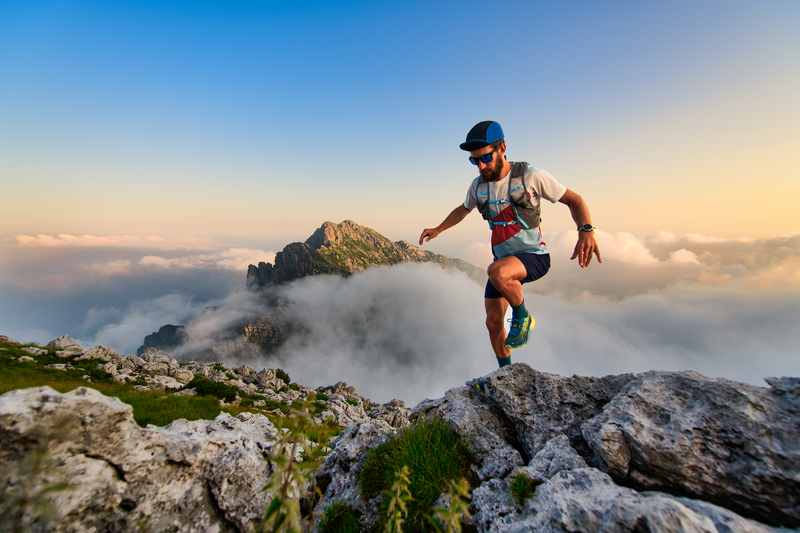
95% of researchers rate our articles as excellent or good
Learn more about the work of our research integrity team to safeguard the quality of each article we publish.
Find out more
ORIGINAL RESEARCH article
Front. Virtual Real. , 02 June 2022
Sec. Virtual Reality in Industry
Volume 3 - 2022 | https://doi.org/10.3389/frvir.2022.891584
Increasing demand for cage-free eggs arises from goals to provide hens with better welfare, particularly in terms of natural behavior. However, most laying hens are kept in conventional cages, and cage-free systems can present challenges, such as injuries, floor eggs, and bacterial infections. We proposed using virtual reality (VR) as a feasible means for combining the positive attributes of natural environments while mitigating health risks. To our knowledge, no animal study has provided evidence that VR can trigger biological changes to improve animal health and well-being nor whether VR can affect the gut microbiota. In this study, we used VR technology to simulate a natural environment in laying hen housing. Early-lay White Leghorn hens were placed in pens with (VR) or without (CON) video projections displaying free-range chickens interacting with indoor and outdoor environmental features over 5 days. Using in vitro blood bactericidal assays, VR hens exhibited higher resistance against avian pathogenic Escherichia coli versus CON (p < 0.05), which was positively associated with corticosterone levels (p < 0.01). Analyzing intestinal neurochemicals via ultra-high pressure liquid chromatography, salsolinol was the only neurochemical metabolite affected by VR, being greater in CON ileal content (p < 0.0001), in VR ileal mucus (p < 0.01), and in VR ceca tissue (p < 0.05). Using 16S rRNA sequencing and QIIME2 analyses, no differences in alpha nor beta diversity were determined between groups. Although several genera (Megamonas, Ruminococcus, Slackia) were reduced in VR hens versus CON, Mucispirillum schaedleri (member of Deferribacteres Phylum) was the only taxon increased in VR hens, being elevated in ileal mucus (p < 0.05). Lastly, using the QIIME2 plugin mmvec to map microbe-metabolite co-occurrences, we identified several positive relationships between bacterial phyla and neurochemical metabolites, notably finding dopamine and salsolinol levels were related to Deferribacteres and Tenericutes levels. In conclusion, we found that several biological parameters were influenced by VR treatment in hens, suggesting that VR can be used to improve host resistance to pathogens and gut health in poultry.
Commercial poultry are housed in high-density environments to maximize output of products meat and eggs. However, in many caged and cage-free facilities, chickens lack complex natural environmental features with biological relevance, drawing ethical concerns from the public. Although public interests have pushed commercial egg farms to shift towards cage-free housing, cage-free animals are at higher risk for infections, and undesirable behaviors (e.g. cannibalism), as well as higher rates of injury and mortality (Heerkens et al., 2015). Although free-range operations are also used to raise chickens, these conditions present greater risks of predation, parasites/pathogens (Bestman and Bikker-Ouwejan, 2020), and exposure to inclement weather (Singh et al., 2017). Thus, technologies that allow the combination of naturalistic stimuli of free-range housing with the productivity and husbandry benefits in poultry facilities are drastically needed.
Virtual reality (VR) has recently garnered much attention in human medicine given its benefits on post-traumatic stress (Maples-Keller et al., 2017), sports training (Stinson and Bowman, 2014), autism spectrum disorder (Yang et al., 2018), and stroke rehabilitation (Maier et al., 2019). In addition to humans, VR modulates social behaviors in fish, mice, and flies (Stowers et al., 2017). To our knowledge, no study has demonstrated if VR can be protective against infectious disease or whether it can induce changes in the intestinal environment (microbiota, neurochemical production, etc.).
Compared to humans, birds’ vision is tetrachromic with poor depth perception and they better recognize objects when they are moving than stationary (Broom, 1969; Dawkins and Woodington, 2000). Environment factors, such as color, light quality, duration, and intensity affect feeding behaviors of poultry (Lien et al., 2008). Additionally, video images reduces fear response, as birds quickly approach the video image to which they are exposed (Clarke and Jones, 1999). Furthermore, when looking at a video of chicks feeding, birds will imitate these behaviors and approach their feed more quickly (Clarke and Jones, 2001). Thus, chickens are highly receptive to visual stimuli, and VR design should be adapted to accommodate the sensory preferences of chickens. However, the extent to which biological functions aside from behavior are affected by VR is unclear.
