and Martí. This is an open-access article distributed under the terms of the Creative Commons Attribution License (CC BY). The use, distribution or reproduction in other forums is permitted, provided the original author(s) and the copyright owner(s) are credited and that the original publication in this journal is cited, in accordance with accepted academic practice. No use, distribution or reproduction is permitted which does not comply with these terms.
*Correspondence: Warren Meredith, d2FycmVuLm1lcmVkaXRoJiN4MDAwNDA7dWRnLmVkdQ==; Jennifer Drummond, ai5kcnVtbW9uZCYjeDAwMDQwO2JoYW0uYWMudWs=
†These authors have contributed equally to this work and share first authorship