- 1Centre for Hydrogeology and Geothermics (CHYN), University of Neuchâtel, Neuchâtel, Switzerland
- 2Swiss Seismological Service (SED), Swiss Federal Institute of Technology Zürich, Zürich, Switzerland
- 3Department of Environmental Sciences, University of Basel, Basel, Switzerland
- 4Institute of Geological Sciences and Oeschger Centre for Climate Change Research, University of Bern, Bern, Switzerland
Pockmarks are crater-like depressions formed by upward fluid flow (gas and/or liquid) through the unconsolidated sediment column on the floor of oceans and lakes. While pockmarks are well described in the marine realm, they have essentially been overlooked in lacustrine settings, likely due to a lack in economic interest to apply high-resolution hydroacoustic techniques in lakes. A swath-bathymetry survey on Lake Thun, Switzerland, revealed the existence of three pockmark systems. One pockmark (110 m in diameter) was discovered near a big karst system at Beatenberg at a water depth of ~217 m. Its activity is probably associated with episodic groundwater seepage induced by earthquakes, floods and snowmelt. At another site, Daerligen, we detected at ~60 m water depth the presence of multiple smaller pockmarks (~1.5 to 10 m in diameter) that seem to be active, continuously liberating CH4 gas by bubble ebullition. The CH4 displayed a biogenic carbon isotopic signature, however, the exact origin of the gas remains unknown. The third site, Tannmoos (~35 m water depth), comprises two large pockmarks (20–43 m in diameter) connected to a karst system in gypsum-carrying bedrock. One of these pockmarks is constituted of several unit pockmarks (e.g., sub-pockmarks; 0.3 to 0.8 m in diameter). While strong evidence is still lacking, we suggest that groundwater discharge occasionally occurs through these unit pockmarks during periods of intense precipitation. Hence, this study reveals the existence of three pockmark systems of variable morphology and mechanisms of formation within the same lake, reflecting different hydrological and biogeochemical regimes. Moreover, it underscores the potential importance of pockmarks in influencing hydrological and CH4 budgets in lakes. Clearly more work on quantifying seasonal fluxes of groundwater and CH4 release via lacustrine pockmarks is required, and it needs to be seen whether the observations made in Lake Thun are universal and apply also to many other lacustrine environments worldwide.
Introduction
The discovery of pockmarks, i.e. circular or ellipsoidal depressions on the sea floor, in the late 1960s off the coast of Nova Scotia has marked the beginning of a new research era for marine scientists (King and MacLean, 1970). Since then, different types of pockmarks have been observed worldwide in different geological settings, such as the deep sea, coastal oceans, fjords, and lakes (Hovland and Judd, 1988; Judd and Hovland, 2007, Wessels et al., 2010; Wirth et al., 2020). Pockmarks are crater-like depressions that naturally form on the seabed and the bottom of lakes by upward fluid migration of gas and/or liquid through the unconsolidated sediment column (King and MacLean, 1970; Hovland and Judd, 1988). When active, pockmarks can induce significant fluid seepage to the hydrosphere, which may strongly affect the surrounding ecosystem (Bussmann et al., 2011; Virtasalo et al., 2019; Hoffmann et al., 2020). It has previously been shown, for instance, that submarine groundwater discharge via pockmarks can constitute an important source of nutrients, or of harmful substances, to the ocean (Moore, 2010). Also, fluid flow at pockmarks may liberate significant amounts of CH4 to the hydrosphere that contributes to global warming (Bussmann and Suess, 1998), or it may lead to fatalities due to the formation of pits and craters near-shore (Hovland et al., 2002). Hence, pockmarks may play an important role in modulating environmental conditions on multiple spatial scales. However, their worldwide distribution, morphology, mechanisms of formation, and present-day activity are still not well constrained, and this is particularly true for the lacustrine realm.
In marine environments, pockmarks have been detected worldwide at the margin of salt domes, on continental slopes, and along fault zones (Hovland and Judd, 1988; Cathles et al., 2010; Roy et al., 2014; Roelofse et al., 2020). Yet, understanding their exact mechanism of formation remained a challenge for scientists for a long time. Cathles et al. (2010) proposed that a capillary seal can act to retain gas below fine-grained sediment layers, where CH4 gas accumulates until its pressure becomes sufficiently high to invade and destroy the seal. A large fraction of the accumulated gas is thus released into an upward-propagating gas chimney, which liquefies the surface sediments through the displacement of water while rising. The liquefied sediments are then transported away by bottom-water currents, forming pockmarks. More recently, Räss et al. (2018) demonstrated that chimney formation of pockmarks is mainly due to viscous creep of the fluid-rich porous matrix, decompaction weakening, and flow of the pore fluid. After formation, pockmarks are likely maintained by slow and/or occasional pore-water and gas seepage (Cathles et al., 2010). Yet, curiously, most of the pockmarks investigated so far in the marine realm have been dormant or inactive for years or even decades. Despite their apparent inactivity (i.e., no evidence for recent fluid expulsion), pockmarks did not fill with sediments over time. Several scenarios have been suggested: (i) fine sediments are kept in suspension by turbulence due to bottom currents induced by the pockmark structure and are transported away before settling (Pau and Hammer, 2013); (ii) sediment-laden seafloor currents are deflected by the pockmarks preventing the settling of suspended fine particles (Hammer et al., 2009); (iii) or the activity of fishes leads to constant bioerosion inside pockmarks and thus to reduced sedimentation rates (Pau and Hammer, 2013). Yet, it is also possible that pockmarks are episodically active, but their active phases remained unnoticed.
Hydroacoustic surveying techniques have first been applied in the ocean for petroleum exploration. As lakes are of minor interest in this regard, high-resolution bathymetric maps are produced solely out of research interest, with significantly less financial support. This delayed the discovery of pockmarks in the lacustrine realm. The presence of pockmarks in lakes was for the first time highlighted by Pickrill (1993) in Lake Rotoiti, New Zealand. Using high-resolution seismic reflection profiles, the study showed that numerous pockmarks were located in areas of sediments with a high gas content (Pickrill, 1993). Similarly, the presence of hundreds of pockmarks, formed through CH4 ebullition, has been revealed in Lake Constance, Germany (Wessels et al., 2010; Bussmann et al., 2011), where 24 % of them were persistently active (Bussmann et al., 2011). More recently, Reusch et al. (2015) discovered four large (i.e., diameters of 80 to 160 m) pockmark systems in Lake Neuchatel, Switzerland. Given the vicinity and proven connectivity of Lake Neuchatel to the Jura karst system, the pockmark activity was proposed to be mainly driven by groundwater discharge rather than CH4 gas ebullition (Wirth et al., 2020).
In Lake Thun, three pockmark systems were detected based on new swath-bathymetric data. One pockmark was discovered in the deepest part of the lake and located adjacent to a large karst system, the Beatenberg karst system (Häuselmann, 2002; Figure 1). At Daerligen, several smaller pockmarks (~1.5 to 10 m in diameter) were detected near the mouth of the Aare river. Finally, a third pockmark system connected to a sedimentary bedrock with gypsum at Tannmoos was recently presented in the context of a newly detected fault zone by Fabbri et al. (2017). However, no further investigations on these pockmarks were realized. Here, using a multidisciplinary approach combining geophysical, sedimentological, geological, and biogeochemical data, we (1) describe further the characteristics and morphology of these different pockmark systems, (2) elucidate the potential mechanisms of formation, and (3) investigate their past and present-day activity. The overarching goal of the presented study was to provide a better understanding of the functioning of lacustrine pockmarks, and to assess their potential role in influencing hydrological and biogeochemical budgets of the lake.
