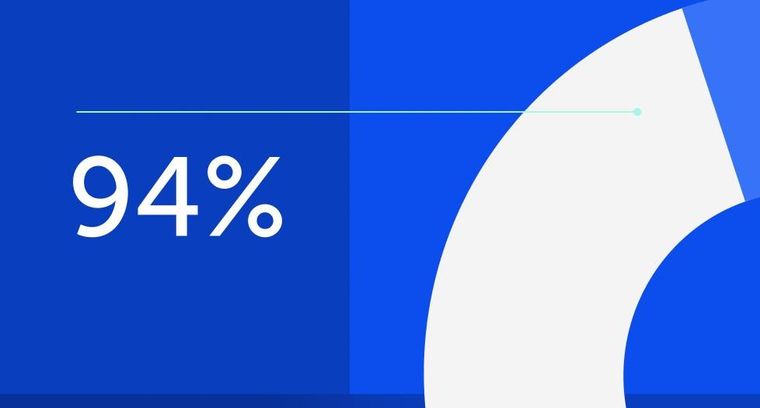
94% of researchers rate our articles as excellent or good
Learn more about the work of our research integrity team to safeguard the quality of each article we publish.
Find out more
ORIGINAL RESEARCH article
Front. Virtual Real., 20 December 2021
Sec. Virtual Reality and Human Behaviour
Volume 2 - 2021 | https://doi.org/10.3389/frvir.2021.761142
The use of virtual reality (VR) promises enormous potential for studying human behavior. While approach and avoidance tendencies have been explored in various areas of basic and applied psychology, such as attitude and emotion research, basic learning psychology, and behavior therapy, they have rarely been studied in VR. One major focus of this research is to understand the psychological mechanisms underlying automatic behavioral tendencies towards and away from positively or negatively evaluated stimuli. We implemented a whole-body movement stimulus-response compatibility task to explore approach-avoidance behavior in an immersive virtual environment. We chose attitudinal stimuli—spiders and butterflies—on which people widely agree in their general evaluations (in that people evaluate spiders negatively and butterflies positively), while there is still substantial inter-individual variance (i. e., the intensity in which people dislike spiders or like butterflies). We implemented two parallel approach-avoidance tasks, one in VR, one desktop-based. Both tasks revealed the expected compatibility effects that were positively intercorrelated. Interestingly, however, the compatibility effect in the VR measure was unrelated to participants’ self-reported fear of spiders and stimulus evaluations. These results raise important implications about the usage of VR to study automatic behavioral tendencies.
One central goal of psychological research is to understand real-life human behavior. However, psychologists have to examine behavior under highly controlled conditions with only a few manipulations at a time in order to obtain scientifically reliable results. Hence, researchers face a dilemma between experimental control and ecological validity. Virtual reality (VR) provides a promising testbed to support researchers from computer science and psychology exploring human nature in an interdisciplinary way. Immersive virtual environments (IVEs) can be superior to traditional experimental settings in terms of both visual fidelity and natural interaction patterns, which can eventually evoke a sense of presence (Slater and Sanchez-Vives, 2016). The degree of elicited presence depends on various factors, including the user’s individual psychological traits (Oh et al., 2018) as well as the VR system’s technical capability to deliver a realistic, convincing environment (i.e., its immersion; Sanchez-Vives and Slater, 2005). While there is an ongoing discussion about the realism of elicited psychological responses in IVEs (Wilson and Soranzo, 2015), the beneficial attributes of VR for behavioral research could be demonstrated in numerous previous research projects, for example, for treating anxiety disorders (Opriş et al., 2012) and for studying the automatic assessment of the valence of environmental stimuli (Mostajeran et al., 2019).
In this article, we present an IVE to explore approach and avoidance responses, that is behavioral responses that are intended to decrease (i.e., approach) or increase (i.e., avoid) the spacial distance between the self and an attitude object. These behavioral responses can be considered a component of attitudes, defined as the psychological tendency to evaluate attitude objects (things, persons, situations, ideas) with some degree of favor or disfavor (e.g., Eagly and Chaiken, 1993). Approach and avoidance behaviors are thus based on the powerful human cognitive-affective mechanisms that allow us to quickly and efficiently avoid unpleasant or dangerous situations and approach desired rewards or pleasant situations (see Krieglmeyer et al., 2013). Most theories assume that the adaptive advantage of these mechanisms lies in their automaticity, that is, the spontaneous, fast, and efficient operation that immediately translates evaluations and emotional responses into behavior without relying on time- and resource-consuming deliberate intentions or conscious decision making, and without even requiring the availability of cognitive resources or control (Moors and De Houwer, 2006). While not all sensorimotor aspects of human behavior can be fully simulated in an IVE, there is positive evidence that particularly these automatic responses occur in virtual environments similar to how they do in the real world (Slater and Sanchez-Vives, 2016).
Because actual observation or measurement of automatic aspects of behavior can be difficult to implement in laboratory research, experimental studies often use rather indirect behavioral measures. These typically rely on artificial measurement apparatuses for assessing arm or hand movements (e. g., levers, joysticks, touchpads) or desktop-based approaches that measure the response times for pressing buttons or moving manikins as indirect indicators of automatic behavioral decisions of approach and avoidance. Albeit this research has provided important empirical evidence and instigated intense theorizing and debate on the underlying psychological mechanisms of approach and avoidance behaviors (for an overview see Krieglmeyer et al., 2013), the employed measures also have a number of limitations. For example, the previously used measures have provided mixed evidence with regard to ecological validity and predictive values of attitude expressions and/or real-life behavior towards attitude objects (e.g., Degner et al., 2016; Rougier et al., 2020). The use of VR and the measurement of real body movements in virtual environments offer a potential improvement to this field of research. Therefore, however, we need to rely on basic research that investigates if and to what extent such experimental procedures in virtual environments actually represent aspects of automatic behavior transferable to the real world.
We conducted a study to compare approach-avoidance measurements between an IVE and a desktop-based environment, the Visual Approach/Avoidance by the Self Task (VAAST; Rougier et al., 2018). In both environments, the study participants performed comparable tasks involving the same stimuli and identical task parameters. Furthermore, in both tasks, the impression of a whole-body movement was conveyed through changes in the visual flow. A key difference between the conditions was the response type. In the desktop-based VAAST, participants responded by pressing keys to represent approach vs. avoidance decisions. In the VR task, they performed actual full-body movements, i.e., taking one step towards or away from the stimuli. In both tasks, responses triggered comparable visual flows—either on the computer monitor or in the virtual environment—simulating visual impressions of own forward and backward body movements. In the presented study, we chose spiders and butterflies as attitudinal stimuli, because people commonly agree on their negative and positive valence most people dislike spiders and like butterflies. Nevertheless, there are reliable differences documented in the intensity of these attitudes, at least of peoples’ negative evaluation of spiders (Rinck and Becker, 2007). All participants in our sample completed an approach-avoidance task in both the VR- and the desktop-based environments along with several self-report measures of attitudes and approach-avoidance tendencies, with a focus on the fear of spiders. Our goal was to explore (1) whether both environments were effective in revealing approach-avoidance compatibility effects, defined as response facilitation of spider-avoidance and butterfly-approach and inhibition of spider-approach and butterfly-avoidance movements and (2) if and to what extent the effects were related to each other as well as to the participants’ self-reported ratings and behavioral tendencies.