Overall, we hypothesized that VR could be designed to impact biological factors, and thus improve laying hen welfare and health. The objectives of the study were to 1) create a virtual free-range environment from video recordings of free-range chickens performing behaviors associated with positive affect (e.g., nesting, dustbathing, preening, perching) to display on the walls of an enclosed pen, 2) test the effect of VR on overall chicken health and welfare, 3) characterize stress and immunological responses to VR, and 4) investigate how VR exposure may affect neurochemical-microbial interactions in the chicken intestine.
Animal experiments were approved by Iowa State University Institutional Animal Care and Use Committee, log #19-211. Animal distress was minimized during experimental procedures via enrichments (ex: perches). Open-floor pens were implemented to enable social interactions between chickens, and chickens were fed with no-medicated feed and water ad libitum. Upon experiment completion at 5 days post pen-placement, chickens were euthanized via CO2 asphyxiation in accordance with American Veterinary Medical Association Guidelines (2020).
Chickens used in video recordings for the creation of VR were enrolled from and maintained on three privately owned backyard flocks in Iowa during summer 2019. These flocks consisted of mature hens, roosters, pullets, and chicks, including a diversity of fancier-type egg-laying, meat and dual-purpose breeds. Housing included one or more indoor facilities, equipped with perches, nest boxes, feeders, drinkers and litter areas, with access to an outdoor fenced scratch area or unfenced open prairie with grasses, shrubs and flowers. Chickens used in the laboratory testing of VR consisted of 34 White Leghorn hens (Dekalb Whites) at early-lay stage (15 weeks post-hatch), randomly split into two groups, one exposed to virtual reality (VR) and the other unexposed (CON) (n = 15) via staff blinded to the experimental groups. Birds were individually identified using food dye on plumage and colored leg bands. Each group was housed in a 9 ft × 9 ft × 7 ft pen with steel spindle walls and pine shavings litter, and pens were maintained at 74°F. In both VR and CON rooms, pen walls were wrapped with vinyl projector screen material on all four sides. Each pen was equipped with a feed hopper, drinker, perches, nest boxes, and a dustbathing area. The photoperiod was 16L:8D. Husbandry consisted of daily inspection of the chickens, replenishing water and a commercial layer diet was provided ad libitum.
Hens from the VR and control groups were housed in 9 ft × 9 ft × 7 ft steel cages wrapped with vinyl projector screen material on all four sides. In the VR pen (Figure 1), video-recorded scenes of free-range chickens (see Supplemental Materials for example videos) were projected on each of the four walls during daylight hours. Each wall depicted a free-range flock interacting with one of the following scenes: 1, perching area; 2, nesting area; 3, outdoor dirt yard scratch area; 4, outdoor vegetative area. The storyboard for wall was constructed with hourly segments depicting scenes intended to provoke desirable behaviors (e.g., nesting, dustbathing, preening, perching) appropriate to the known ethogram and time budget of the laying hen. For example, VR projected behind the nest boxes displayed hens actively performing nesting behavior during the morning hours, when oviposition typically occurs, whereas empty nests with no hens present were displayed during afternoon and evening hours when oviposition is unlikely. The perching area VR storyboard switched hourly between still images of perches with no hens present, followed by video images of hens actively perching during the daylight hours, and concluding with a still image of perches with motionless hens perching immediately prior to and during the dimming lights and dark periods.
FIGURE 1. Representative pictures of Virtual Reality (VR) outside (A,B) and inside (C) of the chickens’ Pens. VR images were projected onto the vinyl projector screens placed against each pen wall in the VR group only.
Prior to necropsy, all hens were weighted and evaluated according to welfare characteristics, including plumage damage, cleanliness, keel bone deformation, comb pecking wounds and comb abnormality as previously described (Oliveira et al., 2019).
Prior to euthanasia at day 5 of VR or control treatment, fresh blood (100 µL) was collected via wing vein via filter-sterilized heparin (1,000 U/ml)-coated needles and immediately placed in citrate-coated tubes at room temperature for 4 h. Blood was centrifuged at 4,000×g for 20 min, and non-hemolyzed serum was individually stored at −80°C. For quantification of corticosterone, serum samples were run in triplicate using a commercial corticosterone ELISA kit (Enzo Life Sciences) in accordance to the manufacturer directions.
Prior to euthanasia at day 5 of VR or control treatment, fresh blood (200 µL) was collected from the right-wing vein of hens via filter-sterilized heparin-coated needles and placed on ice. Blood was evenly split into 2 pools per treatment group (n = 7 hens per pool), then diluted 1:8 in CO2-independent media with 2 mM L-glutamine (Gibco). Cell viability was determined to be greater than 98% via trypan blue staining for all pools. Prior to experiments, reference E. coli strains (Table 1) were stored in peptone-glycerol solution at -80°C. Strains were grown in Luria-Bertani (LB) agar (0.1% glucose) overnight at 37°C, colonies were mixed into phosphate-buffered saline (PBS) until OD600 reached 0.1, and solutions were diluted to reach 102 CFU/20 µL. Individual wells in 96-well plates were filled with whole blood solution and bacterial inoculum (9:1, respectively) and incubated for 30 min at 40°C. After brief resuspension, samples were serially diluted, plated on MacConkey agar, and incubated overnight at 37°C. Assays were performed in triplicate.
To calculate H:L ratios, a biomarker for stress (Gross and Siegel, 1983), approximately 3 μL of fresh blood (acquired prior to euthanasia as described earlier) were aliquoted and smeared onto a standard microscope slide (one slide per hen, n = 15 per group), briefly air dried, fixed and stained using a Neat Stain Hematology Staining Kit (Polysciences, Inc.). For each slide, approximately 200 leukocytes were enumerated via light microscopy based on phenotypic characteristics (de Macchi et al., 2013). Thereafter, H:L ratios were then calculated by averaging heterophils and lymphocytes identified within each slide.