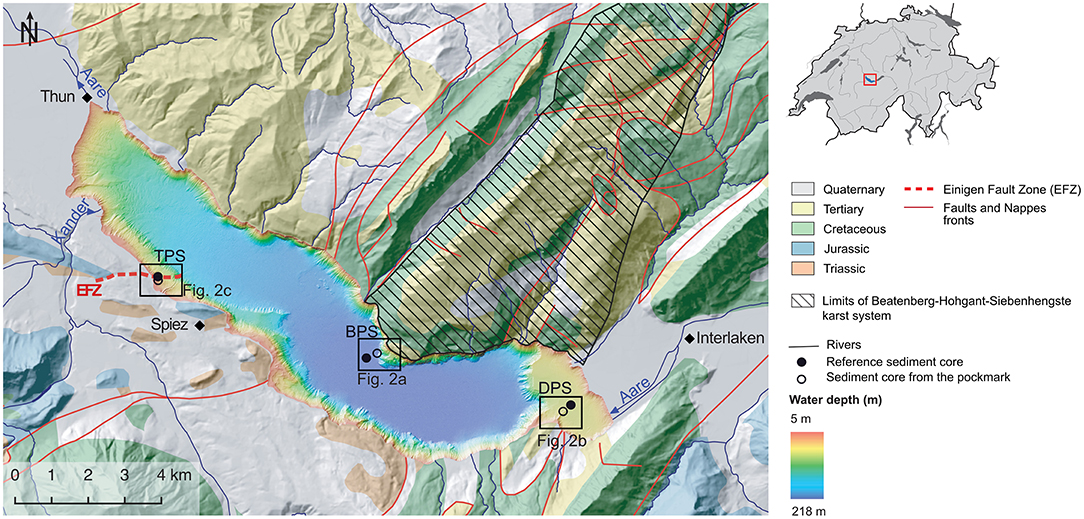
Figure 1. Bathymetric map of Lake Thun, Switzerland. Also indicated is the geology of the area [Swisstopo (2014) Vector dataset], the area of the Beatenberg-Hohgant-Siebenhengste karst system (Géotope Suisse n° 127), the location of the Einigen Fault Zone (EFZ; Fabbri et al., 2017), and the different sampling sites, Beatenberg (BPS; a), Daerligen (DPS; b), and Tannmoos (TPS; c). The sampling positions at the reference and pockmark sites are represented by the black circles and dots, respectively.
Study Area
Lake Thun (48.3 km2 surface area) is a peri-alpine oligo-mesotrophic lake with a maximum depth of 216.5 m situated in the upper Aare Valley between the cities of Interlaken and Thun, Switzerland (Figure 1). During past glaciations, subglacial meltwater erosion of the Aare glacier led to glacial overdeepening, shaping the bedrock surface of the valley (Häuselmann, 2002; Reber and Schlunegger, 2016; Fabbri et al., 2018). The late-glacial sedimentary infill (~175 ± 10 m; Fabbri et al., 2018) of the Aare Valley may have been formed in less than 1000 years (from ~17.7 ± 0.8 to 16.5 ± 1.4 ka; Fabbri et al., 2018), which was likely due to a rapid disintegration of the Aare glacier, accompanied by extremely high sedimentation rates (Ravazzi et al., 2014; Fabbri et al., 2018). The Holocene sedimentary infill is about 40 m thick in the shallower part, at the end of the lake, and 60 m in the deep basin (Fabbri et al., 2018). More recently, human activities have strongly affected the lake system through river deviations and excessive nutrient input (Rellstab et al., 2011; Wirth et al., 2011). Nowadays, the Kander and Aare rivers, the inflows of which are located close to Einigen and Interlaken, respectively, supply ~80% of the inflowing water to the lake. In contrast, the Kander River alone is responsible for 85 % of the total sediment supply, while the Aare River deposits most of its sediment load already in upstream Lake Brienz (Wirth et al., 2011; Figure 1).
Lake Thun is tectonically located at the North Alpine Front (e.g., Strasser et al., 2013). The geology of the northwestern shore of the lake is dominated by Subalpine Molasse, while the rest of the lake is surrounded by sedimentary Penninic and Helvetic tectonic units. Particularly important with regards to the Beatenberg pockmark system (BPS) is a substantial karst system, comprising extensive caves and phreatic tubes within the limestones of the Wildhorn nappe located at the northeastern shore of the lake. This system is known as the Beatenberg karst system (Häuselmann, 2002; Wirth, 2008). Dye tracer experiments and diving surveys in the past, led to the discovery of lacustrine groundwater discharge via subaquatic springs in Lake Thun. The well-known “Bätterich” spring is located ~4 km east of the BPS (Häuselmann, 2002). The geology at the location of the Daerligen pockmark system (DPS) is characterized by the Wildhorn nappe (limestones, sandstones), as well as by Ultrahelvetic Flysch (Swiss Federal Office of Topography, swisstopo, Wabern, Switzerland). At the Tannmoos pockmark system (TPS), located at the southwestern shore, the Klippen nappe, containing Triassic gypsum-bearing limestones, represents the most prominent near-lake geologic feature. Fabbri et al. (2017) highlighted the presence of a potentially active fault zone in the gypsum bedrock near Tannmoos (Einigen Fault Zone, Figure 1), along which subaquatic gas and water seeps, as well as pockmarks were observed.
Materials and Methods
High-resolution multibeam bathymetry data of Lake Thun were obtained as described in Fabbri et al. (2017), and these data were adopted here. Further surveys of the lake floor were performed using a remotely operated underwater vehicle (ROV, RB600, Eprons) in March 2017, October 2019, and March 2020. The ROV was equipped with a camera and a 360° sonar (Tritech Seaprince) that allowed the investigation of pockmarks and the detection of potential gas seeps. In fact, the 360° sonar allowed detecting structures at greater detail than what was previously achieved by the bathymetric data. Moreover, for the survey at the Tannmoos pockmark, a conductivity-temperature-depth (CTD; DCX-22-CTD, Keller, Switzerland) probe was mounted on the ROV.
Sediment cores were collected at all three pockmark systems, BPS, DPS, and TPS, and their respective reference location (Figure 1). One long Kullenberg-type sediment core (maximum length ~10 m) was collected at BPS and DPS in May 2018, while four short cores (0.5 to 1 m long) were sampled with a gravity corer or by a coring procedure supported by diving techniques at TPS in March and May 2020. Samples for analyzing the concentration and carbon isotopic composition (δ13C) of methane (CH4) dissolved in sediment porewater at DPS were subsampled directly onboard. 3 mL cut-off syringes were pushed into the sediments through pre-drilled holes (12 mm in diameter) in the PVC tubes. For headspace analysis, aliquots of 2.5 mL sediments were subsampled into 30 mL glass flasks prefilled with 4 mL NaOH (2.5 % w/v), which were then immediately closed with thick butyl rubber stoppers (Niemann et al., 2015). In a second core, porewater was extracted using Rhizons samplers (Rhizosphere Research Product) that were directly inserted after core retrieval through pre-drilled holes (3 mm in diameter) and connected to evacuated tubes via a needle. Samples were kept frozen until analysis. Additional intact short cores were stored in a cold room (4°C) for sedimentological analyses.
Dissolved CH4 concentration was analyzed in the headspace of the 30 mL flasks with a gas chromatograph with a flame ionization detector (GC-FID, Agilent 6890N) and helium as carrier gas. The concentrations in the wet sediment samples were then calculated based on the sediment-volume to headspace ratio. The carbon (C) isotopic composition of CH4 from the headspace was determined using a preconcentration unit (Precon, Finnigan) connected to an isotope ratio mass spectrometer (IRMS; Delty XL, Finnigan). Stable C-isotope values are reported in the conventional δ notation (in ‰) relative to the Vienna Pee Dee Belemnite standard (V-PDB) with an analytical error of ± 1‰. Concentrations of cations (Fe2+, Mn2+, Na+, K+, Ca2+, Mg2+, Sr2+) and anion (Cl−, Br−, F−, , , ) were determined by inductively coupled plasma-optical emission spectroscopy (ICP-OES; Perkin-Elmer Optima 2100) and ion chromatography (IC, Dionex ICS-1600), respectively.
The density and the magnetic susceptibility (MS) of the sediment material were logged at a 0.5-cm down-core resolution on unsplit cores using a Geotek multi-sensor core logger (MSCL) located at the Institute of Geological Sciences, Bern, Switzerland. Immediately after core opening, the fresh sediment surface was photographed using the Line Scan Camera system of an XRF core scanner at Eawag (Avaatech). 137Cs dates were adopted from Wirth et al. (2011).
Results and Discussion
In the following, we will present and discuss the results for each of the pockmark systems individually, documenting the different morphologies and highlighting the differential formation mechanisms and past/present activities.
Beatenberg Pockmark System BPS—A Giant Pockmark Activated by Episodic Groundwater Discharge
Results—The Beatenberg pockmark (110 m in diameter) is located adjacent to the bedrock wall of the Beatenberg karst system in the deepest part of the lake (216.5 m water depth; Figure 1). Two Kullenberg cores of 6 and 10 m length were taken inside the pockmark (THU18-Ku4) and at a reference site outside (THU18-Ku8), respectively (Figure 2). The reference site is located 325 m southwest of the center of the pockmark.
