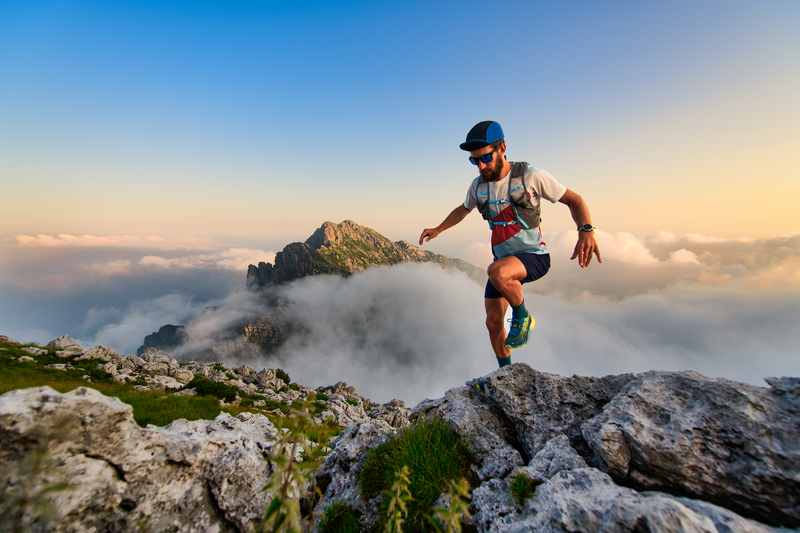
95% of researchers rate our articles as excellent or good
Learn more about the work of our research integrity team to safeguard the quality of each article we publish.
Find out more
ORIGINAL RESEARCH article
Front. Water , 09 July 2020
Sec. Water and Human Systems
Volume 2 - 2020 | https://doi.org/10.3389/frwa.2020.00014
This article is part of the Research Topic Modeling-based Approaches for Water Resources Problems - Volume II View all 5 articles
Groundwater management policy around the world increasingly seeks to protect groundwater-dependent ecosystems and associated human uses and values. This includes uses of ecosystems and agricultural systems linked to natural spring discharge. Yet, there are few examples of practical tools to balance human groundwater use with ecological water demand related to spring discharge. Using a simulation optimization framework, we directly incorporate a spring discharge constraint into the analysis of sustainable yield for operationalizing groundwater policy in the state of Hawai‘i. Our application on the island of O‘ahu is a spring discharge-dependent watercress farm with historical, cultural, and ecological significance. This research provides decision-makers in Hawai‘i with information regarding the trade-off between groundwater pumping and spring discharge, which is connected to multiple benefits, including historical and cultural values in line with codified state beneficial use protections. Because this trade-off provides an important step in operationalizing sustainable yield policy in Hawai‘i, we conclude by discussing further conceptual and technical developments necessary to move groundwater policy in Hawai‘i closer to full incorporation of the public trust principles of the state water code.
Groundwater sustainable yield evaluation is increasingly moving to consider multiple concerns beyond threats to quantity and quality of the freshwater resource available for extraction (Pierce et al., 2013; El-Kadi et al., 2014; Owen et al., 2019). While the need to include cultural, ecological, and social values in sustainable yield quantification is articulated in the literature (Alley and Leake, 2004), many existing definitions of sustainable yield are fairly general, e.g., “the development and use [of groundwater] in a manner that can maintain an aquifer for an infinite time without causing unacceptable environmental, economic, or social consequences” (Alley et al., 1999). Such broad definitions allow for flexibility in interpretation when dealing with complex systems and multiple values, and can be determined based on aquifer performance factors, governance structures, and societal preferences (Pierce et al., 2013; Elshall et al., 2020). Yet operationalizing a groundwater sustainability policy can be challenging in practice (Elshall et al., 2020). In this paper, we provide an example of sustainable yield estimation in Hawai‘i, wherein spring discharge serves as a metric for the continued provision of cultural, ecological, and social values related to the groundwater resource of interest.
The value of considering a range of diverse benefits in estimating sustainable groundwater pumping is particularly salient in geographically isolated areas like the Hawaiian Islands, where judicious management of limited local freshwater resources is essential to sustaining the well-being of residents and the health of linked ecosystems. Hawai‘i is heavily reliant on groundwater, with more than 90% of domestic freshwater sourced from aquifers (Tribble, 2008). There is a long-standing concern about stress on the state's groundwater resources (Chun et al., 2017). Sustainable management of the highly utilized Pearl Harbor aquifer on O‘ahu, in particular, requires consideration of multiple competing uses of water (Hutchins, 1946; Mink, 1980) as it supports a large portion of the population of Honolulu, as well as military, agricultural, and tourism sectors of the economy. Estimates of aquifer sustainable yield insofar as they are currently calculated do not explicitly take into account competing beneficial uses.
The objective of this paper is to assess the trade-off between optimal groundwater pumping and protecting the culturally and historically important spring system in Pearl Harbor aquifer, Hawai‘i under the state water code (HRS Chapter 174C, 1987). Groundwater management in Hawai‘i operates under the Hawai‘i State Water Code which specifies a number of protections under the public trust doctrine, including the maintenance of proper ecological balance as well as preservation and enhancement of waters for consumptive and recreational uses (HRS Chapter 174C, 1987). In particular, we consider the protection of spring discharge to Sumida Farm, an ecologically, socially, and historically important watercress (Nasturtium officinale) farm on the island of O‘ahu that is fully dependent on spring discharge for maintaining crop production. Our approach for estimating sustainable yield, defined here as optimal groundwater pumping given the aquifer performance and governance constraints, is described in detail in the methods section.