Post-euthanasia at day 5 of VR or control treatment, intestinal segments (i.e., ileum and ceca) were aseptically removed from birds in both groups (n = 15 per group). Ileal and cecal contents were squeezed from the intestinal segments into respective tubes. Mucus from each section was collected by longitudinally dissecting intestinal tissues to expose the intestinal lumen and then gently scraping the mucosa with fresh, autoclaved glass microscope slides, which were then directly transferred into respective cryotubes. The remaining tissues were then placed into respective cryotubes. All sample cryotubes were briefly homogenized with 0.2 M perchloric acid (1:10 sample-acid ratio) and flash-frozen via liquid nitrogen prior to −80°C storage. For neurochemical and short chain fatty acid analyses, samples were homogenized using bead mill homogenization (Omni Bead Ruptor Elite) and centrifuged at 2,950 × g at 4°C. Thereafter, supernatant was transferred to 2–3 kDa spin filter and centrifuged again at 2,950 × g at 4°C. Flow-through was collected and then analyzed via ultra-high-performance liquid chromatography with electrochemical detection (UHPLC-ED) using the 99 Dionex UltiMate 3000 with MD-TM Mobile Phase Solution as sample diluent (Fisher Scientific) as described previously (Villageliú et al., 2018b).
Total DNA was isolated from 0.25 g intestinal samples (ileal mucus, ceca mucus, ceca content) via bead-beating using the DNeasy PowerSoil Kit (Qiagen). Extracted DNAs were assessed for quality using a NanoDrop 2000 spectrophotometer 260–280 nm ratios. Concentrations were determined using a Qubit fluorometer with the double-stranded DNA broad range kit (ThermoFisher Scientific) and adjusted to 50 ng/μL in nuclease-free water. Ileal content was initially-sampled, but insufficient DNA yield prevented further analyses. Library preparation was performed using the MiSeq and HiSeq2500 kit (Illumina) following all manufacturer’s instructions with 151 × 151 paired-end MiSeq sequencing (Illumina). DNAs were then sequenced via Illumina MiSeq (v3) at the Iowa State DNA facility. For 16S rRNA analysis, QIIME2 (version 2019.10) was used to analyze the 16S rRNA data between all sequenced groups. Sequences were demultiplexed using the QIIME2 demux emp-paired function and denoised using the QIIME2 plugin DADA2. The number of good quality reads for taxonomic assignment ranged from 37,495 to 114,115 reads. GreenGenes database (version 13.8) at the 99% operational taxonomic units (OTUs) spanning the V4 and V5 regions (515F, GTGYCAGCMGCCGCGGTAA; 926R, CCGYCAATTYMTTTRAGTTT) was used to classify each of the reads using QIIME2’s feature-classifier function. Alpha and beta diversity analyses were calculated using QIIME2’s built-in functions. Mmvec plugin (Morton et al., 2019) was used to explore taxonomic balances and taxonomic group differences between treatment groups. T tests were used to determine statistical significance between groups (see Section 2.10 for further details).
GraphPad Prism software version 6.0 (San Diego, CA) was used to calculate significance and construct figures for all analyses. Parametric, unpaired t-tests (two-tailed; α = 0.05) were used to compare differences between CON and VR groups. For bactericidal assays, where samples were pooled, assays were performed in triplicate per pool (n = 6 per treatment group). For 16S rRNA bacterial abundances and neurochemical data, differences were compared within sample site (ex: content, mucus, or tissue from ceca or ileum). For all statistical tests, data sets for each group were assumed to follow a normal distribution. p values <0.05 were considered significant.
General welfare assessment has shown no significant differences in body weight between control (1.15 kg ± 0.066) and VR (1.15 kg ± 0.063) groups (Supplementary Figure S1). Additionally, birds in both treatment groups exhibited no signs of injury from negative behaviors like pecking.
Using a blood bactericidal assay to test resistance to avian pathogenic E. coli (APEC), we found that blood from VR hens exhibited higher APEC killing to the three APEC serotypes O1, O2, and O78 tested compared to CON hens (p < 0.05; Figure 2A). This phenotype was positively-associated with an increase in serum corticosterone levels (p < 0.01; Figure 2B). However, H:L ratio was not significantly different between treatment groups (p > 0.05; Figure 2C).
FIGURE 2. Summary of data from chicken circulatory system (blood and plasma) (n = 15/group). Differences in E. coli blood bactericidal responses in vitro (A), plasma corticosterone levels (B), and heterophil:lymphocyte ratios (C) were evaluated between CON and VR hens. * p < 0.05; ** p < 0.01; *** p < 0.001.
Ultra-high pressure liquid chromatography (U-HPLC) data for neurochemicals and related metabolites in the content, mucus, and tissue of the chicken gut are summarized in Figure 3 (ileum) and Figure 4 (ceca). In the ileum, all detected neurochemicals were not significantly different in each site except for salsolinol (Figure 3C), which was higher in the content of CON hens (p < 0.0001) yet higher in mucus of VR hens (p < 0.01). Similarly, only salsolinol levels changed in the ceca between groups (Figure 4E), although differences were only seen in the tissue with VR hens having greater salsolinol levels (p < 0.05).
FIGURE 3. Neurochemical metabolite levels in chicken ileum (n = 15/group) measured by U-HPLC. (A), DOPAC. (B), dopamine. (C), salsolinol. (D), 5-HIAA. (E), serotonin. ** p < 0.01; **** p < 0.0001.
FIGURE 4. Neurochemical metabolite levels in chicken ceca (n = 15/group) measured by U-HPLC. (A), L-DOPA. (B), DOPAC. (C), dopamine. (D), norepinephrine. (E), salsolinol. (F), 5-HIAA. (G), serotonin.