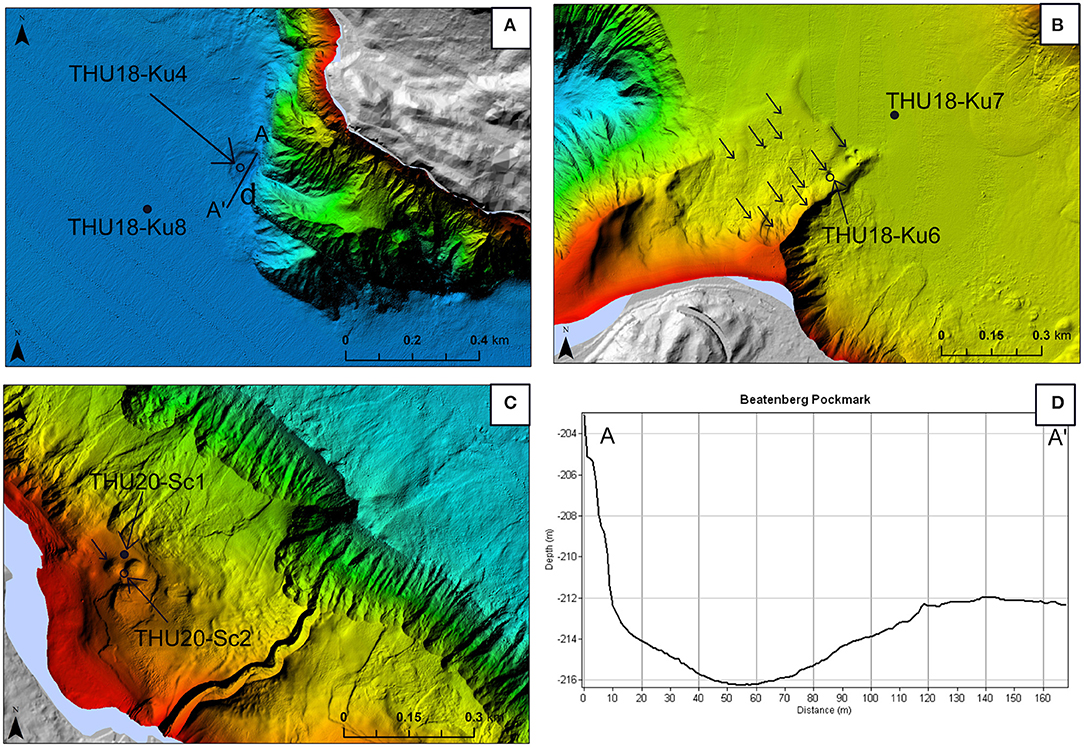
Figure 2. Zoom-in of the bathymetric map (Figure 1) showing the locations of the pockmark and reference cores sampled at BPS (A), DPS (B), and TPS (C). (D) Shows a profile (A-A') through the Beatenberg pockmarks, representing the deepest point of the lake. The arrows show the different pockmarks present on the lake bed at the three sites. The sampling positions at the reference and pockmark sites are represented by the black circles and dots, respectively.
The sediments retrieved from the reference core were in general composed of light grayish to yellowish/light brownish mud interpreted as background sedimentation, intercalated by (1) cm-thick gray silty to muddy layers with a fining upwards grainsize that are interpreted as flood deposits and (2) mass movement-related turbidites identified following Wirth et al. (2011; Figure 3). The sediments retrieved from the pockmark core showed a similar sediment pattern than in the reference core and a correlation was possible between reference and pockmark core for the upper first meter. However, in the pockmark core, three clayey layers of gray-blueish color were identified and interpreted as overflow deposits. The top and bottom of the first two overflow deposits were gradual and no sharp limit was visible. The third overflow deposit (~10 cm thick; Figure 3) showed a turbidite stratum of ~5 cm at its base while the top was composed of a sharp silty sediment layer. The top of the overflow deposit highlighted a mm thin gray clay layer. From one meter sediment depth downwards, the sediments of the pockmark core were deformed, and no layering or stratification was visible. The sediments were mostly chaotic with disrupted laminations and individual downward (e.g., fluidization; Frey et al., 2009) warped laminae (e.g., 530 m depth, Figure 3). The MS was quite constant (4.9 ± 0.6 SI x 10−5) in the surface sediments (0 to 84 cm depth) at the reference site, while, inside the pockmark, the MS locally increased from 6.7 ± 0.6 SI x 10−5 (average of MS baseline) to 25.9, 11.6, and 17.5 SI × 10−5 at ~61, 26, and 13 cm core depth, respectively (Figure 3). These MS peaks corresponded to three clayey layers of gray color, which did not exist at the reference site. Based on 137Cs dating of the sediments, as well as on the flood-layer chronology (Wirth et al., 2011), estimated ages of these deposits are 1898, 1963, and 1999 (Figure 3).
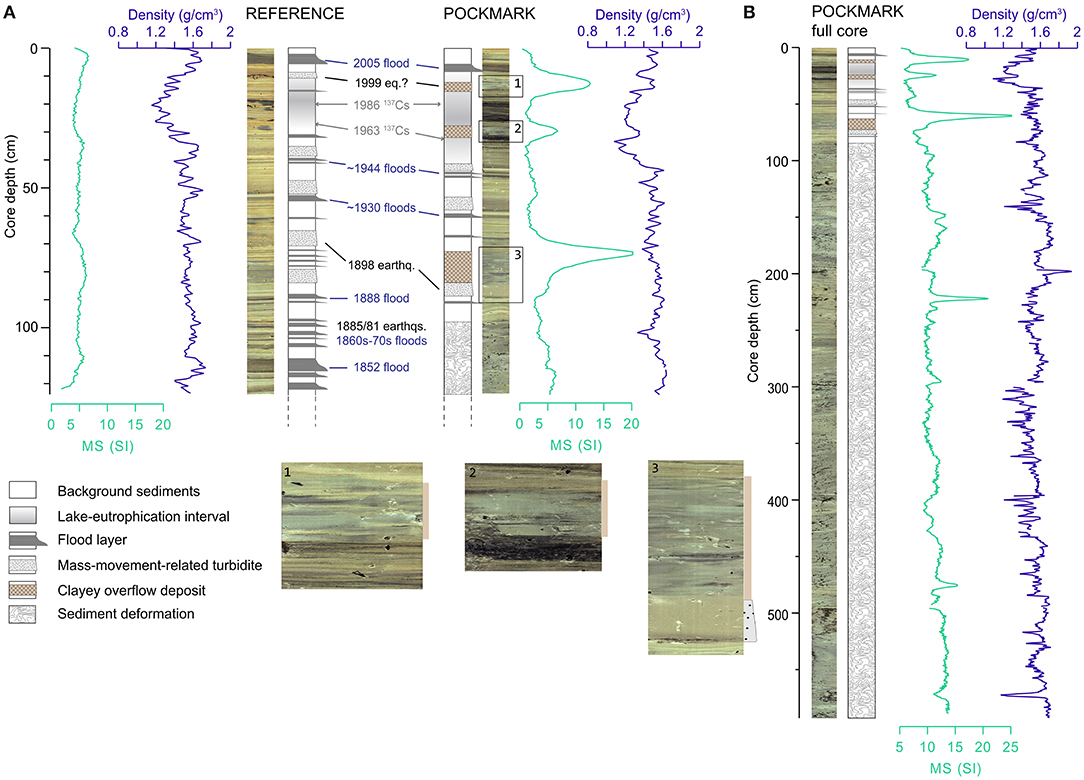
Figure 3. Sediment description of the pockmark and reference core of the BPS including photographs and lithology correlated with the magnetic susceptibility (MS) and density of (A) the uppermost 120 cm of the reference and pockmark cores, and (B) the full Kullenberg core taken inside the pockmark. 137Cs dating and the flood-layer chronology in (A) is based on Wirth et al. (2011).
The porewater biogeochemistry was quite similar at both sites. The concentration of cations and anions was quite constant all along the cores, except for (Supplementary Figure 1). Indeed, at the reference site, the vertical concentration profile showed peaks up to 8 mg L−1 at several sediment depths, while such feature was not observed at the pockmark site. However, a potential O2 contamination leading to the reoxidation of H2S to due to the method used (Rhizons) cannot be rules out, and further investigation is required to draw conclusion about the activity of the biogeochemical S cycle in the sediments.
Upward transport of liquefied late-glacial clay sediments—The intercalated clayey sediments (gray color, higher MS, smeared structure) that were observed at distinct depths in the BPS core were not found in the reference core (i.e., maximal long-core length of 10 m), nor in previously investigated sediments from Lake Thun (Rellstab et al., 2011; Wirth et al., 2011). It is thus likely that these clayey sediments occur only very localized and originate from greater sediment depths that were not reached by the Kullenberg coring. The observed clayey layers are typical for late-glacial clay, as also observed in other lake basins in northern Switzerland. Fabbri et al. (2018) reported that, based on the interpretation of airgun reflection seismic profiles, such late-glacial clays are indeed present in Lake Thun below 75 m sediment depth. This in turn suggests that the clayey sediments found in much shallower depth at the pockmark site may have been transported upward through the pockmark sediment column during times with upward migration of fluid in the past.