The remainder of this article is structured as follows. Section 2 provides details on the psychological fundamentals of approach-avoidance behaviors as well as an overview of traditional and VR-based research of approach-avoidance tendencies. In Section 3, we introduce the performed study, including the experimental materials and methods. Section 4 describes the results of the study, which are discussed in Section 5. Finally, Section 6 concludes the article and provides an outlook on future work.
In the following, we will introduce fundamental terms in the context of approach-avoidance research before summarizing related studies on approach-avoidance tendencies, both using traditional experimental setups and IVEs.
Approach and avoidance tendencies have been explored in various basic and applied areas of psychology, such as emotion research, attitude research, neuropsychology, and behavior therapy (e.g., Rinck and Becker, 2007; Beatty et al., 2016; Mostajeran et al., 2019). A vast number of studies have demonstrated that people automatically assess the valence of environmental stimuli (e.g., Zajonc, 1980; Fazio et al., 1986; Wentura and Degner, 2010) and that these spontaneous evaluations are related to facilitation or inhibition of approach and avoidance behaviors (see Krieglmeyer et al., 2013, for an overview). For the investigation of such tendencies, a variety of so-called stimulus-response compatibility tasks has been developed. In such tasks, the participants are presented successively with stimuli of different valence to which they should react as quickly and accurately as possible with approach or avoidance behavior. The reaction time is usually measured as a dependent variable (e.g., Phaf et al., 2014). Accordingly, stimulus-response combinations can be divided into compatible and incompatible conditions: Approaching movements for positively evaluated stimuli as well as avoidance behavior for negatively evaluated stimuli are considered compatible stimulus-response combinations. In contrast, avoidance behavior for positively classified stimuli and approach behavior to negatively classified stimuli are considered incompatible (Phaf et al., 2014). Concerning response times, the initiation of compatible behavior is usually faster than for incompatible behavior (Krieglmeyer and Deutsch, 2010). However, the exact cognitive, affective, and embodied mechanisms that are involved in this decision and behavior initiation process are still actively researched and debated.
First demonstrations of approach-avoidance compatibility effects stem from a seminal study by Solarz (1960), in which participants had to respond to cards showing positive and negative words by pushing them away or pulling them towards themselves using a hand lever. They responded with faster push movements for cards with negative words rather than positive words and, vice versa, with faster pull movements for cards with positive rather than negative words (Solarz, 1960). In the last 3 decades, a variety of computerized measures have been proposed that allow a more precise and efficient investigation of such automatic behavioral effects. For example, there are several behavioral paradigms in which participants push and pull lever constructions (e.g., Chen and Bargh, 1999; Rotteveel et al., 2015) or a joystick (e.g., Rinck and Becker, 2007) in response to positive and negative stimuli presented on a computer monitor. Observed compatibility effects in these paradigms have often been explained in terms of embodied or grounded cognition and direct affect-motor links (e.g., Barsalou, 2008; Barsalou, 2015). However, concerns have been raised since there is no one-on-one mapping between specific muscle activation and approach or avoidance behavior. For example, the same arm muscle extensions occur both when pushing away an undesired object as well as when stretching an arm to grab a desired object (e.g., Eder and Rothermund, 2008).
In other applications, a variety of computerized tasks have been developed with the aim of achieving unambiguous interpretability of behavioral tendencies representing approach and avoidance. Most of them do not require motor responses such as push and pull movements but rely on keypresses symbolizing approach and avoidance decisions instead. Therefore, these tasks often include some sort of visual response feedback signaling behavioral consequences of approach or avoidance, such as an increasing stimulus size after approach responses and decreasing stimulus size after avoidance responses (Seibt et al., 2008), or showing a manikin figure moving towards or away from the stimuli (De Houwer et al., 2001). In this context, Rougier and colleagues implemented a desktop-based pseudo-3D environment that simulated visual feedback of whole-body movements towards and away from target stimuli (Rougier et al., 2018). In the presented Visual Approach/Avoidance by the Self Task, short VAAST, participants responded to the displayed stimuli by pressing vertically arranged keys. As a result, the size of the stimuli as well as their background image changed, creating the visual impression of a forward or backward whole-body movement. Evaluating this task in a series of experiments, Rougier et al. demonstrated large and replicable compatibility effects outperforming other desktop-based task procedures.
While the VAAST simulates the visual flow which would be experienced during a whole-body movement, the study participants remain seated in front of a computer monitor during the entire procedure. However, it is not only the distance regulation itself that plays a key role in the activation of approach-avoidance behavior but also the type of regulation (Krieglmeyer and Deutsch, 2010). In this regard, we can differentiate between movements of the stimulus itself and the person who is observing the stimulus. We assume that a measure relying on actual movements of the self is more closely related the sensori-motor experiences of real-life behavior and thus more closely related to spontaneous and automatic behavioral regulation in real-life (Krieglmeyer and Deutsch, 2010; Rougier et al., 2018). Therefore, IVEs could be beneficial to observe realistic approach-avoidance behavior since users can respond to stimuli via actual whole-body movements. In the next section, we will consider further advantages of VR setups for experimental psychological research and discuss related research projects in the context of approach-avoidance behavior.
VR technologies allow users to immerse themselves in computer-generated environments. For this purpose, users can use different forms of head-mounted displays (HMDs) or immersive projection systems (CAVE) typically combined with a tracking system, which monitors the user’s movements and maps them to the virtual environment. For instance, HMD users can move their head to spontaneously and naturally explore the virtual environment. Using VR technology promises a high value for the scientific research of underlying psychological mechanisms of approach and avoidance tendencies under experimentally controlled conditions (Wilson and Soranzo, 2015). The ability to create visually realistic three-dimensional scenes and to measure responses to the visual stimuli enables behavioral researchers to test hypotheses in a manner and scale that were previously unfeasible. Furthermore, studies in VR can be conducted when behavioral observations are difficult to obtain (e.g., rare stimuli, specific social groups) or even ethically questionable (e.g., stimuli that pose a danger or are associated with intense emotional responses). Concerning approach-avoidance tasks, another beneficial characteristic of VR technology is that users can estimate distances to virtual objects by processing binocular cues, such as disparity, as well as oculomotor cues, such as convergence. In real environments, disparity and convergence are two of the most accurate depth cues in personal space (El Jamiy and Marsh, 2019), outperforming cues such as stimulus size, which is used to simulate distance changes in many of the desktop-based approach-avoidance tasks presented in Traditional Approach-Avoidance Tasks. It has to be pointed out, though, that many VR studies reported a tendency towards depth underestimation in head-mounted displays (HMDs; El Jamiy and Marsh, 2019).
The potential advantages of VR in behavioral research have been recognized for at least 2 decades (Loomis et al., 1999), but recent advantages in technology and the availability of hardware and software are making VR a feasible tool for many behavioral researchers rather than for a limited number of specialized VR labs. For example, researchers can now access powerful software engines that allow the creation of rich 3D environments.