Using 16S rRNA sequencing and QIIME2 analysis, microbiome alpha diversity (Faith PD, Pielou’s Evenness) was similar between groups (Supplementary Figure S2). Similarly, microbiome beta diversity was highly similar between groups at each intestinal site, although samples from the ileum mucus clustered distinctly along Axis 1 away from other intestinal samples in every beta diversity measure (Figures 5A–C, Weighted Unifrac; 5D-F, Unweighted UniFrac; 6A-C, Jaccard; 6D-E, Bray-Curtis). Looking at bacterial abundances, several taxa were consistently lower in VR hens compared to CON. For example, looking at ceca genera, Megamonas were lower in the mucus and content (p < 0.01), whereas Ruminococcus and Slackia were lower in content alone (p < 0.05; Figure 6A). Looking at the species level, Barnesiella viscericola and Blautia producta were reduced in the ceca mucus of VR hens (p < 0.05; Figure 6B). Uniquely, Mucispirillum schaedleri was the only taxon higher in VR hens, being greater in ileum mucus versus CON hens (p < 0.05; Figure 7B). Notably, these shifts did not have any impacts on short chain fatty acid (SCFA) production (Supplementary Figure S3).
FIGURE 5. Beta diversity plots of ceca microbiome from CON and VR birds (n = 15/group) via weighted UniFrac (A–C) and unweighted UniFrac (D–F) PCoA plots. Each sphere represents intestinal site from an individual bird, colored per respective group. Figures were generated by QIIME2 software. Yellow, CON ileum mucus. Green, VR ileum mucus. Bright red, CON ceca mucus. Bright blue, VR ceca mucus. Dark red, CON ceca content. Dark blue, VR ceca content.
FIGURE 6. Summary of bacterial taxonomic differences at genus (A) and species (B) levels between CON and VR birds (n = 15/group) via 16S rRNA sequencing. Yellow, CON ileum mucus. Green, VR ileum mucus. Bright red, CON ceca mucus. Bright blue, VR ceca mucus. Dark red, CON ceca content. Dark blue, VR ceca content. * p < 0.05; ** p < 0.01.
FIGURE 7. Beta diversity plots of ceca microbiome from CON and VR birds (n = 15/group) via weighted Bray-Curtis (A–C) and Jaccard (D–F) PCoA plots. Each sphere represents intestinal site from an individual bird, colored per respective group. Figures were generated by QIIME2 software. Yellow, CON ileum mucus. Green, VR ileum mucus. Bright red, CON ceca mucus. Bright blue, VR ceca mucus. Dark red, CON ceca content. Dark blue, VR ceca content.
To explore the possibility that specific bacterial taxa may be related to intestinal neurochemical synthesis, we used the QIIME2 plugin mmvec (Morton et al., 2019) to draw relationships between neurochemical and microbiome data from the ileum mucus, ceca mucus, and ceca content. Using an Emperor plot to display microbial or metabolite co-occurrences, two phyla co-occurrences (1: Firmicutes and Cyanobacteria; 2: Tenericutes, Deferribacteres, and Euryarchaeota) appear frequently along Axis 1 (Supplementary Figure S4A). These co-occurrences are amplified when plotted against Axis 3 (Supplementary Figure S4B) versus Axis 2 (Supplementary Figure S4A). Similarly, norepinephrine and homovillanic acid appear to frequently co-occur regardless of axes (Supplementary Figure S4). Lastly, to observe microbial-metabolite co-occurrences, inferred log conditional probabilities (LCPs) of microbial correlations with neurochemicals are displayed as a heatmap in Figure 8 and numerically summarized in Supplementary Table S1, in which positive probabilities are distinguished as red whereas low and negative values (i.e., light red to dark blue) indicate no relationship, not necessarily a negative relationship (see Github mmvec page: https://github.com/biocore/mmvec). L-DOPA and norepinephrine are positively related to Cyanobacteria (LCP = 1.52) and Euryarchaeota (LCP = 1.78), respectively. Dopamine and its waste metabolite salsolinol are positively related with Deferribacteres (LCP = 1.51 and 1.73, respectively) and Tenericutes (LCP = 1.69 and 1.71, respectively). Serotonin (5-HT) and its waste metabolite 5-hydroxyindoleacetic acid (5-HIAA) are positively related with Bacteroidetes (LCP = 3.19 and 2.05, respectively), Cyanobacteria (LCP = 3.43 and 2.33, respectively), Firmicutes (LCP = 3.30 and 2.63, respectively), and Proteobacteria (LCP = 2.74 and 2.07, respectively), with serotonin being specifically related with Spirochaetes (LCP = 1.71) and Tenericutes (LCP = 1.72). Uniquely, homovillanic acid and DOPAC are not related to any bacterial taxa at the phylum level.
FIGURE 8. Heatmap output of QIIME2 mmvec plugin analyses of microbe-metabolite co-occurrences. Darker shades of red indicate higher levels of co-occurrences between bacterial phyla and neurochemical metabolites.
In this study, we tested whether VR video in chickens’ pens could improve hens’ welfare and health. Five-day-exposure to a VR video of free-range hens induced changes in several biological parameters in onset-lay hens. Given that layer hens at this stage (15 weeks post-hatch) are moved from pullet to egg-laying facilities and are plausibly at greater risk for stress (Janczak and Riber, 2015), we used this period to model how VR could be implemented in a commercial practice. Previous studies have demonstrated a positive correlation between an exposure to a video image of conspecific animals with increased food intake and positive social behaviors in chickens (Keeling and Hurnik, 1993). However, our study did not find an effect of a short exposure period to VR on chickens’ body weight, as both VR and control had the same body weight (∼1.15 kg) and similar levels of stress-related catecholamines like norepinephrine and epinephrine. Furthermore, although corticosterone level was higher in VR hens, corticosterone concentrations from both groups, ranging from <1 to nearly 6 ng corticosterone/ml serum, were in-line for what has been observed in unstressed hens, which have been reported to exceed over 20 ng corticosterone/ml serum (Scanes, 2016). Thus, it appears our pen design itself may have minimized stress in both animal groups. The little effect of VR on chickens could be due to the short duration of exposure (5 days) to VR videos. Previous work has shown that the interest to the video image by experimental birds is gradual. At Day 1, hens avoid looking at the screen, whereas hens demonstrate an increased interest by Day 8 (Clark and Jones, 2001). Future studies should test longer exposure time to VR and consider the incorporation of our VR construct with hens from different stages (i.e., pullet stage) to determine if VR exposure is more influential at certain ages.