Lake Thun is an area where earthquakes of low to moderate magnitude and important floods were recorded during the past centuries (Earthquake Catalog of Switzerland 2009, ECOS-09, Swiss Seismological Service; Fäh et al., 2011; Wirth et al., 2011). Potentially, such events may have triggered groundwater discharge through subaquatic springs. During periods of elevated hydraulic head in the form of intense groundwater discharge, the clayey sediments may have been transported upward through a vertical to subvertical channel from a spring located in the bedrock wall of the karst system. On the lake floor, these sediments were distributed within the perimeter of the pockmark, deposited as clayey overflow sediments. The fact that we see, within the first meter of sediment in the pockmark core, these three clay layers within an otherwise undisturbed sediment stratigraphy (Figure 3), suggests that the coring site does not correspond to the actual location of the channel, through which the groundwater seepage occurred. If the core location and the actual channel location today corresponded, one would expect that the sediment strata had been entirely perturbed. We suggest thus that the active channel is located at an unknown distance to our coring site within the perimeter of the pockmark.
Evidence for sublacustrine groundwater discharge from the karst system—The first clayey layer (e.g., located at 61 cm depth within the pockmark core) was likely deposited around 1898. This layer is preceded by a mass movement-related turbidite deposit layer (Figure 3), which is also recognized at the reference site at a core depth of about 77 cm. Mass movement-related turbidite layers can be generated by slope failures due to earthquake-induced shaking (Schnellmann et al., 2005; Wirth et al., 2011; Strasser et al., 2013) among other triggers (e.g., lake level fluctuation, storm; Råman Vinnå et al., 2020). The observed turbidite layers in Lake Thun are thus likely the result of an earthquake recorded in 1898, which occurred at about 20 km away from the pockmark with a maximum magnitude of 4.8 (06/05/1898, Kandersteg; Earthquake Catalog of Switzerland 2009, ECOS-09, Swiss Seismological Service). A prior study of the lake sediments has already highlighted the presence of this mass-movement turbidite layer in the deep lake basin (Wirth et al., 2011). Indeed, at some core locations studied in Wirth et al. (2011), the turbidite layer is associated with simultaneously occurring sediment micro-deformations that indicate earthquake-induced shaking. At the pockmark site, this turbidite layer is directly followed by the clayey sediment layer at ~84 cm depth (Figure 3). A relation between the 1898 earthquake and the deposition of the clayey layer is therefore highly plausible. We speculate that the earthquake triggered increased groundwater flow in the karst system via water flow acceleration in the aquifers (Gaffet et al., 2003) or reactivation of water flow from isolated low-permeability karstic pore spaces (Charmoille et al., 2005). Hence, the activation of important groundwater discharge through subaquatic springs located in the bedrock adjacent to the pockmark dragged the late-glacial sediments along through the unconsolidated sediment column and deposited them at the sediment surface. The absence of late-glacial clay sediments above the turbidite deposit layer at the reference site suggests that the morphology of the pockmark (e.g., depression with rims) prevented the overflow of clayey material all the way into the deep basin and/or that the overflow volume was simply not large enough to deposit a discernible clayey layer at the reference site (325 m away; Figure 2).
The second clayey layer (e.g., located at 26 cm depth within the pockmark core) can likely be pinpointed to the year 1963. No earthquakes were recorded in 1963 in the region (within a radius of about 40 km). However, during that year, Switzerland suffered from a particular cold winter (January-February), with monthly average temperatures between −3.9°C and −5°C, and moderately heavy snowfall (22–25 cm monthly average depth of lying snow) were recorded near Lake Thun (Interlaken, 566 m asl; Meteosuisse IDAweb database, available at http://www.meteosuisse.admin.ch/). Excessive snowfall can also be assumed for the catchment area of the Beatenberg karst system (2,063 m asl highest altitude), which may have subsequently resulted in intense groundwater discharge and activation of the pockmark upon snowmelt in spring. As no mass movement-related turbidite layer preceding the clayey sediments was observed, we exclude the occurrence of an earthquake as potential trigger mechanism.
The third clayey layer (e.g., located at 13 cm depth within the pockmark core) is most probably associated to an event in 1999. In 1999, the occurrence of an earthquake at less than 20 km away from the pockmark site (Lake Brienz) was recorded (M = 3.61, 14/05/1999; Earthquake Catalog of Switzerland 2009, ECOS-09, Swiss Seismological Service). However, in contrast to 1898, the earthquake did not result in the deposition of mass movement-related turbidites, neither at the pockmark nor at the reference site. It is thus plausible to assume that the magnitude of the earthquake was insufficient to induce a slope failure (and in turn the deposition of a mass movement-related turbidite layer) but was strong enough to trigger increased water flow in the karst system leading to sediment overflow at the pockmark site. Yet, alternative triggering mechanisms are also possible. Intense flooding occurred in May 1999 (13-14/05/1999; Hochwasser 1999, Federal Office for Water and Geology), which likely induced high groundwater discharge via subaquatic springs, and possibly activated the pockmark. Interestingly, the earthquake and the flood occurred at the very same day. It has been shown previously that excessive precipitation during rain-storm events may trigger low-magnitude earthquakes in karst systems (M = 1–2.4), by channeling large volumes of water directly into the system and inducing pore pressure changes beyond failure thresholds (Hainzl et al., 2006; Miller, 2008). However, it is unlikely that an earthquake of such magnitude (M = 3.6) was triggered through karst discharge.
We suggest that the present-day activity of the pockmark (e.g., upward transport of the sediments) is episodic. A recent study from Lake Neuchatel highlighted the presence of liquefied sediments in a lacustrine pockmark that is connected to a karst system, and is persistently active at present (Wirth et al., 2020). Here, in contrast, the sediments were not fully liquefied, and the clayey layers are observed only episodically.
Pockmark activity before 1898—While we could identify the different mechanisms potentially responsible for the Beatenberg pockmark activity until 1898, we have less constraints on the pockmark activity in the earlier past. Indeed, in contrast to the reference site, we observed a continuous sediment deformation in the deeper layers in the pockmark core (~100 cm to the core bottom at 593 cm depth; Figure 3). Originally, the deeper sediment column was probably as well stratified as at the reference site, but upward migration of karst groundwater through the sediment column, may have disturbed the sediment stratification. The difference in sediment properties between the upper part (<1 m; intact stratification, three clayey layers) and the lower part of the core implies that the pockmark displayed a greater activity in the past due to hydrologically or seismically enhanced groundwater discharge in the karst system. Indeed, intense flooding occurred between 1865 and 1890 in the area of Lake Thun, concurrently with the end of the Little Ice Age in the Swiss Alps (Wirth et al., 2011; Painter et al., 2013). The end of this period correlates well with the upper limit of the depth zone where sediments are disturbed (Figure 3). Alternatively, it is possible that the channel connecting a spring in the bedrock with the lake floor, where liquefied sediments migrate upward, was not stationary and may have been subject to lateral shifts over time. For example, an active channel may have become clogged, and vertical fluid flow would have then been displaced further away from the sampling location, allowing undisturbed sediment layering in the upper part of the core. Also, fluid flow and clay expulsion may have been more intense (or with more channels involved) before 1898, which led to the deformation of sediments over a larger area.
Daerligen Pockmark System DPS—Small Pockmarks Formed Through Continuous CH4 Ebullition
Results—The bathymetric data revealed the existence of several pockmarks of 10 to 21 m in diameter close to the Aare inflow at DPS (Figures 1, 2, 4). The pockmarks are positioned in the vicinity of a tectonic fault zone near Daerligen (Figure 1; Swiss Federal Office of Topography, swisstopo, Wabern, Switzerland), yet no conclusive evidence exists that confirms mechanistic links to the fault zone. Kullenberg-type sediment cores of 5.8 and 10 m length were collected at the pockmark (THU18-Ku6; Figure 2) and a reference site (THU18-Ku7), respectively. In addition, a ROV was used to investigate the activity of these pockmarks.