While returning spring discharge to historical pre-development levels is likely an unrealistic goal, we begin with this target and work backward to current levels of discharge to provide a clear description of tradeoffs for decision makers regarding the balance between sustainable yield and spring discharge. Working within the confines of existing water infrastructure, we use a simulation optimization method to estimate sustainable yield for varying levels of spring protection while maintaining the quality of pumped water. We then compare those values to existing estimates of sustainable yield, as well as current levels of pumping in the Pearl Harbor aquifer. Our framework allows us to infer an implied value of spring protection using a “tradeoff curve” between groundwater pumping and spring discharge. Our integrated groundwater management framework aims to improve integration and interlinkages of science, policy, and practice in a manner that is sufficiently protective of ecological, environmental, and cultural needs now and into the future.
The manuscript is organized as follows. The Materials and Methods section provides an overview of groundwater sustainable yield policy in Hawai‘i and how spring protection may be accounted for in this policy framework. It then introduces the Pearl Harbor aquifer study site and our modeling framework to estimate sustainable yield under different spring discharge thresholds. The Results section presents the results, and the Discussion section summarizes our findings and provides suggestions for groundwater sustainable yield policy applications in Hawai‘i.
Hawaiian culture and history play an influential role in contemporary water management, policy, and conflict (see Figure 1 for a description of three eras of water management in Hawai‘i). The current State Water Code was established in 1987, after a mandate for the creation of a state water management agency (Commission on Water Resource Management, or CWRM) was established during the 1978 Constitutional Convention. In addition to defining sustainable yield and outlining the State's obligation to manage water resources for the benefit of its people (i.e., per the public trust doctrine), the water code established the process for creating a Hawai‘i Water Plan as a guide for continued comprehensive water resources planning. The Water Resources Protection Plan component of the Hawai‘i Water Plan is prepared by CWRM and provides the overall legal and policy framework that guides the development, conservation, and use of water resources.
The state water code defines a number of protections under the public trust doctrine to balance with beneficial use: (1) the protection of traditional and customary Hawaiian rights, (2) the protection and procreation of fish and wildlife, (3) the maintenance of proper ecological balance and scenic beauty, and (4) the preservation and enhancement of waters of the state for consumptive and recreational uses. The legacy of water management structures that were put into place during the Plantation Era continues to lead to disputes and litigation to define the bounds of protections under the public trust nature of the state water code, especially with regards to traditional and customary Hawaiian rights (Beamer, 2004; Sproat, 2014). The placement under the law of water in the public trust itself is a reaffirmation of the indigenous Hawaiian tradition of resource management (MacKenzie, 2010). The water code further defines groundwater sustainable yield as “the maximum rate at which water may be withdrawn from a water source without impairing the utility or quality of the water source as determined by the commission.” The definition of sustainable yield in the water code does not provide quantitative measures for “utility” and “quality,” nor does the code quantify baselines toward which “protection,” “maintenance,” and “preservation” should be aiming. This is consistent with the concept of the public trust doctrine allowing the community members to manage the aquifer as a common pool resource, according to societal values and preferences.
The Robust Analytical Model (RAM) (Liu et al., 1981; Mink, 1981) was used to establish the values of sustainable yield per aquifer system that were included in the inaugural Water Resources Protection Plan in 1990. As a lumped analytical function type model, RAM requires only estimates of aggregate recharge and an estimate of the initial average head level of the aquifer, with saltwater intrusion as a driving constraint, to generate an estimate of sustainable yield. While the model has the benefit of being a simple management tool, state water management agencies recognized many of RAM's limitations, including the abstraction from a thick brackish transition zone that occurs at the freshwater-saltwater interface and the lumped (i.e., not spatially distributed) nature of the estimated sustainable yield outputs. Consequently, sustainable yield values published in the 2008 version of the WRPP (Wilson Okamoto Corporation, 2008) were updated based on a review of the original RAM SY estimates, RAM SY with updated recharge estimates, and RAM2 (Liu, 2006, 2007) SY estimates. While RAM2 more explicitly accounts for the brackish transition zone, it is still a lumped type model that cannot account for the effects of different pumping patterns at the individual well level. In 2019, CWRM updated the Water Resources Protection Plan (Townscape Inc., 2019), this time considering both RAM and RAM2 estimates with updated recharge information. In addition to improving the accuracy of existing methods to calculate sustainable yield values, the report emphasizes the importance of better understanding groundwater and surface water interactions, impacts to submarine groundwater and spring discharge, and the spatial distribution of water use impacts to the water supply. Our approach addresses many of those concerns by integrating a spring discharge constraint and spatially distributed pumping into a groundwater simulation optimization model for the estimation of sustainable yield in the Pearl Harbor aquifer.
Our research objective is to operationalize groundwater management by directly incorporating elements of the state water code, with particular focus on the protection of historically and culturally important spring resources. Our framework incorporates a simulation optimization procedure to estimate optimal maximum pumping given a spring discharge threshold, and salinity and drawdown constraints.