Reducing stress could also affect immunological responses against bacterial pathogens like APEC (Mellata, 2013). Given the interconnectedness between the animal brain, gut, and its gut microbiota (Clarke et al., 2013; Yano et al., 2015; Valles-Colomer et al., 2019), we hypothesized that VR stimuli may modulate neurochemical metabolism and microbiota composition in the gut. Most VR studies focus on behavioral and neuroscience changes (Bohil et al., 2011); therefore, we focused on how VR affected the immune system and microbiota of hens. One notable finding was that on the fifth 5 day of VR exposure, blood from VR hens had increased killing of APEC in vitro compared to that of control birds. Furthermore, this observation was positively-associated with an increase in serum corticosterone. Although high glucocorticoid concentrations (i.e., > 9 ng/ml) induce immunosuppression (Davison et al., 1988; El-Lethey et al., 2003; Shini et al., 2010), acute exposure to moderate levels of cortisol (5 ng/ml) in bovine endometrial epithelial cells increase inflammatory responses against E. coli when compared to cells not conditioned with cortisol (Cui et al., 2020). Thus, the moderate increase in corticosterone could have primed immune cells to allow a greater APEC-bactericidal response. Since our VR model only lasted for 5 days, it is not clear if continued exposure to these visual stimuli would result in chronically-elevated corticosterone, which might have deleterious effects on immune function (Coutinho and Chapman, 2011).
To our knowledge, no study has investigated whether exposure to VR can alter the gut microbiota. Here, almost every taxon with significantly-changed relative abundances were higher in CON versus VR hens, regardless of intestinal site. Many of these taxa (ex: Megamonas, Blautia, and Ruminococcus) are major producers of SCFAs (Takahashi et al., 2016; Louis and Flint, 2017; Zhao et al., 2017), which confer numerous benefits such as pathogen exclusion and immune activation (Parada Venegas et al., 2019). However, despite these microbiome changes, we did not identify any changes in SCFAs in any intestinal site. Thus, it appears that changes in these SCFA-producing populations did not have a functional impact on VR hens in terms of SCFA production at the time point tested. Additionally, a single taxon, M. schaedleri, was higher in the ileal mucus of VR hens relative to CON. M. schaedleri, the sole member of the Deferribacteres phylum detected in this study, is an obligate anaerobic bacterium, which colonizes (but does not degrades) host mucus (Loy et al., 2017) and is associated with several inflammatory pathologies in mammals (Loy et al., 2017) and fat deposition in poultry (Wen et al., 2019). At this time, it is unclear how VR treatment increases M. schaedleri abundances in the ileum. However, we did observe a strong, positive relationship between Deferribacteres (i.e., M. schaedleri) and salsolinol-dopamine in the intestine. Salsolinol is a dopaminergic waste metabolite (Yamanaka et al., 1970), which has gained recent attention given its role in Parkinson’s disease in mammals (Naoi et al., 2002). Altogether, these findings suggest that M. schaedleri may either produce salsolinol directly [as demonstrated by other microbes (Villageliú et al., 2018a)] or positively-responds to its presence in the intestine. However, the changes to both M. schaedleri and salsolinol production are driven by VR exposure, and future studies will investigate the mechanisms in which these visual stimuli drive such changes in the chicken intestine.
In addition to the relationship between Deferribacteres and salsolinol-dopamine, we identified several other positive relationships between microbial phyla and neurochemicals. We measured these relationships using co-occurrence probabilities (i.e., the QIIME2 mmvec plugin) versus correlative measures like Pearson’s, which are too simplistic for compositional data due to high false-discovery rates (Morton et al., 2019). Similar to Deferribacteres, Tenericutes abundances exhibited high relatedness with salsolinol and dopamine, suggesting that members of these two phyla may collaborate to directly or indirectly facilitate dopamine-metabolism to salsolinol. Notably, we also identified several phyla (Bacteriodetes, Cyanobacteria, Firmicutes, Proteobacteria, Spirochaetes, and Tenericutes) positively-related to serotonin in the chicken intestine. In mice, spore-forming gut microbes are crucial for host serotonin synthesis in the gut (Yano et al., 2015), the source of roughly 95% of the body’s total serotonin (Gershon and Tack, 2007). Given that several Firmicutes form endospores (Galperin, 2013) and the positive relationship between Firmicutes and serotonin in our study, we speculate a similar relationship may exist in chickens. However, it is unclear whether these microbes are directly producing these neurochemicals (Lyte, 2014), influencing host neurochemical synthesis (Yano et al., 2015), or are responding to these neurochemicals in the gut via activities like virulence gene expression (Freestone et al., 2008).
In conclusion, we found host resistance to E. coli, corsticosterone levels, neurochemical metabolism, and gut microbiota composition were all affected by VR exposure in laying hens. Importantly, this study is the first to demonstrate that VR exposure can modulate the gut microbiota, which was related to the production of several neurochemicals and associated metabolites in the gut. Current work is underway to look at whether more-complex animal behaviors were either affected by VR in general or if birds were particularly-responsive to certain scenes in these VR videos.
The datasets presented in this study can be found in online repositories. The names of the repository/repositories and accession number(s) can be found below: https://www.ncbi.nlm.nih.gov/, PRJNA638088.
The animal study was reviewed and approved by Iowa State University Institutional Animal Care and Use Committee, log #19-211. Written informed consent was obtained from the owners for the participation of their animals in this study.