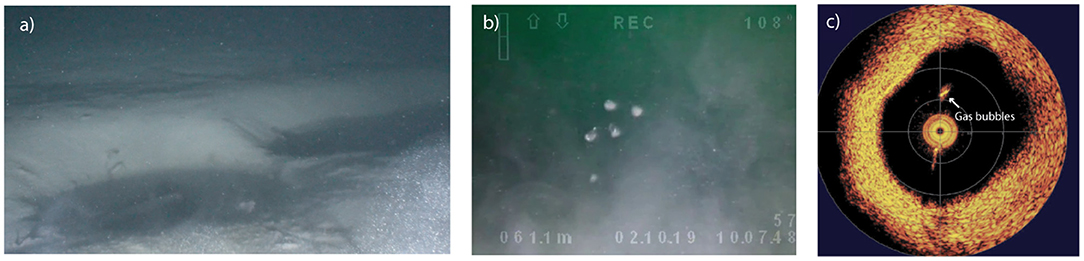
Figure 4. Selection of images of the DPS. Using the ROV, we cruised around the pockmark site (17 m south from the pockmark) that consists of a field of numerous smaller pockmarks than those observed on the bathymetric map (Figure 2). (a) Picture of pockmarks located in the area. (b) Gas bubbling in bottom waters coming directly from some of these pockmarks. (c) Sonar picture taken during the ROV descent showing gas bubbles in bottom waters above pockmarks. The picture shows the amplitude in dB where the yellow color represents high amplitude (e.g., reflection) while the orange toward black one show low amplitude sound echoes, respectively.
The biogeochemical vertical profiles of cations and anions were very similar at the reference and pockmark sites (Supplementary Figure 2). The concentration of most cations was constant in depth, except for Fe2+ and Ca2+, which increased up to ~40 and ~80 mg L−1, respectively, at about 100 cm depth and then decreased with depth. Similarly, anions concentration was quite constant with depth, except for that showed a peak at 300 cm depth, however, additional concentrations measurements are required to confirm this result. The character of the sediments is purely clastic, with basically no organic material (naked-eye and microscopic observations). The cored sediment sequence consists of clastic layers with thicknesses from the sub-mm to the dm scale (ca. 10 cm), deposited during flood events, which are intercalated by fine-grained lacustrine sediments consisting of clay and authigenic calcite (Figure 5). On average, porewater CH4 concentrations were slightly higher at the reference (250 m away from the pockmarks area; Figure 2) than at the pockmark site (Figure 5). At the reference site, CH4 concentration increased to a maximum of 255 μM at 66 cm depth and then tended to decrease in deeper sediment layers with relatively large variability. At the pockmark site, CH4 concentrations increased from ~40 μM in surface sediments to ~140 μM at 222 cm depth, again, with a high degree of variability. From 268 cm depth to 552 cm depth, CH4 concentrations decreased again (Figure 5). The δ13CH4 isotopic signature was typical for a microbial origin at the two sites (−70.6 ± −6.3 ‰ mean value, Figure 5; Whiticar, 1999).
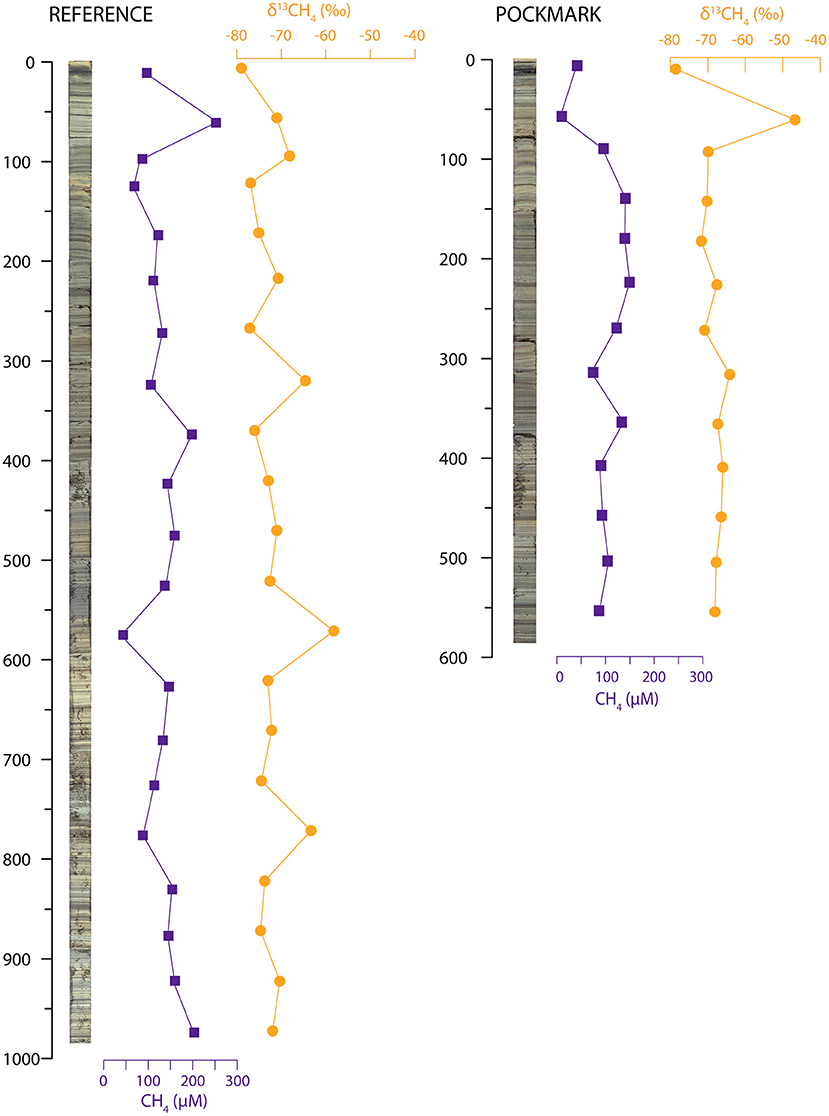
Figure 5. Vertical profiles of CH4 concentration and δ13CH4 isotopic signature of sediment cores collected at the pockmark and reference sites of DPS.
In addition to the pockmarks detected in the swath bathymetry, the ROV survey highlighted the presence of unit pockmarks (e.g., sub-pockmarks) of about 1.5 to 6 m in diameter, as well as intensive gas bubbling originating from some of these pockmarks (Figure 4; Supplementary Video 1). In oceans, unit pockmarks have been defined as “very small (<5 m) seabed depressions, which are found in isolation, in groups and in association with larger pockmarks” (Hovland and Judd, 1988). In order to determine the gas composition in bubbles, the ROV was placed in one of these pockmarks (6 m in diameter) and a gas sample was collected directly above the bubbling area. The gas sample was mostly CH4 (> 85%) of biogenic origin (δ13CH4 > −81‰). An attempt to collect bottom water and a sediment core within one of the active unit pockmarks without sample leakage failed unfortunately.
Unexpected similarities between pockmark and reference sites—In the attempt to unravel the mechanisms of formation and activity of these pockmarks, our results did not reveal any clear systematic differences between the reference and pockmark sites, neither with regard to the sedimentology nor with regard to the pore water and sediment biogeochemistry (Figure 5; Supplementary Figure 2). The CH4 concentrations at the two coring sites were lower than what has been measured in sediments in other Swiss lakes (Schubert et al., 2011; Cojean, 2019; Su et al., 2020). The fact that methane concentrations, in comparison to other lake sediments, were not elevated argue against methane oversaturation and gas expulsion as the driving mechanism during pockmark formation. It is very likely that we simply cored a non-active section of the “mother pockmark,” missing the center of one of the small active (i.e., bubbling) unit pockmarks visualized with the ROV. This highlights the importance of precise sampling via ROV surveys or even scuba diving for pockmark studies. We predict that within the active pockmarks, the sediment stratigraphy will be largely perturbed and deformed due to continuous gas ebullition, and the porewater CH4 concentration will be higher than at the reference site.
Methane in the studied pockmark is mostly of bacterial origin—Methane bubbling was recorded above the unit pockmarks of 1.5 to 6 m in diameter with the ROV survey, indicating their present activity. There are two potential sources for the evading methane. Thermogenic methane is generated when complex and long-chain organic molecules (i.e., kerogen) are degraded under high-temperature and high-pressure conditions at depths greater than 1 km (Whiticar, 1999; Judd et al., 2002). In contrast, bacterial CH4 is generally produced by methanogenic archaea that degrade organic matter from acetate or CO2 reduction in shallow anoxic sediment depths in which sulfate is depleted (Conrad, 2005). In sediments hosting high organic carbon concentration, CH4 levels can increase to exceed CH4 solubility, which allows the formation of gas bubbles. Once generated, CH4 migrates toward the atmosphere either through CH4 gas-bubble release (ebullition), diffusive transport when the overlying water is anoxic (Schmid et al., 2017), or advective transport by groundwater discharge (Schlüter et al., 2004; Hoffmann et al., 2020). The CH4 isotopic signature in the cored sediments at DPS (Figure 5) suggests a bacterial origin, with a δ13CH4 that is similar to the CH4 isotopic composition measured in several active pockmarks in Lake Constance, Germany (~-69 to −80 ‰; Bussmann et al., 2011). However, we did not observe an organic-rich interval within the vertical dimension of the cored sediment sequence, which could have provided source material for extensive CH4 formation. Hence, we conclude that the methane has its origin at a greater depth, in an organic-rich sediment section outside the reach of the used coring system. At the same time, a contribution from a deep thermogenic source cannot be fully excluded, particularly in light of the vicinity of the pockmarks to the tectonic fault zone. While the isotopic composition clearly speaks against a thermogenic CH4 origin, it has been shown before that a thermogenic CH4 signature may be altered into a mixed bacterial signal while migrating upwards through the sediments (Whiticar, 1999).