The Pearl Harbor aquifer is the state's most heavily utilized aquifer, and has spurred both the sustainable yield policy and methodology that is currently being used by groundwater managers in the state (Mink, 1980; Liu et al., 1981; Oki, 2005; Liu, 2006). The aquifer consists of a highly permeable stack of sub-aerial lava flows extending from land surface to approximately 1,800 meters below sea level, below which are less-permeable pillow lavas. Several geologic barriers, consisting of vertical dikes and post-erosional valley-fill deposits constrain lateral regional groundwater flow. The volcanic rift zones contain thousands of nearly vertical, low-permeability dikes that cut across existing lava flows, impounding water in numerous “dike compartments” (Takasaki and Mink, 1985; Oki, 2005). Vertical dikes act as subsurface dams that block lateral groundwater flow to great depths through the otherwise highly-permeable layered sub-aerial lavas, creating large differences in groundwater levels across the barrier. Low-permeability valley-fill deposits and weathered volcanic rocks beneath the valley-fill deposits impede shallow groundwater movement and create smaller differences in groundwater levels across the valley.
This aquifer is designated as a “groundwater management area” by the state. As such, the groundwater resource is protected by managing pumping rates through a permitting process. For management purposes, the Pearl Harbor Aquifer Sector is divided into three hydrologically connected systems, namely, the Ewa-Kunia system, the Waipahu-Waiawa system, and the Waimalu system (Figure 2). Our study site also includes the Moanalua aquifer system, which is part of Honolulu Aquifer Sector. Historical records have led to concerns regarding potential saltwater-intrusion effects due to large pumping rates from the Waimalu management system. Recharge to the Pearl Harbor aquifer is from direct infiltration of rainfall and irrigation water, as well as discharge from upgradient ground water bodies (for a figure of groundwater flow fields, see Oki, 2005, Figure 11) including the high-level dike compartments and the Schofield Plateau in central O‘ahu. Recharge estimates for this work were taken from Engott et al. (2017). Mean annual rainfall in the Pearl Harbor area ranges from 25 to 120 inches per year (Engott et al., 2017). Further details of the study site, including geological factors, land use and cover, and climate are described in detail by Oki (2005).
Figure 2. Study area. The location of four (sub-) aquifer units (Ewa-Kunia, Waipahu-Waiawa, Waimalu, and Moanalua), Sumida Farm (within Kalauao spring complex) and other coastal springs situated in the Pearl Harbor aquifer of O‘ahu.
Within the Pearl Harbor aquifer, there is a set of four inland springs near the margin of the caprock (Figure 2). According to Visher and Mink (1964), the main spring discharge is from areas where volcanic rocks are exposed at a break in slope of the land surface. Water also discharges as diffuse seeps throughout the aquifer area (Oki, 2005). Historically, the spring discharge was used for wetland crops such as rice, and is currently utilized for watercress cultivation and industrial purposes. Available data for spring discharge, which were utilized in model calibration, are displayed in Figure 26 of Oki (2005). Given the importance of Pearl Harbor aquifer, we selected it as a case study to present a transdisciplinary groundwater management framework that better aligns the operationalization of sustainable yield to its definition in the state water code. The approach and framework are general and can be applied to other sites even utilizing other simulation models.
We focus on a particular site within the Pearl Harbor aquifer, Sumida Farm, to demonstrate the importance of incorporating aspects such as spring discharge into sustainable yield evaluation. Sumida Farm is a ten-acre watercress farm and the only significant green space in the otherwise heavily urbanized Pearl City area. The third and current generation of owners now manages the farm with 11 full-time employees. Sumida Farm is the largest watercress producer in Hawai‘i, producing 70 percent of the watercress grown in the state. The crop is hand planted, harvested, and washed, delivering 4–5 tons per week to the O‘ahu market alone.
There are about a dozen watercress farms within the Pearl Harbor study area, including the Sumida farm, with a crop that can only be grown in free-flowing spring water. A defining characteristic of such a location is the access to abundant and naturally flowing fresh spring water from Kalauao Spring in the highly urbanized Pearl Harbor aquifer (Figure 2). Spring discharge at the farm has decreased by roughly 50% since the farm began in 1928, from 10 million gallons per day (MGD) to approximately 5 MGD by the 1990s (David Sumida, personal communication). During this period of decreasing spring discharge, the Sumida family observed lower yields, watercress “rot” in the hotter summer months, and increased levels of salinity within the plots. While there are likely several factors driving the decline in spring discharge, one possibility is the increase in groundwater pumping from the Pearl Harbor aquifer, especially the increasing quantities being withdrawn and delivered to the urban core of Honolulu to the east.
Aside from being the State's largest producer of watercress, Sumida Farm is a historically and culturally important resource to the local community, providing valuable green space in this highly urbanized area (Figure 3) as well as educational opportunities (Engels et al., in press). An average of 2,000 people visit Sumida every year, particularly children and senior citizens, to tour the farm, learn about local agriculture, and interact with the birds, crawfish, and other wildlife found within the plots. Sumida Farm provides and supports historical and community values (Figure 4). We use Sumida Farm as a case study to examine how protection of spring discharge to preserve such values may impact sustainable yield evaluation.