There are a few research projects in applied psychological research that utilize IVEs, with a particular focus on therapeutic applications of approach and avoidance behaviors. For example, Eiler et al. (2019) developed a demonstrator, which transfers a traditional approach-avoidance task into VR to support the therapy of substance dependency diseases. Moreover, Mostajeran et al. (2019) developed and evaluated four approach-avoidance-based VR applications in the context of alcohol addiction therapy. Other examples include approach-avoidance training for reducing cognitive biases towards unhealthy food (Kakoschke et al., 2021), and for treating spider phobia (Dibbets and Fonteyne, 2015).
While results of these application-driven studies appear promising, caution is advised in interpreting the potential advantages of VR-based approach-avoidance research. VR approaches may promise increased ecological validity; after all, body movements in a virtual environment seem to be more realistic simulations of everyday behavior than key-presses. However, there is a lack of basic research systematically testing this assumption. Alternatively, one may argue that some aspects of VR may actually reduce authenticity and/or have unexpected side effects. For example, the perceived weight of the HMD, its weight distribution, and its cable connections may trigger unnatural body postures, alter head or body movements, or slow movements down (e.g., Nichols, 1999). Furthermore, for users to respond as naturally as possible in VR, they need to feel physically present in the IVE. This sense of presence has been shown to be highly dependent on the level of immersion, in particular, the characteristics of the used display, such as its field of view as well as the latency between body movements and visual feedback (Sanchez-Vives and Slater, 2005). This underlines the need for basic research combining and comparing traditional approach-avoidance research paradigms and the unique characteristics of IVEs and their relationship to real-life approach and avoidance behaviors.
A first experiment on the effects of whole-body movements in a VR approach-avoidance task was recently realized by Eder et al. (2021). Participants of their study were instructed to initiate either a forward or a backward body lean or a footstep in response to a visual stimulus in the form of a spider (negative valence) or a sunflower (positive valence). In addition, two opposite visual flow patterns (natural vs. inverted) were implemented. The study revealed significant compatibility effects, meaning that forward movements were initiated faster for flowers than spiders whereas backward movements were initiated faster for spiders than flowers. The effects were not influenced by the type of visual flow, which the authors interpret as a dominance of whole-body locomotion over visual cues. This interpretation is in contrast to the conclusion of (Rougier et al., 2018) who argue that the results of their VAAST studies indicate that the most relevant sensorimotor modality involved was the visual modality rather than the motor one. While the study by Eder et al. (2021) is highly informative, its ecological validity is questionable given that stimuli appeared larger-than-life-sized in a highly artificial matrix-type environment, which may have artificially boosted effect sizes and may also explain why they observed only inconsistent relationships to self-reported attitudes.
To gain a better understanding of the contribution of visual information in combination with whole-body motor information on approach-avoidance effects, we implemented a direct comparison of an approach-avoidance task in a desktop-based environment and an IVE. Details on the study, including the results and discussion, are presented in the following sections.
We conducted a study to compare an own implementation of an approach-avoidance task in VR (AAVR) with a desktop-based standard task (VAAST; Rougier et al., 2018). Similar to Eder et al. (2021), we used virtual spiders as negative stimuli but contrasted them with virtual butterflies as positive stimuli. More importantly, we employed more than one stimulus per category and all stimuli were presented in close to natural size and in a natural environment (on the leaves of a plant). In two blocks of trials, participants were instructed to react either with valence-compatible movements (i.e., approach-butterfly, avoid-spider) or valence-incompatible movements (i.e., approach-spider, avoid-butterfly). We chose this block-wise procedure in accordance with the typical implementation of the VAAST (Rougier et al., 2018, Rougier et al., 2020) and most other approach-avoidance paradigms in experimental psychological research. While participants’ responses consisted of physical forward and backward steps in the VR task, they responded via key-presses in the desktop-based task. In both tasks, we measured response latencies as a dependent variable. The aim of the study was to investigate whether and to what extent compatibility effects (defined as faster responses in valence-compatible movements compared to valence-incompatible movements) can be reproduced in IVEs. Furthermore, we were interested in differences between effect sizes in the virtual environment and the desktop-based measurement as well as in inter-correlations of both measures and correlations to self-reported attitudes towards spiders and butterflies. We report how we determined our sample size, all data exclusions, all manipulations, and all measures in the study. Materials and the experimental script for the desktop-based task, all raw data, and analysis code are available in the Open Science Framework1.
In the context of the user study, we pre-registered three hypotheses:
(H1) We expected to observe significant approach-avoidance compatibility effects in both the VAAST and the AAVR. Our assumption that both measures should be sensitive to automatic stimulus evaluations is based on findings that for the majority of people spiders and butterflies are negative and positive stimuli (Rinck and Becker, 2006; Krieglmeyer and Deutsch, 2010; Reinecke et al., 2015). Significant compatibility effects would thus conceptually replicate the results of previous approach-avoidance research both in a novel virtual environment and a desktop-based task (e.g., Krieglmeyer and Deutsch, 2010; Rougier et al., 2018; Eder et al., 2021).
(H2) In line with H1, we expected to observe significant positive inter-correlations between compatibility effects in the VAAST and the AAVR indicating that both measures would be sensitive for individual differences in evaluative responses to the stimuli (e.g., Ellwart et al., 2006).
(H3) As support of external validity of both tasks, we expected positive correlations between compatibility effects and self-reported fear of spiders, such that participants with higher levels of self-reported fear of spiders would show larger compatibility effects in both environments (e.g., Rinck and Becker, 2007).
Additionally, we explore effect size differences for the compatibility effects of the two measures and their predictive values for interindividual differences in self-reported fear of spiders. Given that the task in AAVR offers an immersive environment with a potential for a high sense of presence (Slater and Sanchez-Vives, 2016), uses natural full-body movements instead of key-presses as responses, and provides improved visual flow in a larger portion of the user’s field of view, one may presume that the AAVR outperforms the VAAST in terms of effect size and/or sensitivity for interindividual differences (and thus external validity).
For the virtual environment, we developed a digital textured 3D replica of the real laboratory of the Social Psychology Department at the Universität Hamburg, to which we added a model of a plant and a round base plate (see Figure 1). The plant, as well as the markings on the floor, were placed in the same location as in the physical laboratory. By this means, participants of the study had the opportunity to touch the plant with passive haptic feedback, and therefore, to experience a greater sense of presence. The size of the digital laboratory was 6 × 6 m, which corresponds to the physical dimensions of the real laboratory.
FIGURE 1. Illustration of the virtual environment, which is a replica of the real laboratory including the models of a plant and a round base. The inset shows the view from a participant’s perspective.
As stimuli, we placed 3D models of butterflies and spiders on a leaf of the plant [see Figure 1 (inset)], since this is a relatively natural location for both species. We used eight different stimuli, four of which were butterflies and four were spiders (see Figure 2). These were created based on three virtual mid-poly models of spiders and one butterfly model purchased from TurboSquid (2019). All stimuli were animated, with the butterflies slowly opening and closing their wings on the spot and the spiders slightly bobbing up and down. The stimuli were placed on the plant at a distance of 1.34 m from the base plate. To be able to identify them well at this distance, all stimuli were slightly increased in size. A representation in natural size was not possible due to the required space between plant and base plate. At the beginning of the study, the virtual plant was scaled according to the body height of each participant determined by the Y-coordinate of the HMD to be able to present the stimuli approximately at eye level independent of the height of participants.