Tannmoos Pockmark System TPS—Unit Pockmarks Potentially Formed by Groundwater Discharge
Results—Two ROV surveys were performed in March 2017 and 2020 at TPS (Figures 1, 2). The 360° sonar picture revealed the presence of two large pockmarks of about 20–43 m diameter. One of them contains about 41 unit pockmarks (0.3–0.8 m diameter; Figure 6).
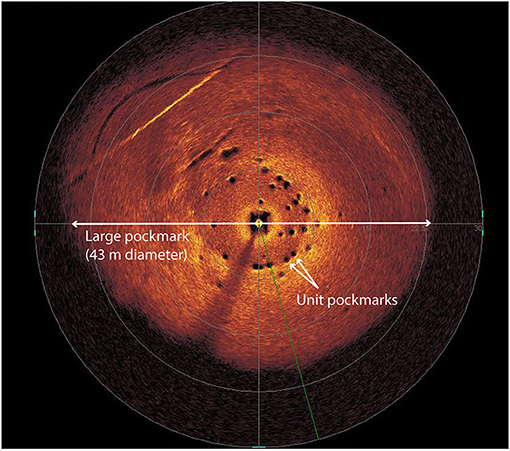
Figure 6. Sonar picture of the largest pockmark at TPS comprising several unit pockmarks of 0.3 to 0.8 m diameter.
During the ROV survey, no gas ebullition from pockmarks was observed, neither from the unit pockmarks nor from the larger ones. However, we detected the presence of intensive gas bubbling and water seepage outside the pockmarks along the Einigen Fault Zone (EFZ; Figure 1). As groundwaters usually have a higher ion strength than lake bottom waters, spikes in electroconductivity in lake bottom waters should be detected when an active groundwater discharge occurs (Wirth et al., 2020). However, when we monitored the electrical conductivity of the water above one of the studied unit pockmark, no ion-concentration anomalies were observed (data not shown), suggesting either that at least the investigated unit pockmark was indeed not active, or that groundwater discharge was too low to be detected.
In order to gain indirect constraints on the potential activity of past or present groundwater discharge, we again looked at the stratigraphy of the sediments inside the unit pockmark and compared it with the one of the reference site (30 m outside the large pockmark; Figure 2). The sediments at TPS reference and pockmark cores look very similar and are composed of grayish to yellowish clayey sediments with few mm-thick laminations (Figure 7). The sediments from 0–30 cm depth are lighter in color than the deeper sediments that are mostly grayish. The only difference observed between reference and pockmark core is that the short gravity core taken inside one of the unit pockmark revealed some slightly oblique layering between 2 and 13 cm depth, while such pattern was not observed at the reference site (Figure 7). Moreover, the sediment of the pockmark core seemed to contain more porewater than those of the reference core, as indicated by a stronger reflectance at the sediment surface. This may suggest that the unit pockmark sediments contain more pore-space, possibly suggesting groundwater discharge. However, clear evidence is still lacking. In contrast to the Beatenberg pockmark, no sediment layers (e.g., clayey sediments) were observed that may indicate sediment overflow induced by groundwater discharge (Figure 7).
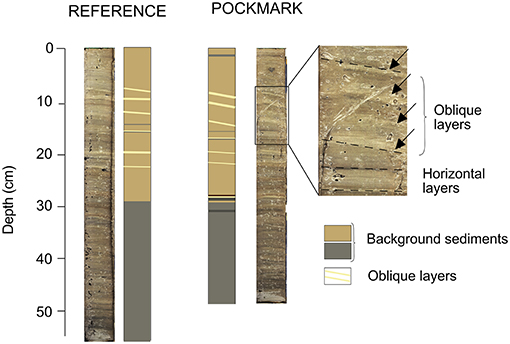
Figure 7. Photographs and lithology of short sediment cores collected inside a unit pockmark and at the reference site (outside the pockmark) at TPS.
Unit pockmarks activity likely triggered by groundwater discharge—Unit pockmarks have already been observed in marine systems but, due to their small size, they remained rather under-investigated, as their contribution to fluid seepage was thought to be negligible compared to larger pockmarks (Hovland et al., 2010). However, more recent studies challenged this assumption, demonstrating that unit pockmarks can significantly contribute to fluid release (Bussmann and Suess, 1998; Hovland et al., 2010; Hoffmann et al., 2020). In particular, Hovland et al. (2010) highlighted that the unit pockmarks present on the Norwegian continental shelf and slope were responsible for intense continuous local porewater seepage, while larger pockmarks seemed to be associated with periodic or intermittent gas ebullition. Similarly, unit pockmarks in Eckernförde have reported to be formed through episodic groundwater release to bottom waters (Hoffmann et al., 2020).
The large pockmarks at Tannmoos were described for the first time during a neotectonic survey of the lake performed by Fabbri et al. (2017). The authors highlighted the presence of several subaqueous morphologic depressions near a fault zone carrying gypsum bedrock (EFZ; Figure 1), along which we also detected, during the ROV survey, several subaquatic springs leading to groundwater discharge to the lake. The linear alignment of pockmarks, gas seeps, and subaquatic springs along the EFZ suggests that all of these different subaquatic features are (more or less) associated to fluid circulation along this fault system (Fabbri et al., 2017). Indeed, it has been shown previously that (re-)activation of faults can enhance water flow in karst systems (Häuselmann et al., 1999). The EFZ displayed repeated and ongoing phases of tectonic activity over time (Fabbri et al., 2017). It is thus possible that the TPS was formed during a reactivation event of the EFZ in the past, which led to an intense episodic groundwater discharge to the lake through different channels.
Since then, the present-day activity of the pockmarks is probably sustained by a facilitated, and episodic, flow of groundwater in the karst system, in particular during periods of intense precipitation (Häuselmann et al., 1999). In the present study, we focused our attention on the unit pockmarks that are comprised in one of the larger pockmarks (Figures 2, 6). If groundwater discharge still happens actively (continuously or episodically) at TPS, it likely occurs through these unit pockmarks rather than via the larger pockmarks, which seem presently in a dormant and inactive state.
Previous studies highlighted that subaquatic groundwater seepage is modulated by seasonal or shorter-term fluctuations in precipitation (often with a lag time of several days; Bussmann and Suess, 1998), and correlates with recharge intensity (Millham and Howes, 1994). Moreover, as shown during a survey on the upper Great Lakes, (Cherkauer and Nader, 1989), groundwater seepage to bottom waters tends to decrease with increasing distance from the shore. In contrast to the unit pockmarks, and their larger host pockmarks, at TPS, we observed continuous gas seepage and groundwater release via subaquatic springs located right along the fault zone. The fact that precipitation rates measured near TPS were relatively low the 14 days before sampling (1.3 and 0.7 mm/day on average measured at Interlaken and Thun; Meteosuisse IDAweb database), and that the pockmarks are located further away from the actual fault zone may explain the seemingly low activity, i.e., that the groundwater efflux through the investigated unit pockmark was either temporarily inactive, or too low to be detected at the time of sampling. We argue that unit pockmarks at TPS are, if at all, periodically active, most likely during periods of intense precipitation or snowmelt. Further investigation of the unit pockmarks combining hydrological, biogeochemical, and microbiological data collected during different seasons of the year should help to better constrain the present-day activity of the pockmark and assess its influence on the lake's hydrological budget. In order to gain further insight into pockmark activity in the past, future work should also include the retrieval of long sediment cores allowing the search for sediment structures and porewater chemical (e.g. water isotopes, main ions) signatures that indicate groundwater-driven fluid flow, and thus to gain a better understanding of the temporal context of pockmark activity and formation.