Figure 4. Multiple benefits associated with 100% spring-fed Sumida Farm. (A) Important habitat for birds, fish, and insects. (B) Cultural and community importance: Barbara and David Sumida, third-generation Sumida siblings and farmers. Moriichi and Makiyo Sumida, Barbara and David's grandparents started the farm in 1928. (C) Provision of goods: Bushels of fresh watercress bundled for delivery. (D) Employment: Sumida farmworker tending to the fields.
Keeping in mind the definition of sustainable yield and the requirements of the state water code, we identified the top three groundwater management objectives in collaboration with water management agencies as: (1) reduction of salinization risk to protect the resilience of the aquifer systems to saltwater intrusion, (2) minimization of drawdown to avoid over-drafting and upconing, and (3) conservation of spring discharge to restore or maintain traditional cultural Hawaiian sites and natural terrestrial ecosystems.
Quantifying constraints to address objectives (1) and (2) were fairly straightforward. For the simulation optimization procedure (described below), maximum salinity thresholds were set at 250 mg/l and 1,000 mg/l chloride at basalt and caprock wells, respectively. The basalt wells salinity threshold follows the U.S. Environmental Protection Agency's (EPA) secondary standard for drinking water. The caprock salinity threshold was recommended by the state's primary regulators, the Commission on Water Resource Management (CWRM), and is also consistent with the state's calculation for sustainable yield. A 5 m drawdown from the pre-development head-level was also enforced at all wells, where the head level is the groundwater table relative to the mean sea level.
Together, these constraints ensured an acceptable quality of pumped groundwater for potable uses. The third objective proved more challenging because the utility of spring discharge for cultural uses and natural ecosystems is not as clearly quantifiable without more specific definitions of “conservation” and “utility.” Ultimately, we conducted multiple simulation optimization runs for different levels of spring discharge constraints to generate a pumping-discharge tradeoff curve. More specifically, a range of minimum allowable spring discharge rate constraints was specified as percentages of the pre-development discharge rate (Oki, 2005).
Using data provided to CWRM by well operators, we initially identified 98 pumping wells in the Pearl Harbor groundwater area (Figure 5A). However, to reduce the problem ill-posedness and reduce the computational cost, we elicited feedback from CWRM on how best to reduce the number of decision variables. Many of the smaller wells (<1 MGD) are privately-owned (yellow circles in Figure 5A) and are a relatively small share of current aggregate pumping. Coordinating the management of pumping from many small privately-owned wells in accordance with an aquifer-scale optimization solution would be challenging. Thus, for both computational and practical reasons, the analyses were restricted to major wells with current pumping rates greater than or equal to 1 MGD: 27 key pumping wells (22 municipal wells, 3 federal wells, and 2 private wells). Pumping from wells currently pumping <1 MGD were held constant in all simulations. Current pumping for each well (Figure 5B) was estimated as the average pumping over the period 2001–2015 using data provided by CWRM.
Figure 5. Maps of the Pearl Harbor aquifer showing: (A) wells categorized by aquifer formation, aquifer management unit, and owner; (B) current pumping of pumping wells to identify potential decision variables) and current spring discharge; (C) optimal solution with 27 decision variables.
Reflecting the importance of the aquifer as a resource, numerical models have been utilized in Hawai‘i in addressing management questions regarding groundwater sustainability. Such models integrate available information with various water flow and chemical transport processes into a conceptual model and a suitable numerical (simulation) model is then chosen that is able to simulate the processes of concern (see, e.g., Souza and Voss, 1987, 1989; Voss and Souza, 1987; Voss, 1999; Gingerich and Voss, 2002, 2005; Oki, 2005). Although such models utilize a scenario simulation approach in answering management questions, they are not able to explicitly identify optimal policies.
We used a simulation optimization method to estimate sustainable yield in Pearl Harbor aquifer for a range of constraints designed to maintain spring discharge at the Kalauao spring complex, which includes Sumida Farm. Simulation optimization is a technique that uses simulation models and an optimization algorithm to maximize or minimize an objective function aimed at identifying optimal management solutions. We used the finite element groundwater SUTRA model of the Pearl Harbor Aquifer (Oki, 2005) to simulate groundwater flow and chloride transport. This model is based on a modified version of SUTRA 2.0 (Voss and Provost, 2002), which was designed to simulate three-dimensional, variable-density ground-water flow and solute transport in heterogeneous anisotropic aquifers. The model mesh consists of 306,432 nodes and 292,875 elements, covering the freshwater-lens system of the Pearl Harbor area and the adjacent Moanalua groundwater area to the east. The model's domain extends vertically 1,800 m below mean sea level to coincide with an assumed aquifer bottom and is laterally defined by no-flow, recharge, or specified-pressure boundaries. Pumping wells were represented in the model by the nearest vertical column of nodes, and discharge from the Pearl Harbor spring complex was modeled as a function of simulated head levels (Oki, 1998). Oki (2005) provides a detailed description of the density dependent groundwater SUTRA model of Pearl Harbor. The Oki (2005) model was calibrated by using published data to constrain values for permeability, storage, and dispersivity. The calibration was aimed at matching measured with simulated values of water levels, spring flows, and salinity profiles at wells in the modeled area. Because the distributions of parameter values were kept simple and some of these parameter values were not known, Oki cautions that additional pumping data in conjunction with water-level drawdown and recovery data is needed for complete calibration of the model. Still, we rely on this model as one trusted by the broader hydrological and water management stakeholder community in Hawai‘i. For the 50-year design period from 2021 to 2070, the simulation optimization scheme estimates the maximum allowable pumping in the Pearl Harbor aquifer without violating the salinity, head drawdown, and spring discharge constraints.