The virtual environment was developed in Unity 2019.2.9f1. For controlling the user study, we utilized the Unity Experiment Framework (Brookes et al., 2020).
For the AAVR task, we used an HTC Vive Pro with 1440 × 1600 pixels resolution per eye and a refresh rate of 90 Hz. During the study, the HMD was connected to a workstation with a 4.20 GHz Intel Core i7-7700k processor and two NVIDIA GeForce GTX 1080 Ti graphics cards. We used a wireless adapter to counteract measurement errors caused by the weight of cables at the back of the head that might restrict fast and spontaneous head movements. The HMD was tracked using two HTC Vive base stations and a SteamVR Unity integration. We dispensed with the Vive controllers since all interactions between the study participants and the virtual environment were based on collisions between the HMD and virtual objects. In particular, the 16 cm virtual base plate served as the most important interaction interface in the AAVR task.
The VAAST was implemented using Inquisit 4.0.2, with a structure that followed the original study of Rougier et al. (2018). Screenshots of the virtual laboratory, including the plant, served as background images in the VAAST task. Screenshots were recorded with a resolution of 2560 × 1920 pixels and presented in full-screen mode on a Windows 7 computer during the study. For responses in the VAAST task, the numpad of a keyboard was used.
At the beginning of the study, we introduced participants to both the scenario and the VR technology. We explained that the study examined approach and avoidance behavior with respect to spiders and butterflies, but we neither mentioned the presumed valence of the stimuli nor the division into compatible and incompatible blocks. The participants were informed about the response time measurements, and we instructed them to respond as quickly and accurately as possible in both the AAVR and VAAST. After receiving initial instructions, each participant first performed the AAVR, followed by the VAAST and the self-reports.
For the AAVR, participants positioned themselves on the ground marker and put on the HMD. We instructed the participants to explore and familiarize themselves with the virtual environment in order to reduce eventual distractions during the approach-avoidance task. After the exploration phase, participants returned to the marker, and the experimenter explained the procedure.
In a first block of eight trials, participants familiarized themselves with the HMD, task instructions, and error feedback while their behavior was not recorded. A small black cubicle was used as a stand-in for the stimuli during this training phase.
If there were no further questions, the AAVR task with recorded responses began. Every participant went through a compatible block (approach butterfly/avoid spider) and an incompatible block (approach spider/avoid butterfly), each consisting of 64 trials. The block order was counterbalanced between participants. At the beginning of each block, specific instructions were displayed in front of the virtual plant. Before each trial, the participant had to stand still in the base position for at least one second and gaze in the direction of the plant. A short tone indicated the start of a trial. To prevent mechanical or rhythmic responding, we inserted a random pause of 800–2000 ms between the start tone and the appearance of the stimulus. This timing was based on the VAAST (Rougier et al., 2018). At the appearance of each stimulus, the participant’s position (i.e., the x,y,z coordinates of the HMD) was stored together with the current time in milliseconds. Participants were instructed to take one step forward or backward. A forward or backward movement was interpreted as a step as soon as the distance from the stored starting position changed by 14 cm (cf. Eder et al., 2021). At this threshold, the time was recorded again, and the time of stimulus onset was subtracted to calculate the reaction latency for the trial. One second after the 14 cm threshold was reached, the stimulus faded out, and the participants’ end position and entire trial duration were stored. The participants then returned to the base. The next trial started with the start tone as soon as both starting conditions were fulfilled (standing on the base plate for one second and looking toward the plant).
During each block, we used pseudo-randomization of stimulus sequence, ensuring that the same stimulus was not presented in two consecutive trials and that each stimulus was presented eight times per block. Participants were allowed to take self-paced breaks at any time.
After completing the AAVR task, we conducted an additional exploratory minimal distance measurement. For this purpose, participants returned to the starting marker, and a butterfly, as well as a spider, were presented on the plant leaf, one after the other. The experimenter instructed participants to move their faces towards the animal on the leaf as closely as they felt comfortable. This task was self-paced to avoid any subjective time pressure. We recorded the minimal distance between the position of the HMD and the spider as well as the butterfly. We presumed that this minimal distance measurement would represent controlled aspects of approach behavior compared to the more automatic responses during the approach-avoidance compatibility task.
After completing the VR measurements, participants removed the HMD and were accompanied to an adjacent room to be seated at a computer. There, they completed four presence items adapted from a questionnaire by Usoh et al. (2000) (e.g., “I had the impression to really be there.”) using response sliders ranging from 0 (low sense of presence) to 7 (high sense of presence).
In the second part of the study, participants completed an adapted desktop-based approach-avoidance task, the VAAST, using screenshots from the virtual environment and stills of the eight stimuli to ensure an equivalent environment for both tasks. Participants responded to each stimulus by pressing the (8) on the numpad (labeled “Forward-Key”) for approach movements and the (2) on the numpad (labeled “Backward-Key”) for avoidance movements. Each trial started with a gray fixation cross in the center of the screen as a signal to initiate the trial by a keypress of the central (5) on the numpad (labeled “Start-Key”). After initiation, the color of the fixation cross changed to white, remaining on the screen for a random duration between 800 and 2000 ms, before being overwritten by the target stimulus, i.e., a spider or a butterfly. Participants were instructed to respond with an approach or avoidance movement by pressing the required key as quickly and as accurately as possible. Each correct response resulted in the entire visual display zooming in or out—giving the visual impression of moving one step forward or backward, respectively. A trial terminated 1000 ms after the keypress or directly after an error message was displayed for 1000 ms following an incorrect response. The trial sequence continued immediately with the initiation signal for the next trial.
All other aspects of the task, such as training trials, total trial number, block order, and randomization, were held identical to the AAVR task. Particularly, identical verbal descriptions for forward and backward movements were used in the instructions of both tasks.
After the VAAST, participants completed several self-report inventories to allow for correlation analyses between subjective attitudes towards the stimuli and the behavioral responses in the approach-avoidance tasks. To assess mild and severe forms of arachnophobia, we used a German translation of the 18-item Fear of Spiders Questionnaire (e.g., “I would feel very nervous if I would see a real spider.“, “Spiders are my worst fear.“; Szymanski and O’Donohue, 1995; Rinck et al., 2002). In addition, we individually presented images of all the spiders and butterflies used in the approach-avoidance tasks and asked the participants to evaluate each stimulus (on a scale ranging from −3 very negative to +3 very positive) as well as the arousal caused by each stimulus (on a scale ranging from −3 very calming to +3 very arousing). Finally, participants answered some demographic questions and filled in a consent form to permit the storage and analyses of the collected data.