MM and JO conceived the project; GR, SM, RR, ML, JO, and MM designed the experiments. GR, RP, AH, KD, and MM performed the experiments and analyzed the data. GR and MM wrote the manuscript. SM, ML, and JO revised the manuscript. MM, SM, RR, and ML contributed reagents, materials, and analysis tools. All authors read and approved the final manuscript.
This research was supported by Iowa State University Presidential Interdisciplinary Research Seed Grant (PIRS) to MM, ML, JO, and SM. Start-up funding and the United States Department of Agriculture (USDA) Hatch project IOW03902 to MM, USDA-National Institute of Food and Agriculture (NIFA) project #021069-00001 to GR.
GR, SM, RR, ML, JO, and MM are listed as inventors on a pending patent covering research presented in this paper.
The remaining authors declare that the research was conducted in the absence of any commercial or financial relationships that could be construed as a potential conflict of interest.
All claims expressed in this article are solely those of the authors and do not necessarily represent those of their affiliated organizations, or those of the publisher, the editors and the reviewers. Any product that may be evaluated in this article, or claim that may be made by its manufacturer, is not guaranteed or endorsed by the publisher.
The authors thank Sasha Celada, Jared Jochum, Logan Ott (MM students), Samantha Wilson (SM student), and the Genome Informatics Facility (Iowa State University) for technical assistance in this study. The authors would also like to thank Iowa Cagefree LLP for donating the hens used in this study and the local backyard producers for allowing us to videorecord their birds.
The Supplementary Material for this article can be found online at: https://www.frontiersin.org/articles/10.3389/frvir.2022.891584/full#supplementary-material
Barbieri, N. L., Oliveira, A. L. d., Tejkowski, T. M., Pavanelo, D. B., Rocha, D. A., Matter, L. B., et al. (2013). Genotypes and Pathogenicity of Cellulitis Isolates Reveal Traits that Modulate APEC Virulence. PLoS One 8 (8), e72322. doi:10.1371/journal.pone.0072322
Bestman, M., and Bikker-Ouwejan, J. (2020). Predation in Organic and Free-Range Egg Production. Animals 10, 177. doi:10.3390/ani10020177
Bohil, C. J., Alicea, B., and Biocca, F. A. (2011). Virtual Reality in Neuroscience Research and Therapy. Nat. Rev. Neurosci. 12 (12), 752–762. doi:10.1038/nrn3122
Broom, D. M. (1969). Reactions of Chicks to Visual Changes during the First Ten Days after Hatching. Anim. Behav. 17 (2), 307–315. doi:10.1016/0003-3472(69)90015-3
Clarke, C. H., and Jones, R. B. (1999). Does Video Stimulation Have an Important Role to Play in Poultry Behaviour Research? Br. Poult. Sci. 40 (Suppl. l), 8–9. doi:10.1080/00071669986530
Clarke, C. H., and Jones, R. B. (2001). Domestic Chicks' Runway Responses to Video Images of Conspecifics. Appl. Animal Behav. Sci. 70 (4), 285–295. doi:10.1016/s0168-1591(00)00161-1
Clarke, G., Grenham, S., Scully, P., Fitzgerald, P., Moloney, R. D., Shanahan, F., et al. (2013). The Microbiome-Gut-Brain axis during Early Life Regulates the Hippocampal Serotonergic System in a Sex-dependent Manner. Mol. Psychiatry 18 (6), 666–673. doi:10.1038/mp.2012.77
Coutinho, A. E., and Chapman, K. E. (2011). The Anti-inflammatory and Immunosuppressive Effects of Glucocorticoids, Recent Developments and Mechanistic Insights. Mol. Cell. Endocrinol. 335 (1), 2–13. doi:10.1016/j.mce.2010.04.005
Cui, L., Wang, Y., Wang, H., Dong, J., Li, Z., Li, J., et al. (2020). Different Effects of Cortisol on Pro-inflammatory Gene Expressions in LPS-, Heat-Killed E.coli-, or Live E.coli-Stimulated Bovine Endometrial Epithelial Cells. BMC Vet. Res. 16, 1–9. doi:10.1186/s12917-020-2231-z
Davison, T. F., Misson, B. H., Williamson, R. A., and Rea, J. (1988). Effect of Increased Circulating Corticosterone in the Immature Fowl on the Blastogenic Responses of Peripheral Blood Lymphocytes. Dev. Comp. Immunol. 12 (1), 131–144. doi:10.1016/0145-305x(88)90031-6
Dawkins, M. S., and Woodington, A. (2000). Pattern Recognition and Active Vision in Chickens. Nature 403 (6770), 652–655. doi:10.1038/35001064
de Macchi, B. M., Miranda, F. J. B., de Souza, F. S., de Carvalho, E. C. Q., Albernaz, A. P., do Nascimento, J. L. M., et al. (2013). Chickens Treated with a Nitric Oxide Inhibitor Became More Resistant to Plasmodium Gallinaceum Infection Due to Reduced Anemia, Thrombocytopenia and Inflammation. Vet. Res. 44, 8. doi:10.1186/1297-9716-44-8
El-Lethey, H., Huber-Eicher, B., and Jungi, T. W. (2003). Exploration of Stress-Induced Immunosuppression in Chickens Reveals Both Stress-Resistant and Stress-Susceptible Antigen Responses. Vet. Immunol. Immunopathol. 95 (3-4), 91–101. doi:10.1016/s0165-2427(02)00308-2
Freestone, P. P. E., Sandrini, S. M., Haigh, R. D., and Lyte, M. (2008). Microbial Endocrinology: How Stress Influences Susceptibility to Infection. Trends Microbiol. 16 (2), 55–64. doi:10.1016/j.tim.2007.11.005