Spring discharge was among the management objectives that were identified by stakeholders as important (Chun et al., 2017) and directly in line with the Hawai‘i State Water Code. Therefore, to better understand the tradeoff between spring discharge protection and pumping, we ran the simulation optimization under four different scenarios regarding spring discharge: maintaining current spring discharge, and spring discharge constrained at 80%, 60%, and 40% of the pre-development level. The simulation optimization procedure utilizes the covariance matrix adaptation-evolution strategy (CMA-ES, Hansen et al., 2003), with parallel computing implementation (Elshall et al., 2015). CMA-ES is a stochastic derivative-free optimization algorithm that numerically estimates a covariance matrix of the decision variables to learn about the underlying objective function.
We use CMA-ES to link the objective function (see the Appendix for details) to the groundwater SUTRA model (Figure 6). Figure 6 illustrates the connection between the optimization and simulation modules. The decision variables (i.e., pumping wells with variable pumping rates) and constraints (i.e., reducing salinization risk, minimizing drawdown, conserving spring discharge) for the optimization problem were selected based on feedback from the state water management agency, CWRM. With the objective function in place, the CMA-ES optimization algorithm starts by generating a candidate spatial pumping setting, which is fed into the SUTRA groundwater model. SUTRA then simulates density dependent flow, and the SUTRA model outputs (i.e., head, salinity, and spring discharge) are evaluated against the set of constraints to calculate the objective function. The spatial setting of pumping rates is then adjusted given the objective function, and the process is repeated to determine optimal pumping.
Over a 50-year period with salinity, drawdown, and current levels of spring discharge constraints, the optimal maximum allowable pumping is 127 MGD (Figure 7C). For comparison, the current pumping rate of 117 MGD is already near the estimated optimal maximum, while the current designated sustainable yield value of 182 MGD using RAM2 is notably higher.
Figure 7. Simulation optimization results with spring discharge constrained at: (A) 80%, (B) 60%, (C) 40%. (D) Estimated spring discharge-SY tradeoff curve. Current pumping within the Pearl Harbor aquifer (117 MGD) allows for approximately 77 MGD of total spring discharge (marked with a diamond) given optimum pumping.
Given the requirements of the State Water Code, the next step was to incorporate spring discharge in the simulation optimization scheme. We then defined additional constraints as different percentages of that pre-development discharge level. Our simulation optimization results suggest that the estimated optimal maximum allowable pumping changes from 127 MGD to between 45 and 150 MGD, depending on the severity of the spring discharge constraint (Figure 7). As the discharge constraint is relaxed (shifting from Figures 7A–C), maximum allowable pumping rates increase and are more uniformly distributed across the aquifer. Given that the current pumping rate from the Pearl Harbor aquifer is 117 MGD, which is more than twice that required to restore 80% of the pre-development spring flow or higher, imposing such a strict constraint is likely infeasible in practice. We, therefore, estimated a “tradeoff curve” to illustrate different combinations of spring discharge constraints and optimal maximum allowable pumping estimate (Figure 7D).
If the relationship between spring discharge and socially, culturally, economically, and historically important resources within the Pearl Harbor aquifer could be more precisely quantified, then the tradeoff curve could be truncated. In the case of watercress production, for example, if data were available on the relationship between spring discharge quality or quantity and farm productivity, then we could focus on feasible discharge-pumping combinations that support a minimum level of production. In general, we believe that this type of approach could be extended to other situations in which pumping rates from the aquifer reduce the amount of discharge available for competing uses.
The simulation optimization results presented here are subject to several limitations, including objective function stability, potential interactions between state variables, solution convergence and repeatability, and others, as discussed in Elshall et al. (2015). The limitations of the numerical groundwater model are discussed in detail by Oki (2005).
This paper provides a framework for calculating optimal water pumping rates (here, “sustainable yield”) given a state water policy requiring the consideration of specific protections to social and ecological uses beyond meeting potable uses. In-so-doing, we provide a practical way to implement growing calls to incorporate valued groundwater dependent ecosystems into groundwater sustainability policies (Elshall et al., 2020). Although the process described here was borne out of local decision support needs identified through an initial engagement process, the framework is generalizable to other areas. The results of the simulation optimization method illustrate the impact and benefits of including spring discharge into groundwater allocation calculations. This approach provides an enhancement over the current method of calculating sustainable yield, which does not account for this component.
We developed a tradeoff curve between spring discharge and pumping to advance sustainable yield policy. The next steps would include further narrowing down preferences on where along the tradeoff curve the community is willing to be. That is, how much spring discharge is society willing to trade off for increased levels of pumping? A number of other targets are possible: protection of (measurable) ecological health, restoration of traditional wetland agriculture, other historical standards, or maintaining a percentage of pre-development levels. Alternatively, because it may not be realistic to maintain a target discharge level indefinitely given current and projected drying trends across the Hawaiian Islands (Elison Timm et al., 2014; Frazier and Giambelluca, 2017) and other external factors, another possibility is an adaptive target that evolves in response to changes in water supply and demand. Regardless of the target, the protection of spring discharge benefits should be balanced with consumptive and recreational uses in mind.