We collected valid data from 75 participants (52 female, 22 male, 1 missing), mostly psychology students, who participated in exchange for course credits. Participants’ age ranged from 18 to 64 years (Md = 23, M = 25.3, SD = 7.7). Self-reported presence averages ranged from 0 to 6.5 (M = 3.927, SD = 1.549). Due to technical problems, the VR data of one participant was missing and the VR data of three further participants was invalid or incomplete. Sample size was based on a pre-registered power analysis suggesting a minimum sample size of N = 64 participants, in order to detect a Pearson’s correlation coefficient of r = 0.30 (with α = 0.05 and 1 − β = 0.80), which can be interpreted as a medium effect size. Post hoc analyses indicated that the collected sample size provided sufficient test power for all of the observed compatibility effects with 1 − β > 0.90.
In both tasks, we expected significant interaction effects between stimulus and movement (H1), such that valence-compatible movements (approach-butterfly, avoid-spider) would be faster than valence-incompatible movements (approach-spider, avoid-butterfly). In addition, we also analyzed the intercorrelation of compatibility scores of both measures (H2) as well as their correlations to self-reported fear of spiders and aggregated stimulus evaluations (H3). Note that analyses of response latencies can be biased by outliers and extreme values, such as slow responses caused by momentary inattention or distraction. Various methods have been proposed to correct response time analyses for outliers (e.g., Ratcliff, 1993), but there are no agreed-upon norms for outlier treatment. We, therefore, pre-registered to apply several methods, such as fixed cut-offs of slow response times (RTs
A 2 (stimulus: spider vs. butterfly) × 2 (movement: approach vs. avoid) ANOVA of log-transformed response latencies revealed a significant interaction effect, F(1, 73) = 4.290, p = 0.042, η2 = 0.055. This interaction was mainly driven by avoidance effects: Participants were significantly faster to avoid spiders (M = 1062 ms, SD = 101) than butterflies (M = 1101 ms, SD = 105), t(73) = 4.296, p < 0.001, d = 0.494. However, participants showed no significant response time difference approaching butterflies (M = 1003 ms, SD = 94) and spiders (M = 1002 ms, SD = 104), t(73) = 0.272, p = 0.786, d = 0.032 (see Figure 3). While this result confirmed H1, the overall effect size was unexpectedly small (explaining only 5.5% of the variance in response times) and not entirely robust against different outlier treatments (see supplemental materials, Supplementary Table S1). There was a numerical trend for larger compatibility effects for participants who reported relatively higher levels of presence, but this relationship was not significant, r(74) = 0.136, p = 0.249.
FIGURE 3. Mean response times and Standard Errors of (blue) approach movements and (red) avoidance movements in (A) the VR-based task and (B) the desktop-based task. Lighter colors indicate response times in the first blocks of the AAVR task only.
In further exploratory analyses, we included trial position within experimental blocks (1st, 2nd, 3rd, 4th quarter with 16 trials per block) as additional factor into the ANOVA and observed that it significantly influenced response times, F(3, 70) = 2.855, p = 0.043, η2 = 0.109. More precisely, we observed the expected significant interaction effect of stimulus and movement on response latencies when analyzing each block’s first quarter of trials, F(1, 73) = 12.406, p = 0.001, η2 = 0.145, which was relatively robust against outlier treatment (see Supplementary Table S2). Thus, in their initial responses, participants were significantly faster to avoid spiders (M = 1055 ms, SD = 105) than butterflies (M = 1116 ms, SD = 118), t(73) = 4.217, p < 0.001, constituting a moderate effect size d = 0.490. Similarly, participants responded faster approaching butterflies (M = 973 ms, SD = 98) than spiders (M = 995 ms, SD = 109), but the effect missed the conventional level of significance, t(73) = − 1.677, p = 0.098, d = 0.183 (see Figure 3). In contrast to the first block, the interaction effect was not significant in any of the later block’s trials (see supplement for detailed analyses). Note that the compatibility effect in the first block was independent of participants’ level of presence, r(74) = − 0.056, p = 0.636.
We conducted the same 2 (stimulus: spider vs. butterfly) × 2 (movement decision: approach vs. avoid) ANOVA on log-transformed response latencies in the VAAST, which revealed the expected significant interaction effect with F(1, 74) = 23.877, p < 0.001, η2 = 0.244. Participants responded significantly faster avoiding spiders (M = 585 ms, SD = 89) than butterflies (M = 628 ms, SD = 90), t(74) = 6.173, p < 0.001, d = 0.713, and, vice versa, responded significantly faster approaching butterflies (M = 578 ms, SD = 95) than spiders (M = 594 ms, SD = 86), t(74) = − 2.519, p = 0.014, d = 0.291 (see Figure 3). Contrary to the AAVR task, there was no significant effect of trial number on response time; that is, compatibility effects were approximately the same in all four quarters of the task.
For analyzing the exploratory minimal distance measure, we calculated the Euclidean distance between the HMD and the center of each stimulus. We expected that participants would move closer towards the butterfly as compared to the spider. These expectations were confirmed: Participants’ minimal distance to spiders (M = 0.393 m, SD = 0.290) was significantly larger than their minimal distance to butterflies (M = 0.252 m, SD = 0.125), t(70) = 4.451, p < 0.001, d = 0.564.
In order to explore inter-individual differences, we first calculated composite scores of the behavioral measures. Therefore, we subtracted the mean response time in valence-behavior compatible trials (avoid-spider, approach-butterfly) from the mean response time of incompatible trials (approach-spider, avoid-butterfly). The resulting composite compatibility scores represent the stimulus-movement interaction in one score (in milliseconds), with positive values indicating relative facilitation of avoidance for spiders and approach for butterflies. Because of the observed effect size differences between blocks in the AAVR, we calculated an additional AAVR composite score based on the first block of trials only. For the minimal distance measure and the stimulus ratings, we computed single difference scores of responses to spiders and responses to butterflies. Positive values indicate (1) a relative closer approach to the butterfly than to the spider in the minimal distance measure in cm, (2) a generally more positive evaluation of butterflies than spiders in the valence ratings, and (3) higher scores for butterflies than for spiders in the arousal rating. Descriptive statistics and correlation coefficients are listed in Table 1.
TABLE 1. Correlations between compatibility scores and self-reported measures (p-values in parentheses).
As can be seen in Table 1, the two approach-avoidance scores were positively correlated. However, these correlations constitute only small-to-moderate effect sizes. Furthermore, the compatibility effects in the AAVR task were not related to any other measure, whereas the compatibility effect in the desktop-based VAAST was only related to stimulus valence ratings. Again, this correlation coefficient constitutes a small-to-moderate effect size.
Finally, the minimal distance measure in VR showed the biggest correlations to the three self-report measures. That is, the more participants evaluated spiders as negative and arousing than butterflies and the higher their self-reported fear of spiders, the more distance participants kept to a spider as compared to a butterfly when instructed to move as closely as possible to the virtual stimuli. Interestingly, the minimal distance measure was not significantly related to the composite scores of the approach-avoidance measures, neither for the AAVR nor for the VAAST.
In the current study, we implemented two different behavioral tasks as measures of automatic approach vs. avoidance tendencies towards spiders and butterflies. Both behavioral measures, the AAVR and the desktop-based VAAST, showed the expected significant compatibility effects in that participants responded faster in congruent trials (avoid-spider, approach-butterfly) than in incongruent trials (approach-spider, avoid-butterfly) (H1). The effect in VR, however, was relatively small and not very robust and seemed to fade out throughout trial repetitions. Both effects were significantly intercorrelated, suggesting cross-modal validity (H2). Furthermore, the VAAST compatibility effect was only significantly related to stimulus valence ratings but no other self-report measures, whereas the AAVR compatibility effect was not related to any of the self-report measures (H3).