Galperin, M. Y. (2013). Genome Diversity of Spore-Forming Firmicutes. Microbiol. Spectr. 1, 2. doi:10.1128/microbiolspectrum.TBS-0015-2012
Gershon, M. D., and Tack, J. (2007). The Serotonin Signaling System: from Basic Understanding to Drug Development for Functional GI Disorders. Gastroenterology 132 (1), 397–414. doi:10.1053/j.gastro.2006.11.002
Gross, W. B., and Siegel, H. S. (1983). Evaluation of the Heterophil/lymphocyte Ratio as a Measure of Stress in Chickens. Avian Dis. 27 (4), 972–979. doi:10.2307/1590198
Heerkens, J. L. T., Delezie, E., Kempen, I., Zoons, J., Ampe, B., Rodenburg, T. B., et al. (2015). Specific Characteristics of the Aviary Housing System Affect Plumage Condition, Mortality and Production in Laying Hens. Poult. Sci. 94 (9), 2008–2017. doi:10.3382/ps/pev187
Janczak, A. M., and Riber, A. B. (2015). Review of Rearing-Related Factors Affecting the Welfare of Laying Hens. Poult. Sci. 94 (7), 1454–1469. doi:10.3382/ps/pev123
Johnson, T. J., Kariyawasam, S., Wannemuehler, Y., Mangiamele, P., Johnson, S. J., Doetkott, C., et al. (2007). The Genome Sequence of Avian Pathogenic Escherichia coli Strain O1:K1:H7 Shares Strong Similarities with Human Extraintestinal Pathogenic E. coli Genomes. J. Bacteriol. 189 (8), 3228–3236. doi:10.1128/JB.01726-06
Johnson, T. J., Siek, K. E., Johnson, S. J., and Nolan, L. K. (2006). DNA Sequence of a ColV Plasmid and Prevalence of Selected Plasmid-Encoded Virulence Genes Among Avian Escherichia coli Strains. J. Bacteriol. 188 (2), 745–758. doi:10.1128/JB.188.2.745-758.2006
Keeling, L. J., and Hurnik, J. F. (1993). Chickens Show Socially Facilitated Feeding Behaviour in Response to a Video Image of a Conspecific. Appl. Anim. Behav. Sci. 36 (2-3), 223–231. doi:10.1016/0168-1591(93)90012-E
Lien, R. J., Hess, J. B., McKee, S. R., and Bilgili, S. F. (2008). Effect of Light Intensity on Live Performance and Processing Characteristics of Broilers. Poult. Sci. 87 (5), 853–857. doi:10.3382/ps.2007-00277
Louis, P., and Flint, H. J. (2017). Formation of Propionate and Butyrate by the Human Colonic Microbiota. Environ. Microbiol. 19 (1), 29–41. doi:10.1111/1462-2920.13589
Loy, A., Pfann, C., Steinberger, M., Hanson, B., Herp, S., Brugiroux, S., et al. (2017). Lifestyle and Horizontal Gene Transfer-Mediated Evolution of Mucispirillum Schaedleri, a Core Member of the Murine Gut Microbiota. mSystems 2, 1. doi:10.1128/mSystems.00171-16
Lyte, M. (2014). Microbial Endocrinology and the Microbiota-Gut-Brain axis. Adv. Exp. Med. Biol. 817, 3–24. doi:10.1007/978-1-4939-0897-4_1
Maier, M., Rubio Ballester, B., Duff, A., Duarte Oller, E., and Verschure, P. F. M. J. (2019). Effect of Specific over Nonspecific VR-Based Rehabilitation on Poststroke Motor Recovery: A Systematic Meta-Analysis. Neurorehabil. Neural. Repair 33 (2), 112–129. doi:10.1177/1545968318820169
Maples-Keller, J. L., Yasinski, C., Manjin, N., and Rothbaum, B. O. (2017). Virtual Reality-Enhanced Extinction of Phobias and Post-Traumatic Stress. Neurotherapeutics 14 (3), 554–563. doi:10.1007/s13311-017-0534-y
Mellata, M. (2013). Human and Avian Extraintestinal PathogenicEscherichia Coli: Infections, Zoonotic Risks, and Antibiotic Resistance Trends. Foodborne Pathogens Dis. 1011, 916–932. doi:10.1089/fpd.2013.1533
Morton, J. T., Aksenov, A. A., Nothias, L. F., Foulds, J. R., Quinn, R. A., Badri, M. H., et al. (2019). Learning Representations of Microbe-Metabolite Interactions. Nat. Methods 16 (12), 1306–1314. doi:10.1038/s41592-019-0616-3
Naoi, M., Maruyama, W., Akao, Y., and Yi, H. (2002). Dopamine-derived Endogenous N-methyl-(R)-salsolinol. Neurotoxicology Teratol. 24 (5), 579–591. doi:10.1016/s0892-0362(02)00211-8
Oliveira, J. L., Xin, H., Chai, L., and Millman, S. T. (2019). Effects of Litter Floor Access and Inclusion of Experienced Hens in Aviary Housing on Floor Eggs, Litter Condition, Air Quality, and Hen Welfare. Poult. Sci. 98 (4), 1664–1677. doi:10.3382/ps/pey525
Parada Venegas, D., De la Fuente, M. K., Landskron, G., González, M. J., Quera, R., Dijkstra, G., et al. (2019). Short Chain Fatty Acids (SCFAs)-Mediated Gut Epithelial and Immune Regulation and its Relevance for Inflammatory Bowel Diseases. Front. Immunol. 10, 277. doi:10.3389/fimmu.2019.00277
Scanes, C. G. (2016). Biology of Stress in Poultry with Emphasis on Glucocorticoids and the Heterophil to Lymphocyte Ratio. Poult. Sci. 95 (9), 2208–2215. doi:10.3382/ps/pew137
Shini, S., Huff, G. R., Shini, A., and Kaiser, P. (2010). Understanding Stress-Induced Immunosuppression: Exploration of Cytokine and Chemokine Gene Profiles in Chicken Peripheral Leukocytes. Poult. Sci. 89 (4), 841–851. doi:10.3382/ps.2009-00483