Without reducing total water demand, reducing total pumping to maintain a desired rate of spring discharge generally increases costs to other water users. With a constraint in place, a combination of behavioral, policy, and technological changes would be required to increase water use efficiency to some degree. Any remaining demand in excess of the maximum allowable pumping of groundwater would have to be met by an alternative, likely more expensive, water source. This would put upward pressure on water prices for all customers of the municipal water system, including domestic, commercial, industrial, and some agricultural users. At the same time, however, maintenance of spring discharge generates a number of benefits beyond those related to spring-dependent agriculture. Many coastal ecosystems, both terrestrial and marine, depend on groundwater discharge to some extent. Coral reef, algae, and fish health, for example, can be affected by changes in salinity or nutrient concentrations carried into the nearshore by groundwater (Duarte et al., 2010; Delevaux et al., 2018; Taniguchi et al., 2019). These ecosystem impacts can, in turn, affect social systems like commercial or subsistence fisheries and recreational activities for both residents and visitors. The latter of which is of particular concern for a location like Hawai‘i, where tourism accounts for a relatively large share of the economy.
While the analysis presented here did not attempt to quantify the many types of linkages between spring discharge and spring-dependent ecosystems, the illustrative tradeoff curve provides a practical way to visualize the tradeoff between aboveground groundwater uses and spring discharge quantities. We believe that this approach has broad relevance for stakeholders in the Pearl Harbor aquifer and beyond and can be used to initiate an iterative process of stakeholder-engaged science and scientifically informed policy.
The datasets generated for this study are available on request to the corresponding author.
Written informed consent was obtained from Barbara and David Sumida for the publication of any potentially identifiable images or data included in this article.
KB, AE, CW, CV, and LB: motivation and framing for the project. AE: simulation optimization method development and execution. AE-K and CV: simulation optimization method support. KB, AE, CW, and AA: manuscript development and writing. KB, AE, CW, and JD: figure and map development. AE-K, CV, JD, and LB: manuscript editing and improvements. All authors contributed to the manuscript revision, read, and approved the submitted version.
This work is dedicated to the memory of Barbara Masae Sumida. Our research was funded in part by National Science Foundation EPSCoR Award No. OIA 1557349 and National Science Foundation ICER/ASPIRE Grant No. 1645515.
The authors declare that the research was conducted in the absence of any commercial or financial relationships that could be construed as a potential conflict of interest.
We also gratefully acknowledge guidance from Gregory Chun and other partners from the Ike Wai project, and Roy Hardy and Lenore Ohye and team with the Hawai‘i Commission on Water Resource Management. This case study was inspired by our visits and conversations with the extended Sumida family: Barbara, David, and Stephen Sumida; John McHugh, and Emi and Kyle Suzuki, and would not have been possible without the leadership and help of Jennifer Engels. Delwyn Oki provided technical support with respect to the SUTRA model. David Schanzenback, Ron Merrill, and Sean Cleveland provided high performance computing technical support. The simulation-optimization runs were carried out using the high-performance computing environments of ITS-CI at the University of Hawai‘i System, and XSEDE (Towns et al., 2014). This work used the Extreme Science and Engineering Discovery Environment (XSEDE), which was supported by National Science Foundation grant number ACI-1548562; we used XSEDE TACC Dell/Intel Knights Landing, Skylake System (Stampede2) and TACC Long-term tape Archival Storage (Ranch) through allocation EAR190024 New. We thank two reviewers for providing feedback that helped to improve the article. This is contributed paper WRRC-CP-2020-14 of the Water Resources Research Center, and SOEST publication #11083 University of Hawai‘i at Manoa, Honolulu, Hawai‘i.
Alley, W. M., and Leake, S. A. (2004). The journey from safe yield to sustainability. Groundwater 42, 12–16. doi: 10.1111/j.1745-6584.2004.tb02446.x
Alley, W. M., Reilly, T. E., and Franke, O. L. (1999). Sustainability of Groundwater Resources. Denver, CO: U.S. Geological Survey circular. doi: 10.3133/cir1186
Chun, G., Adler, P., Arik, A. D., Burnett, K. M., Redding, N., and Wada, C. A. (2017). Organizational Study of Water Management Networks and Processes in Hawai‘i. Honolulu, HI: Study conducted and prepared for The Ulupono Initiative.