In an additional exploratory measure implemented in the virtual environment, we had instructed participants to move as closely as they could comfortably do towards one virtual spider and one virtual butterfly. This task yielded a significant difference between the two types of stimuli that was significantly related to participants’ self-reported attitudes. In the following, we will discuss the results of our statistical analyses with respect to the previously stated hypotheses. We will consider multiple factors that may have influenced the results, in particular with a focus on the interpretation of the AAVR measurements.
Participants in this study were instructed to respond as quickly as possible to virtual spiders and butterflies by taking one step towards or away from them. We had expected that valence-compatible movements would be initiated and executed faster compared to incompatible movements (H1) and explored whether this effect would be larger for task execution in VR as compared to the desktop-based task. While hypothesis (H1) was confirmed for both tasks, the effect in the VR-based task was unexpectedly small compared to the desktop-based task and not robust against outlier treatment. Exploratory analyses further showed that the expected interaction effect between stimulus and movement only occurred in the first 16 trials of each block, for which it showed a large effect size. While we interpret this initial compatibility effect as an indicator of automatic approach and avoidance tendencies that facilitated congruent behavior and/or inhibited incongruent behavior, the effect seems to diminish the more often the same movements were repeated. This may be due to some sort of habituation or behavioral practice effects or a combination of both, an effect that may have overwritten initial spontaneous action tendencies. Habituation refers to the repeated exposure to the stimuli, which may reduce their affective and/or arousing quality over time. For example, such habituation effects are typically used in therapeutic settings, in which enduring exposure to feared stimuli reduces fear responses (Benito and Walther, 2015). In particular, exposure therapy via VR HMDs has been shown to be effective for the treatment of spider phobia (Garcia-Palacios et al., 2002; Lindner et al., 2020). Although such habituation effect could in principle have decreased avoidance tendencies to spider stimuli over time, we deem this an unlikely explanation, given that we observed strong and robust compatibility effects in the following desktop-based task. A second interpretation of the decreasing compatibility effect with an increasing number of repetitions is based on practice effects. Because the task involved a high number of trial repetitions (with altogether 64 forward steps and 64 backward steps), participants may have formed strategies to mechanistically fulfill the task instructions in order to respond as fast as possible. Such factors were reported in previous approach-avoidance studies (Klein et al., 2011) and may explain the observed dependency of compatibility effects on trial number. Interestingly, no such effects were observed in the desktop-based variant of the task, which consistently showed compatibility effects over the course of 2 × 64 trials. This difference between the VAAST and AAVR results may indicate that the varying physical demand represents another influencing factor in our study design. While participants had to respond via keypresses in the VAAST, repeated whole-body movements in the AAVR may have led to increased fatigue and reduced sensitivity to stimulus valence. Similarly, there may be other, yet unnoticed, context differences between the two tasks that explain their differences.
Alternatively, one may conclude that both tasks only partly measure overlapping action tendencies. For example, given the general difference in response speed between the two tasks, one may argue that the AAVR with an average response speed of
We had expected to observe significant positive inter-correlations between compatibility effects in the VAAST and the AAVR (H2). This hypothesis was indeed confirmed, albeit effect sizes were rather small (rs ≤ 0.275). This result indicates that both measures were somehow sensitive for shared between-individual variance in the effects. Compatibility effects in both tasks were mostly unrelated to participants’ self-reported fear of spiders and their evaluations of the presented stimuli, thus, not supporting our hypothesis (H3). There was only one exception, which is the small and only marginally significant correlation of the VAAST compatibility effect with participants’ average valence ratings of stimuli. At first sight, the correlational results appear to indicate a lack of external validity, because individual effect size differences in the compatibility effects were not predictive of individual differences in self-reported attitudes. However, this observation could also be due to the relatively low variation of attitudes towards spiders within the sample, given that the majority of participants expressed to have no or little fear of spiders (as measured with the Fear of Spiders Questionnaire, see Section 3.3). A more diverse sample covering different intensities of arachnophobia may be necessary to reveal correlations of the considered measures (but see Rinck and Becker, 2007).
The aforementioned lack of correlation between compatibility effects and self-reported fear of spiders could also indicate that the measures were conducted under different processing conditions. Whereas AAVR and VAAST may have tapped more automatic—in terms of speeded—action tendencies, the self-report measure may have tapped slower and deliberate responses. This interpretation is corroborated by the finding that the self-report measures were only correlated with the minimal distance measure, which did not require speeded responding and which we presume to have been under participants’ intentional and conscious control rather than driven by automatic action tendencies. This presumption is based on the procedure of distance measurement since the experimenter explicitly instructed participants to move as close to the stimuli as they felt comfortable with and to act without time pressure. At this point, these interpretations remain speculative, and further research is needed to understand the interplay of automatic action tendencies, deliberate behavioral decisions, and self-reported attitudes.
There are some procedural characteristics of the current task design that limit the interpretability and generalizability of our findings and thus require further investigations of the used approach-avoidance measure in VR. As elaborated before, our results indicate that the original task structure contained too many trials, which may have made the task less sensitive to automatic action tendencies of approach and avoidance. While a high number of trials is desirable from a measurement point of view (as it promises a more reliable estimation of effects), it is problematic if repetition alters the monitored behavior of participants. Further empirical studies are necessary to estimate the optimal trial number that allows reliable measurement without compromising observed effects. This may also be supported by creating smaller blocks and repeatedly altering compatible and incompatible task instructions.
Another limiting factor may be the fixed order of the tasks. While the sequence of compatible and incompatible blocks was counterbalanced between participants, and the presentation of stimuli was pseudo-randomized within each block, every participant started with the AAVR task before completing the VAAST. In future studies, we plan to counterbalance both conditions in order to exclude possible order effects. In particular, the previously mentioned practice effects could have caused or intensified the observed compatibility effects in the VAAST since all participants already performed two blocks of 64 trials each in the AAVR task.
For further research studies, we also deem it valuable to record participants’ positions throughout the task continuously. For our current analyses, we defined a fixed step length of 14 cm that had to be reached before a forward or backward movement was registered and measurements of the response latency were initiated. However, this procedure may have introduced measurement errors, given that natural step length depends on participants’ body size, and 14 cm may constitute a relatively big step for short people but a relatively small step for tall people. Continuous position tracking allows investigating step length itself as an additional dependent variable. Although participants may be able to follow instructions and thus initiate approach and avoidance movements towards positive and negative stimuli with comparable response latency, automatic behavioral tendencies may alter the length of the steps they take (e.g., shorter steps towards unpleasant stimuli). In line with this, automatic behavioral tendencies may be observable in other characteristics of the behavior (e.g., body sways) rather than mere response times.