Conclusion
In this study, we investigated three different pockmark systems of variable morphology, mechanisms of formation, and activity within the same lake (Lake Thun, Switzerland). At Beatenberg, we detected the presence of a giant pockmark that is connected to a large karst system and that seems to be episodically activated through groundwater release, triggered by earthquakes, floods, or snowmelt. Here, evidence for past pockmark activity is clearly indicated by the expulsion of liquefied clayey sediments, producing distinct layers of overflow sediments. At Daerligen, the formation of multiple smaller pockmarks is/was likely associated to continuous CH4 ebullition, the origin of which must lay in the deeper subsurface, probably within organic rich sediment layers. The isotopic signatures of the analyzed CH4 suggest a bacterial origin. However, contribution from a thermogenic source of CH4 should at this stage not be fully excluded, simply because the area with the pockmarks is located close to a tectonic fault zone. Finally, at Tannmoos, large pockmarks (20–43 m diameter) were detected along the EFZ fault zone that is connected to a gypsum-carrying bedrock, which hosts several unit pockmarks located within them. At least one of these unit pockmarks may be episodically activated by groundwater discharge to the lake, however, the exact formation mechanisms and the controls on temporal activity fluctuations are still uncertain. Overall, this work underscores the potential importance of pockmarks in influencing hydrological and CH4 budgets in lakes through different mechanisms, and encourages further research in this field.
Data Availability Statement
The raw data supporting the conclusions of this article will be made available by the authors, without undue reservation.
Author Contributions
SW initiated the project. AC, SW, and KK collected samples. ML and MB provided the instrument for CH4 analysis and helped with the interpretation of the results. AC and SW wrote the manuscript with help from all co-authors. KK helped to prepare figures. All authors contributed to the article and approved the submitted version.
Funding
This study was funded by the Swiss National Science Foundation (SNF) project 175820 granted to SW.
Conflict of Interest
The authors declare that the research was conducted in the absence of any commercial or financial relationships that could be construed as a potential conflict of interest.
Publisher's Note
All claims expressed in this article are solely those of the authors and do not necessarily represent those of their affiliated organizations, or those of the publisher, the editors and the reviewers. Any product that may be evaluated in this article, or claim that may be made by its manufacturer, is not guaranteed or endorsed by the publisher.
Acknowledgments
We thank J. Matthieu for his help during the ROV campaigns and the Police of Lake Thun, R. Seifert, S. Bishop, A. Gilli, S. Wohlwend, L. Cheng, and J. Zimmermann for assistance during sampling on the lake. We also thank N. Dubois at the Department of Surface Waters Research and Management, EAWAG, and F. Anselmetti at the Institute of Geological Sciences, University of Bern, for the use of their laboratories. Finally, we thank P. Häuselmann for inputs on the Beatenberg karst system.
Supplementary Material
The Supplementary Material for this article can be found online at: https://www.frontiersin.org/articles/10.3389/frwa.2021.666641/full#supplementary-material
References
Bussmann, I., Schlömer, S., Schlüter, M., and Wessels, M. (2011). Active pockmarks in a large lake (Lake Constance, Germany): effects on methane distribution and turnover in the sediment. Limnol. Oceanogr. 56, 379–393. doi: 10.4319/lo.2011.56.1.0379
Bussmann, I., and Suess, E. (1998). Groundwater seepage in Eckernforde Bay (Western Baltic Sea): effect on methane and salinity distribution of the water column. Cont. Shelf Res. 18, 1795–1806. doi: 10.1016/S0278-4343(98)00058-2
Cathles, L. M., Su, Z., and Chen, D. (2010). The physics of gas chimney and pockmark formation, with implications for assessment of seafloor hazards and gas sequestration. Mar. Pet. Geol. 27, 82–91. doi: 10.1016/j.marpetgeo.2009.09.010
Charmoille, A., Fabbri, O., Mudry, J., Guglielmi, Y., and Bertrand, C. (2005). Post-seismic permeability change in a shallow fractured aquifer following a ML 5.1 earthquake (Fourbanne karst aquifer, Jura outermost thrust unit, eastern France). Geophys. Res. Lett. 32, 1–5. doi: 10.1029/2005GL023859
Cherkauer, D. S., and Nader, D. C. (1989). Distribution of groundwater seepage to large surface-water bodies: the effect of hydraulic heterogeneities. J. Hydrol. 109, 151–165. doi: 10.1016/0022-1694(89)90012-7
Cojean, A. N. Y. (2019). Environmental Controls of Benthic Nitrogen Cycling in Lake Lugano South Basin, Switzerland—Pathways, Rates, Isotopic Signatures and Microbial Communities. Basel: University of Basel.
Conrad, R. (2005). Quantification of methanogenic pathways using stable carbon isotopic signatures: a review and a proposal. Org. Geochem. 36, 739–752. doi: 10.1016/j.orggeochem.2004.09.006
Fabbri, S. C., Buechi, M. W., Horstmeyer, H., Hilbe, M., Hübscher, C., Schmelzbach, C., et al. (2018). A subaquatic moraine complex in overdeepened Lake Thun (Switzerland) unravelling the deglaciation history of the Aare Glacier. Quat. Sci. Rev. 187, 62–79. doi: 10.1016/j.quascirev.2018.03.010
Fabbri, S. C., Herwegh, M., Horstmeyer, H., Hilbe, M., Hübscher, C., Merz, K., et al. (2017). Combining amphibious geomorphology with subsurface geophysical and geological data: a neotectonic study at the front of the Alps (Bernese Alps, Switzerland). Quat. Int. 451, 101–113. doi: 10.1016/j.quaint.2017.01.033
Fäh, D., Giardini, D., Kästli, P., Deichmann, N., Gisler, M., Schwarz-Zanetti, G., et al. (2011). ECOS-09 Earthquake Catalogue of Switzerland Release 2011. Report and Data., Public Catalogue, 17.04.2011. Zürich: Swiss Seismological Service ETH Zürich.
Frey, S. E., Gingras, M. K., and Dashtgard, S.E. (2009). Experimental studies of gas-escape and water-escape structures: mechanisms and morphologies. J. Sediment. Res. 79, 808–816. doi: 10.2110/jsr.2009.087
Gaffet, S., Guglielmi, Y., Virieux, J., Waysand, G., Chwala, A., Stolz, R., et al. (2003). Simultaneous seismic and magnetic measurements in the Low-Noise Underground Laboratory (LSBB) of Rustrel, France, during the 2001 January 26 Indian earthquake. Geophys. J. Int. 155, 981–990. doi: 10.1111/j.1365-246X.2003.02095.x
Hainzl, S., Kraft, T., Wassermann, J., Igel, H., and Schmedes, E. (2006). Evidence for rainfall-triggered earthquake activity. Geophys. Res. Lett. 33, 1–5. doi: 10.1029/2006GL027642
Hammer, Ø., Webb, K. E., and Depreiter, D. (2009). Numerical simulation of upwelling currents in pockmarks, and data from the inner Oslofjord, Norway. Geo-Mar. Lett. 29, 269–275. doi: 10.1007/s00367-009-0140-z
Häuselmann, P. (2002). Cave Genesis and Its Relationship to Surface Processes: Investigations in the Siebenhengste Region (BE, Switzerland). Freiburg: University of Freiburg.