Singh, M., Ruhnke, I., de Koning, C., Drake, K., Skerman, A. G., Hinch, G. N., et al. (2017). Demographics and Practices of Semi-intensive Free-Range Farming Systems in Australia with an Outdoor Stocking Density of ≤1500 Hens/hectare. PLoS One 12 (10), e0187057. doi:10.1371/journal.pone.0187057
Stacy, A. K., Mitchell, N. M., Maddux, J. T., De la Cruz, M. A., Durán, L., Girón, J. A., et al. (2014). Evaluation of the Prevalence and Production of Escherichia coli Common Pilus Among Avian Pathogenic E. coli and its Role in Virulence. PLoS One 9 (1), e86565. doi:10.1371/journal.pone.0086565
Stinson, C., and Bowman, D. A. (2014). Feasibility of Training Athletes for High-Pressure Situations Using Virtual Reality. IEEE Trans. Vis. Comput. Graph. 20 (4), 606–615. doi:10.1109/TVCG.2014.23
Stowers, J. R., Hofbauer, M., Bastien, R., Griessner, J., Higgins, P., Farooqui, S., et al. (2017). Virtual Reality for Freely Moving Animals. Nat. Methods 14 (10), 995–1002. doi:10.1038/nmeth.4399
Takahashi, K., Nishida, A., Fujimoto, T., Fujii, M., Shioya, M., Imaeda, H., et al. (2016). Reduced Abundance of Butyrate-Producing Bacteria Species in the Fecal Microbial Community in Crohn's Disease. Digestion 93 (1), 59–65. doi:10.1159/000441768
Valles-Colomer, M., Falony, G., Darzi, Y., Tigchelaar, E. F., Wang, J., Tito, R. Y., et al. (2019). The Neuroactive Potential of the Human Gut Microbiota in Quality of Life and Depression. Nat. Microbiol. 4 (4), 623–632. doi:10.1038/s41564-018-0337-x
Villageliú, D. N., Borts, D. J., and Lyte, M. (2018a). Production of the Neurotoxin Salsolinol by a Gut-Associated Bacterium and its Modulation by Alcohol. Front. Microbiol. 9, 3092. doi:10.3389/fmicb.2018.03092
Villageliú, D. N., Rasmussen, S., and Lyte, M. (2018b). A Microbial Endocrinology-Based Simulated Small Intestinal Medium for the Evaluation of Neurochemical Production by Gut Microbiota. FEMS Microbiol. Ecol. 94, 7. doi:10.1093/femsec/fiy096
Wen, C., Yan, W., Sun, C., Ji, C., Zhou, Q., Zhang, D., et al. (2019). The Gut Microbiota Is Largely Independent of Host Genetics in Regulating Fat Deposition in Chickens. ISME J. 13 (6), 1422–1436. doi:10.1038/s41396-019-0367-2
Yamanaka, Y., Walsh, M. J., and Davis, V. E. (1970). Salsolinol, an Alkaloid Derivative of Dopamine Formed In Vitro during Alcohol Metabolism. Nature 227 (5263), 1143–1144. doi:10.1038/2271143a0
Yang, Y. J. D., Allen, T., Abdullahi, S. M., Pelphrey, K. A., Volkmar, F. R., and Chapman, S. B. (2018). Neural Mechanisms of Behavioral Change in Young Adults with High-Functioning Autism Receiving Virtual Reality Social Cognition Training: A Pilot Study. Autism Res. 11 (5), 713–725. doi:10.1002/aur.1941
Yano, J. M., Yu, K., Donaldson, G. P., Shastri, G. G., Ann, P., Ma, L., et al. (2015). Indigenous Bacteria from the Gut Microbiota Regulate Host Serotonin Biosynthesis. Cell. 161 (2), 264–276. doi:10.1016/j.cell.2015.02.047
Zhao, J., Nian, L., Kwok, L. Y., Sun, T., and Zhao, J. (2017). Reduction in Fecal Microbiota Diversity and Short-Chain Fatty Acid Producers in Methicillin-Resistant Staphylococcus aureus Infected Individuals as Revealed by PacBio Single Molecule, Real-Time Sequencing Technology. Eur. J. Clin. Microbiol. Infect. Dis. 36 (8), 1463–1472. doi:10.1007/s10096-017-2955-2
Keywords: virtual reality, chickens, corticosterone, neurochemicals, gut microbiota, APEC, salsolinol
Citation: Redweik GAJ, Millman ST, Parsons RL, Hurtado Terminel AN, Radkowski R, Daniels K, Lyte M, Oliver J and Mellata M (2022) Exposure to a Virtual Environment Induces Biological and Microbiota Changes in Onset-of-Lay Hens. Front. Virtual Real. 3:891584. doi: 10.3389/frvir.2022.891584
Received: 07 March 2022; Accepted: 19 May 2022;
Published: 02 June 2022.
Edited by:
Dioselin Gonzalez, Independent researcher, Seattle, WA, United StatesReviewed by:
Fangshen Guo, China Agricultural University, ChinaCopyright © 2022 Redweik, Millman, Parsons, Hurtado Terminel, Radkowski, Daniels, Lyte, Oliver and Mellata. This is an open-access article distributed under the terms of the Creative Commons Attribution License (CC BY). The use, distribution or reproduction in other forums is permitted, provided the original author(s) and the copyright owner(s) are credited and that the original publication in this journal is cited, in accordance with accepted academic practice. No use, distribution or reproduction is permitted which does not comply with these terms.
*Correspondence: Melha Mellata, bW1lbGxhdGFAaWFzdGF0ZS5lZHU=
Disclaimer: All claims expressed in this article are solely those of the authors and do not necessarily represent those of their affiliated organizations, or those of the publisher, the editors and the reviewers. Any product that may be evaluated in this article or claim that may be made by its manufacturer is not guaranteed or endorsed by the publisher.
Research integrity at Frontiers
Learn more about the work of our research integrity team to safeguard the quality of each article we publish.