Delevaux, J. M. S., Jupiter, S. D., Stamoulis, K. A., Bremer, L. L., Wenger, A. S., Dacks, R., et al. (2018). Scenario planning with linked land-sea models inform where forest conservation actions will promote coral reef resilience. Sci. Rep. 8:12465. doi: 10.1038/s41598-018-29951-0
Duarte, T. K., Pongkijvorasin, S., Roumasset, J., Amato, D., and Burnett, K. (2010). Optimal management of a Hawaiian Coastal aquifer with nearshore marine ecological interactions. Water Resour. Res. 46:11545. doi: 10.1029/2010WR009094
Elison Timm, O., Giambelluca, T. W., and Diaz, H. F. (2014). Statistical downscaling of rainfall changes in Hawai'i based on the CMIP5 global model projections. J. Geophys. Res. 120, 92–112. doi: 10.1002/2014JD022059
El-Kadi, A. I., Tillery, S., Whittier, R. B., Hagedorn, B., Mair, A., Ha, K., et al. (2014). Assessing sustainability of groundwater resources on Jeju Island, South Korea, under climate change, drought, and increased usage. J. Hydrogeol. 22, 625–642. doi: 10.1007/s10040-013-1084-y
Elshall, A. S., Arik, A. D., El-Kadi, A., Pierce, S., Ye, M., Burnett, K. M., et al. (2020). Groundwater sustainability: a review of the interactions between science and policy. Environ. Res. Lett. doi: 10.1088/1748-9326/ab8e8c
Elshall, A. S., Pham, H. V., Yan, L., Tsai, F.T.-C., and Ye, M. (2015). Parallel inverse modeling and uncertainty quantification of computationally demanding groundwater flow models using covariance matrix adaptation. J. Hydrol. Eng. 20:04014087. doi: 10.1061/(ASCE)HE.1943-5584.0001126
Engels, J. L., Watson, S., Dulai, H., Burnett, K. M., Wada, C. A., and Aga, A. (in press). Collaborative research to support urban agriculture in the face of change: the case of the Sumida watercress farm on O'ahu. PLOS One.
Engott, J. A., Johnson, A. G., Bassiouni, M., Izuka, S. K., and Rotzoll, K. (2017). Spatially Distributed Groundwater Recharge for 2010 Land Cover Estimated Using a Water-Budget Model for the Island of O‘ahu, Hawai‘i (ver. 2.0, December 2017). U.S. Geological Survey Scientific Investigations Report. doi: 10.3133/sir20155010
Frazier, A. G., and Giambelluca, T. W. (2017). Spatial trend analysis of Hawaiian Rainfall from 1920 to 2012. Int. J. Climatol. 37, 2522–2531. doi: 10.1002/joc.4862
Gingerich, S. B., and Voss, C. I. (2002). “Three-dimensional variable-density flow simulation of a coastal aquifer in southern Oahu, Hawaii, U. S. A,” in Proceedings of 17th Salt Water Intrusion Meeting (SWIM-17), Delft University of Technology (Delft), 93–103.
Gingerich, S. B., and Voss, C. I. (2005). Three-dimensional variable-density flow simulation of a coastal aquifer in southern Oahu, Hawaii, U. S. A. Hydrogeol. J. 13, 436–450. doi: 10.1007/s10040-004-0371-z
Hansen, N., Müller, S. D., and Koumoutsakos, P. (2003). Reducing the time complexity of the derandomized evolution strategy with covariance matrix adaptation (CMA-ES) Evol. Comput. 11, 1–18. doi: 10.1162/106365603321828970
Hutchins, W. A. (1946). The Hawaiian System of Water Rights. Honolulu, HI: Honolulu Board of Water Supply.
Liu, C. C. K. (2006). Analytical Groundwater Flow and Transport Modeling for the Estimation of the Sustainable Yield of Pearl Harbor Aquifer. Water Resources Research Center, University of Hawaii at Manoa.
Liu, C. C. K. (2007). RAM2 Modeling and the Determination of Sustainable Yields of Hawaii Basal Aquifers. Water Resources Research Center, University of Hawaii at Manoa.
Liu, C. C. K., Lau, L. S., and Mink, J. F. (1981). Numerical Simulation of a Thick Freshwater Lens: Pearl Harbor Groundwater Model. Water Resources Research Center, University of Hawaii at Manoa.
MacKenzie, M. K. (2010). Hawaiian custom in Hawai'i state law. Yearbook of New Zealand Jurisprudence 13/14, 112–151.
Mink, J. F. (1980). State of the Groundwater Resources of Southern Oahu. Honolulu, HI: Board of Water Supply.
Mink, J. F. (1981). Determination of sustainable yields in basal aquifers of Hawai‘i Groundwater,” in Hawai‘i: A Century of Progress, eds F. N. Fujimura and W. B. C. Chang (Honolulu, HI: University Press of Hawaii), 101–116.
Oki, D. S. (1998). Geohydrology of the Central Oahu, Hawaii, Ground-Water Flow System and Numerical Simulation of the Effects of Additional Pumping. U.S. Geological Survey Water-Resources Investigations Report 97-4276.
Oki, D. S. (2005). Numerical Simulation of the Effects of Low-Permeability Valley-Fill Barriers and the Redistribution of Ground-Water Withdrawals in the Pearl Harbor Area, Oahu, Hawaii. U.S. Scientific Investigations Report 2005–5253. doi: 10.3133/sir20055253
Owen, D., Cantor, A., Nylen, N. G., and Harter, T. Kiparsky, M. (2019). California groundwater management, science-policy interfaces, and the legacies of artificial legal distinctions. Environ. Res. Lett. 14:4. doi: 10.1088/1748-9326/ab0751
Pierce, S. A., Sharp, J. M., Guillaume, J. H. A., Mace, R. E., and Eaton, D. J. (2013). Aquifer-yield continuum as a guide and typology for science-based groundwater management. Hydrogeol. J. 21, 331–340. doi: 10.1007/s10040-012-0910-y
Souza, W. R., and Voss, C. I. (1987). Analysis of an anisotropic coastal aquifer using variable-density flow and solute transport simulation. J. Hydrol. 92, 17–41. doi: 10.1016/0022-16948790087-4
Souza, W. R., and Voss, C. I. (1989). “Assessment of potable ground water in a fresh water lens using a variable-density flow and solute transport simulation,” in Proceedings of the NWWA Conference on Solving Ground Water Problems With Models (Indianapolis, IN).