Häuselmann, P., Jeannin, P. Y., and Bitterli, T. (1999). Relationships between karst and tectonics: case-study of the cave system north of Lake Thun (Bern, switzerland). Geodin. Acta 12, 377–388. doi: 10.1080/09853111.1999.11105357
Hoffmann, J. J. L., Schneider von Deimling, J., Schröder, J. F., Schmidt, M., Held, P., Crutchley, G. J., et al. (2020). Complex eyed pockmarks and submarine groundwater discharge revealed by acoustic data and sediment cores in Eckernförde Bay, SW Baltic Sea. Geochem. Geophys. Geosyst. 21, 1–18. doi: 10.1029/2019GC008825
Hovland, M., Gardner, J. V., and Judd, A. G. (2002). The significance of pockmarks to understanding fluid flow processes and geohazards. Geofluids 2, 127–136. doi: 10.1046/j.1468-8123.2002.00028.x
Hovland, M., Heggland, R., De Vries, M. H., and Tjelta, T. I. (2010). Unit-pockmarks and their potential significance for predicting fluid flow. Mar. Pet. Geol. 27, 1190–1199. doi: 10.1016/j.marpetgeo.2010.02.005
Hovland, M., and Judd, A. G. (1988). Seabed Pockmarks and Seepages: Impact on Geology, Biology and the Marine Environment. London: Graham and Trotman
Judd, A., and Hovland, M. (2007). Seabed Fluid Flow: Impact on Geology, Biology, and the Marine Environment. Cambridge: Cambridge University Press. doi: 10.1017/CBO9780511535918
Judd, A. G., Hovland, M., Dimitrov, L. I., García Gil, S., and Jukes, V. (2002). The geological methane budget at continental margins and its influence on climate change. Geofluids 2, 109–126. doi: 10.1046/j.1468-8123.2002.00027.x
King, L. H., and MacLean, B. (1970). Pockmarks on the scotian shelf. Geol. Soc. Am. Bull. 81, 3141–3148. doi: 10.1130/0016-7606(1970)81[3141:POTSS]2.0.CO;2
Miller, S. A. (2008). Note on rain-triggered earthquakes and their dependence on karst geology. Geophys. J. Int. 173, 334–338. doi: 10.1111/j.1365-246X.2008.03735.x
Millham, N. P., and Howes, B. L. (1994). Nutrient balance of a shallow coastal embayment. 1.Patterns of groundwater discharge. Mar. Ecol. Prog. Ser. 112, 155–168. doi: 10.3354/meps112155
Moore, W. S. (2010). The effect of submarine groundwater discharge on the ocean. Ann. Rev. Mar. Sci. 2, 59–88. doi: 10.1146/annurev-marine-120308-081019
Niemann, H., Steinle, L., Blees, J., Bussmann, I., Treude, T., Krause, S., et al. (2015). Toxic effects of lab-grade butyl rubber stoppers on aerobic methane oxidation. Limnol. Oceanogr. Methods 13, 40–52. doi: 10.1002/lom3.10005
Painter, T. H., Flanner, M. G., Kaser, G., Marzeion, B., Van Curen, R. A., and Abdalati, W. (2013). End of the little ice age in the Alps forced by industrial black carbon. Proc. Natl. Acad. Sci. U. S. A. 110, 15216–15221. doi: 10.1073/pnas.1302570110
Pau, M., and Hammer, T. (2013). Sediment mapping and long-term monitoring of currents and sediment fluxes in pockmarks in the Oslofjord, Norway. Mar. Geol. 346, 262–273. doi: 10.1016/j.margeo.2013.09.012
Pickrill, R. A. (1993). Shallow seismic stratigraphy and pockmarks of a hydrothermally influenced lake, Lake Rotoiti, New Zealand. Sedimentology 40, 813–828. doi: 10.1111/j.1365-3091.1993.tb01363.x
Råman Vinnå, L., Bouffard, D., Wüest, A., Girardclos, S., and Dubois, N. (2020). Assessing subaquatic mass movement hazards: an integrated observational and hydrodynamic modelling approach. Water Resour. Manag. 34, 4133–4136. doi: 10.1007/s11269-020-02660-y
Räss, L., Simon, N. S. C., and Podladchikov, Y. Y. (2018). Spontaneous formation of fluid escape pipes from subsurface reservoirs. Sci. Rep. 8, 1–11. doi: 10.1038/s41598-018-29485-5
Ravazzi, C., Pini, R., Badino, F., De Amicis, M., Londeix, L., and Reimer, P. J. (2014). The latest LGM culmination of the Garda Glacier (Italian Alps) and the onset of glacial termination. Age of glacial collapse and vegetation chronosequence. Quat. Sci. Rev. 105, 26–47. doi: 10.1016/j.quascirev.2014.09.014
Reber, R., and Schlunegger, F. (2016). Unravelling the moisture sources of the Alpine glaciers using tunnel valleys as constraints. Terra Nov. 28, 202–211. doi: 10.1111/ter.12211
Rellstab, C., Keller, B., Girardclos, S., Anselmetti, F. S., and Spaaka, P. (2011). Anthropogenic eutrophication shapes the past and present taxonomic composition of hybridizing Daphnia in unproductive lakes. Limnol. Oceanogr. 56, 292–302. doi: 10.4319/lo.2011.56.1.0292
Reusch, A., Loher, M., Bouffard, D., Moernaut, J., Hellmich, F., Anselmetti, F. S., et al. (2015). Giant lacustrine pockmarks with subaqueous groundwater discharge and subsurface sediment mobilization. Geophys. Res. Lett. 42, 1–9. doi: 10.1002/2015GL064179
Roelofse, C., Alves, T. M., and Gafeira, J. (2020). Structural controls on shallow fluid flow and associated pockmark fields in the East Breaks area, northern Gulf of Mexico. Mar. Pet. Geol. 112:104074. doi: 10.1016/j.marpetgeo.2019.104074
Roy, S., Senger, K., Braathen, A., Noormets, R., Hovland, M., and Olaussen, S. (2014). Fluid migration pathways to seafloor seepage in inner Isfjorden and Adventfjorden, Svalbard. Nor. Geol. Tidsskr. 94, 99–199.
Schlüter, M., Sauter, E. J., Andersen, C. E., Dahlgaard, H., and Dando, P. R. (2004). Spatial distribution and budget for submarine groundwater discharge in Eckernförde Bay (Western Baltic Sea). Limnol. Oceanogr. 49, 157–167. doi: 10.4319/lo.2004.49.1.0157
Schmid, M., Ostrovsky, I., and McGinnis, D. F. (2017). Role of gas ebullition in the methane budget of a deep subtropical lake: what can we learn from process-based modeling? Limnol. Oceanogr. 62, 2674–2698. doi: 10.1002/lno.10598
Schnellmann, M., Anselmetti, F. S., Giardini, D., and McKenzie, J. A. (2005). Mass movement-induced fold-and-thrust belt structures in unconsolidated sediments in Lake Lucerne (Switzerland). Sedimentology 52, 271–289. doi: 10.1111/j.1365-3091.2004.00694.x
Schubert, C. J., Vazquez, F., Lösekann-Behrense, T., Knittel, K., Tonolla, M., and Boetius, A. (2011). Evidence for anaerobic oxidation of methane in sediments of a freshwater system (Lago di Cadagno). Fems Micriobiol. Ecol. 76, 26–38. doi: 10.1111/j.1574-6941.2010.01036.x
Strasser, M., Monecke, K., Schnellmann, M., and Anselmetti, F. S. (2013). Lake sediments as natural seismographs: a compiled record of Late Quaternary earthquakes in Central Switzerland and its implication for Alpine deformation. Sedimentology 60, 319–341. doi: 10.1111/sed.12003
Su, G., Zopfi, J., Yao, H., Steinle, L., Niemann, H., and Lehmann, M. F. (2020). Manganese/iron-supported sulfate-dependent anaerobic oxidation of methane by archaea in lake sediments. Limnol. Oceanogr. 65, 863–875. doi: 10.1002/lno.11354
Virtasalo, J. J., Schröder, J. F., Luoma, S., Majaniemi, J., Mursu, J., and Scholten, J. (2019). Submarine groundwater discharge site in the First Salpausselkä ice-marginal formation, south Finland. Solid Earth 10, 405–423. doi: 10.5194/se-10-405-2019
Wessels, M., Bussmann, I., Schloemer, S., Schlüter, M., and Böder, V. (2010). Distribution, morphology, and formation of pockmarks in Lake Constance, Germany. Limnol. Oceanogr. 55, 2623–2633. doi: 10.4319/lo.2010.55.6.2623
Whiticar, M. J. (1999). Carbon and hydrogen isotope systematics of bacterial formation and oxidation of methane. Chem. Geol. 161, 291–314. doi: 10.1016/S0009-2541(99)00092-3
Wirth, S. B. (2008). Lake Thun Sediment Record−300 Years of human Impact, Flood Events and Subaquatic Slides. Zürich: Swiss Federal Institute of Technology Zurich.
Wirth, S. B., Bouffard, D., and Zopfi, J. (2020). Lacustrine groundwater discharge through giant pockmarks (Lake Neuchatel, Switzerland). Front. Water 2:13. doi: 10.3389/frwa.2020.00013
Keywords: pockmarks, lacustrine groundwater discharge, earthquakes, lakes, chimney, ROV remotely operated vehicle
Citation: Cojean ANY, Kremer K, Bartosiewicz M, Fabbri SC, Lehmann MF and Wirth SB (2021) Morphology, Formation, and Activity of Three Different Pockmark Systems in Peri-Alpine Lake Thun, Switzerland. Front. Water 3:666641. doi: 10.3389/frwa.2021.666641
Received: 01 March 2021; Accepted: 08 July 2021;
Published: 04 August 2021.
Edited by:
Pamela L. Sullivan, Oregon State University, United StatesReviewed by:
Randy Stotler, University of Waterloo, CanadaJoonas Johannes Virtasalo, Geological Survey of Finland, Finland
Copyright © 2021 Cojean, Kremer, Bartosiewicz, Fabbri, Lehmann and Wirth. This is an open-access article distributed under the terms of the Creative Commons Attribution License (CC BY). The use, distribution or reproduction in other forums is permitted, provided the original author(s) and the copyright owner(s) are credited and that the original publication in this journal is cited, in accordance with accepted academic practice. No use, distribution or reproduction is permitted which does not comply with these terms.
*Correspondence: Adeline N. Y. Cojean, adeline.cojean@unine.ch