Finally, using butterflies as a contrasting stimulus category may have caused undesired side effects. During the conception of this study, butterflies appeared as an appropriate control condition due to their commonly assumed positive valence and their shared characteristics with the target category of spiders (e.g., size, likelihood to sit on leaves). Remarks by participants, however, pointed us towards one important caveat: In order to observe a butterfly from up close, approaching movements usually have to be very slow and careful to not shoo the insect away. Thus, whereas the spontaneous, positive evaluations may trigger automatic approach tendencies, participants may have learned to suppress these action tendencies and approach with care. Additionally, it turned out that butterflies were not unambiguously positive for all participants, but appeared as creepy creatures to some. In that line, prior research has shown that fear of spiders is largely related to the specific movement patterns of spiders (Davey, 1991), which are also typical for many insects. It thus seems advisable to use a different contrast stimulus category to which such limitations do not apply, such as flowers.
In this article, we investigated a whole-body movement stimulus-response compatibility task to explore automatic approach-avoidance behavior in VR. We implemented two conditions, one in an IVE, one in a desktop-based environment, with identical positively and negatively valenced stimuli (butterflies and spiders, respectively). The conditions differed from each other concerning the response mechanisms to approach or avoid displayed stimuli. In the desktop-based task, participants of the study pressed keys that represented approach and avoidance decisions, while in the VR task, whole-body movements were performed. In both environments, we found compatibility effects that were positively correlated. Interestingly, however, the compatibility effect in the VR environment was unrelated to participants’ self-reported fear of spiders and stimulus evaluations. We discussed a number of potential influencing factors and identified procedural changes to the approach-avoidance task in VR, which should be considered in follow-up studies.
While the current results only allow preliminary conclusions that require further research for corroboration, they also provide an interesting outlook. The two behavioral measures that we implemented in VR seem to be sensitive for different aspects of behavioral control. We propose that response latency of compatible and incompatible approach and avoidance movements may be sensitive for automatic behavioral tendencies of facilitation and inhibition. The minimal distance measure, on the other hand, may be more sensitive for deliberate and voluntary behavioral control. This interpretation is supported by the pattern of correlations to self-report measures and stimulus evaluations. Exploring these measures and their interplay may provide an interesting future approach to the measure of automatic vs controlled behaviors, which is at the forefront of many research endeavors in social psychology and may have high application value in attitude domains (e.g., interpersonal attitudes and social group attitudes).
The datasets presented in this study can be found in online repositories. The names of the repository/repositories and accession number(s) can be found below: https://osf.io/wyge3/.
Ethical review and approval was not required for the study on human participants in accordance with the local legislation and institutional requirements. The participants provided their written informed consent to participate in this study.
JD designed the study, programmed the desktop-based data collection (i.e., VAAST, self-reports), conducted all statistical analyses, and wrote the first draft of this manuscript. LS programmed the virtual environment and acted as instructor and experimenter for data collection. FS and SS contributed to writing the manuscript.
The authors declare that the research was conducted in the absence of any commercial or financial relationships that could be construed as a potential conflict of interest.
All claims expressed in this article are solely those of the authors and do not necessarily represent those of their affiliated organizations, or those of the publisher, the editors and the reviewers. Any product that may be evaluated in this article, or claim that may be made by its manufacturer, is not guaranteed or endorsed by the publisher.
The Supplementary Material for this article can be found online at: https://www.frontiersin.org/articles/10.3389/frvir.2021.761142/full#supplementary-material
1https://osf.io/wyge3/. Due to copyright restrictions on the virtual stimuli used in the AAVR, we cannot make this code openly available, but share it after contacting the first or fourth author
Barsalou, L. W. (2008). Grounded Cognition. Annu. Rev. Psychol. 59, 617–645. doi:10.1146/annurev.psych.59.103006.093639
Barsalou, L. W. (2015). “Situated Conceptualization: Theory and Applications,” in Perceptual and Emotional Embodiment (New York: Routledge/Taylor and Francis Group), 19–45.
Beatty, G. F., Cranley, N. M., Carnaby, G., and Janelle, C. M. (2016). Emotions Predictably Modify Response Times in the Initiation of Human Motor Actions: A Meta-Analytic Review. Emotion 16, 237–251. doi:10.1037/emo0000115
Benito, K. G., and Walther, M. (2015). Therapeutic Process during Exposure: Habituation Model. J. Obsessive-Compuls. Relat. Disord. 6, 147–157. doi:10.1016/j.jocrd.2015.01.006
Brookes, J., Warburton, M., Alghadier, M., Mon-Williams, M., and Mushtaq, F. (2020). Studying Human Behavior with Virtual Reality: The Unity Experiment Framework. Behav. Res. 52, 455–463. doi:10.3758/s13428-019-01242-0
Chen, M., and Bargh, J. A. (1999). Consequences of Automatic Evaluation: Immediate Behavioral Predispositions to Approach or Avoid the Stimulus. Pers Soc. Psychol. Bull. 25, 215–224. doi:10.1177/0146167299025002007
Davey, G. C. L. (1991). Characteristics of Individuals with Fear of Spiders. Anxiety Res. 4, 299–314. doi:10.1080/08917779208248798
De Houwer, J., Crombez, G., Baeyens, F., and Hermans, D. (2001). On the Generality of the Affective Simon Effect. Cogn. Emot. 15, 189–206. doi:10.1080/02699930125883
Degner, J., Essien, I., and Reichardt, R. (2016). Effects of Diversity versus Segregation on Automatic Approach and Avoidance Behavior towards Own and Other Ethnic Groups. Eur. J. Soc. Psychol. 46, 783–791. doi:10.1002/ejsp.2234
Dibbets, P., and Fonteyne, R. (2015). High Spider Fearfuls Can Overcome Their Fear in a Virtual Approach-Avoidance Conflict Task. J. Depress. Anxiety 4, 1–7. doi:10.4172/2167-1044.1000182
Eagly, A. H., and Chaiken, S. (1993). The Psychology of Attitudes. Harcourt brace Jovanovich college publishers.