Sproat, D. K. (2014). “From Wai to Kānawāi: Water Law in Hawai‘i,” in Native Hawaiian Law: A Treatise, eds M. K. MacKenzie, S. K. Serrano, and D. K. Sproat (Honolulu, HI: Kamehameha Publishing), 90.
Takasaki, K. J., and Mink, J. F. (1985). Evaluation of major dike-impounded groundwater reservoirs, island of Oahu. U.S. Geological Survey Water-Supply Paper 2217.
Taniguchi, M., Dulai, H., Burnett, K. M., Santos, I. R., Sugimoto, R., Stieglitz, T., et al. (2019). Submarine groundwater discharge: updates on its measurement techniques, geophysical drivers, magnitudes, and effects. Front. Environ. Sci. 7:141. doi: 10.3389/fenvs.2019.00141
Towns, J., Cockerill, T., Dahan, M., Foster, I., Gaither, K., Grimshaw, A., et al. (2014). XSEDE: accelerating scientific discovery Comput. Sci. Eng. 16, 62–74. doi: 10.1109/MCSE.2014.80
Tribble, G. (2008). Ground Water on Tropical Pacific Islands–Understanding a Vital Resource. U.S. Geological Survey Circular 1312. doi: 10.3133/cir1312
Visher, F. N., and Mink, J. F. (1964). Groundwater resources in southern Oahu. Hawaii, HI: U.S. Geological Survey Water-Supply Paper 1778.
Voss, C. I. (1999). “USGS SUTRA Code – history, practical use, and application in Hawaii,” in Seawater Intrusion in Coastal Aquifers – Concepts, Methods and Practices, eds J. Bear, A.H.-D. Cheng, S. Sorek, D. Ouzar, I. Herrera (Boston, MA: Kluwer Academic Publishers), 249–313. doi: 10.1007/978-94-017-2969-7_9
Voss, C. I., and Provost, A. M. (2002). SUTRA, A Model for Saturated-Unsaturated Variable-Density Ground-Water Flow With Solute or Energy Transport. U.S. Geological Survey Water Resources Investigation Report 02-4231250.
Voss, C. I., and Souza, W. R. (1987). Variable density flow and solute transport simulation of regional aquifers containing a narrow freshwater-saltwater transition zone. Water Resour. Res. 23, 1851–1866 doi: 10.1029/WR023i010p01851
Wilson Okamoto Corporation (2008). Hawaii Water Resource Protection Plan. State of Hawaii Commission on Water Resource Management.
The object function is to maximize allowable pumping without violating the constraints. For any m decision variables (pumping wells), we used a pumping-dependent objective function
such that the solution will be penalized if the chloride concentration or drawdown, Wi, obs, in any pumping well i exceeds its chloride concentration or drawdown threshold, Wi, threshold; qi is pumping from well i; and pi is a penalty term. The weighting term, ri, represents the relative importance of pumping well i, and we assume equal weighting for all decision variables. We observe the chloride concentration and drawdown, Wi, obs, for each well at the end of the simulation period (50 years). The spring objective function is a hard penalty constraint
such that Sobs is the total spring discharge observed at the end of the simulation period (50 years); Sthreshold is the spring discharge threshold for all springs combined; and p is a penalty term for total spring discharge.
Keywords: groundwater dependent ecosystems, sustainable yield, sustainable groundwater management, spring discharge, Hawai‘i state water code, water policy, simulation optimization, Pearl Harbor aquifer
Citation: Burnett KM, Elshall AS, Wada CA, Arik A, El-Kadi A, Voss CI, Delevaux JMS and Bremer LL (2020) Incorporating Historical Spring Discharge Protection Into Sustainable Groundwater Management: A Case Study From Pearl Harbor Aquifer, Hawai‘i. Front. Water 2:14. doi: 10.3389/frwa.2020.00014
Received: 13 March 2020; Accepted: 29 May 2020;
Published: 09 July 2020.
Edited by:
Roman Seidl, Leibniz University Hannover, GermanyReviewed by:
Lindsey M. W. Yasarer, United States Department of Agriculture, United StatesCopyright © 2020 Burnett, Elshall, Wada, Arik, El-Kadi, Voss, Delevaux and Bremer. This is an open-access article distributed under the terms of the Creative Commons Attribution License (CC BY). The use, distribution or reproduction in other forums is permitted, provided the original author(s) and the copyright owner(s) are credited and that the original publication in this journal is cited, in accordance with accepted academic practice. No use, distribution or reproduction is permitted which does not comply with these terms.
*Correspondence: Kimberly M. Burnett, a2J1cm5ldHRAaGF3YWlpLmVkdQ==
Disclaimer: All claims expressed in this article are solely those of the authors and do not necessarily represent those of their affiliated organizations, or those of the publisher, the editors and the reviewers. Any product that may be evaluated in this article or claim that may be made by its manufacturer is not guaranteed or endorsed by the publisher.
Research integrity at Frontiers
Learn more about the work of our research integrity team to safeguard the quality of each article we publish.