Eder, A. B., and Rothermund, K. (2008). When Do Motor Behaviors (Mis)match Affective Stimuli? an Evaluative Coding View of Approach and Avoidance Reactions. J. Exp. Psychol. Gen. 137, 262–281. doi:10.1037/0096-3445.137.2.262
Eder, A. B., Krishna, A., Sebald, A., and Kunde, W. (2021). Embodiment of Approach-Avoidance Behavior: Motivational Priming of Whole-Body Movements in a Virtual World. Motiv. Sci. 7, 133–144. doi:10.1037/mot0000205
Eiler, T. J., Grünewald, A., Machulska, A., Klucken, T., Jahn, K., Niehaves, B., et al. (2019). “A Preliminary Evaluation of Transferring the Approach Avoidance Task into Virtual Reality,” in Information Technology in Biomedicine. Advances in Intelligent Systems and Computing, Osaka, Japan, March 23–27, 2019 1011, 151–163. doi:10.1007/978-3-030-23762-2_14
El Jamiy, F., and Marsh, R. (2019). Survey on Depth Perception in Head Mounted Displays: Distance Estimation in Virtual Reality, Augmented Reality, and Mixed Reality. IET Image Process. 13, 707–712. doi:10.1049/iet-ipr.2018.5920
Ellwart, T., Rinck, M., and Becker, E. S. (2006). From Fear to Love: Individual Differences in Implicit Spider Associations. Emotion 6, 18. doi:10.1037/1528-3542.6.1.18
Fazio, R. H., Sanbonmatsu, D. M., Powell, M. C., and Kardes, F. R. (1986). On the Automatic Activation of Attitudes. J. Personal. Soc. Psychol. 50, 229. doi:10.1037/0022-3514.50.2.229
Garcia-Palacios, A., Hoffman, H., Carlin, A., Furness, T. A., and Botella, C. (2002). Virtual Reality in the Treatment of Spider Phobia: A Controlled Study. Behav. Res. Ther. 40, 983–993. doi:10.1016/s0005-7967(01)00068-7
Kakoschke, N., Page, R., de Courten, B., Verdejo-Garcia, A., and McCormack, J. (2021). Brain Training with the Body in Mind: Towards Gamified Approach-Avoidance Training Using Virtual Reality. Int. J. Human-Comput. Stud. 151, 102626. doi:10.1016/j.ijhcs.2021.102626
Klein, A. M., Becker, E. S., and Rinck, M. (2011). Approach and Avoidance Tendencies in Spider Fearful Children: The Approach-Avoidance Task. J. Child Fam. Stud. 20, 224–231. doi:10.1007/s10826-010-9402-7
Krieglmeyer, R., and Deutsch, R. (2010). Comparing Measures of Approach–Avoidance Behaviour: The Manikin Task vs. Two Versions of the Joystick Task. Cogn. Emot. 24, 810–828. doi:10.1080/02699930903047298
Krieglmeyer, R., De Houwer, J., and Deutsch, R. (2013). On the Nature of Automatically Triggered Approach–Avoidance Behavior. Emot. Rev. 5, 280–284. doi:10.1177/1754073913477501
Lindner, P., Miloff, A., Bergman, C., Andersson, G., Hamilton, W., and Carlbring, P. (2020). Gamified, Automated Virtual Reality Exposure Therapy for Fear of Spiders: A Single-Subject Trial under Simulated Real-World Conditions. Front. Psychiatry 11, 116. doi:10.3389/fpsyt.2020.00116
Loomis, J., Blascovich, J., and Beall, A. (1999). Immersive Virtual Environment Technology as a Basic Research Tool in Psychology. Behav. Res. Methods Instr. Comput. 31, 557–564. doi:10.3758/bf03200735
Moors, A., and De Houwer, J. (2006). Automaticity: A Theoretical and Conceptual Analysis. Psychol. Bull. 132, 297–326. doi:10.1037/0033-2909.132.2.297
Mostajeran, F., Kirsten, A., Steinicke, F., Gallinat, J., and Kühn, S. (2019). “Towards Gamified Alcohol Use Disorder Therapy in Virtual Reality: A Preliminary Usability Study,” in 2019 IEEE Conference on Virtual Reality and 3D User Interfaces (VR), Osaka, Japan, March 23–27, 2019, 1471–1476. doi:10.1109/vr.2019.8797817
Nichols, S. (1999). Physical Ergonomics of Virtual Environment Use. Appl. Ergon. 30, 79–90. doi:10.1016/s0003-6870(98)00045-3
Oh, C. S., Bailenson, J. N., and Welch, G. F. (2018). A Systematic Review of Social Presence: Definition, Antecedents, and Implications. Front. Rob. AI 5, 114. doi:10.3389/frobt.2018.00114
Opriş, D., Pintea, S., García-Palacios, A., Botella, C., Szamosközi, Ş., and David, D. (2012). Virtual Reality Exposure Therapy in Anxiety Disorders: A Quantitative Meta-Analysis. Depress. Anxiety 29, 85–93. doi:10.1002/da.20910
Phaf, R. H., Mohr, S. E., Rotteveel, M., and Wicherts, J. M. (2014). Approach, Avoidance, and Affect: A Meta-Analysis of Approach-Avoidance Tendencies in Manual Reaction Time Tasks. Front. Psychol. 5, 378. doi:10.3389/fpsyg.2014.00378
Ratcliff, R. (1993). Methods for Dealing with Reaction Time Outliers. Psychol. Bull. 114, 510. doi:10.1037/0033-2909.114.3.510
Reinecke, A., Becker, E. S., and Rinck, M. (2015). Three Indirect Tasks Assessing Implicit Threat Associations and Behavioral Response Tendencies. Z. für Psychologie/Journal Psychol
Rinck, M., and Becker, E. S. (2006). Spider Fearful Individuals Attend to Threat, Then Quickly Avoid it: Evidence from Eye Movements. J. Abnormal Psychol. 115, 231. doi:10.1037/0021-843x.115.2.231
Rinck, M., and Becker, E. S. (2007). Approach and Avoidance in Fear of Spiders. J. Behav. Ther. Exp. Psychiatry 38, 105–120. doi:10.1016/j.jbtep.2006.10.001
Rinck, M., Bundschuh, S., Engler, S., Müller, A., Wissmann, J., Ellwart, T., et al. (2002). Reliabilität und validität dreier instrumente zur messung von angst vor spinnen. Diagnostica 48, 141–149. doi:10.1026//0012-1924.48.3.141
Rotteveel, M., Gierholz, A., Koch, G., van Aalst, C., Pinto, Y., Matzke, D., et al. (2015). On the Automatic Link between Affect and Tendencies to Approach and Avoid: Chen and Bargh. Front. Psychol. 6, 335. doi:10.3389/fpsyg.2015.00335
Rougier, M., Muller, D., Ric, F., Alexopoulos, T., Batailler, C., Smeding, A., et al. (2018). A New Look at Sensorimotor Aspects in Approach/avoidance Tendencies: The Role of Visual Whole-Body Movement Information. J. Exp. Soc. Psychol. 76, 42–53. doi:10.1016/j.jesp.2017.12.004
Rougier, M., Muller, D., Courset, R., Smeding, A., Devos, T., and Batailler, C. (2020). Toward the Use of Approach/avoidance Tendencies as Attitude Measures: Individual-And Group-Level Variability of the Ingroup Bias. Eur. J. Soc. Psychol. 50, 857–875. doi:10.1002/ejsp.2653
Sanchez-Vives, M. V., and Slater, M. (2005). From Presence to Consciousness through Virtual Reality. Nat. Rev. Neurosci. 6, 332–339. doi:10.1038/nrn1651
Seibt, B., Neumann, R., Nussinson, R., and Strack, F. (2008). Movement Direction or Change in Distance? Self and Object Related Approach–Avoidance Movements. J. Exp. Soc. Psychol. 44, 713–720. doi:10.1016/j.jesp.2007.04.013
Slater, M., and Sanchez-Vives, M. V. (2016). Enhancing Our Lives with Immersive Virtual Reality. Front. Robotics AI 3, 74. doi:10.3389/frobt.2016.00074
Solarz, A. K. (1960). Latency of Instrumental Responses as a Function of Compatibility with the Meaning of Eliciting Verbal Signs. J. Exp. Psychol. 59, 239–245. doi:10.1037/h0047274
Szymanski, J., and O’Donohue, W. (1995). Fear of Spiders Questionnaire. J. Behav. Ther. Exp. Psychiatry 26, 31–34. doi:10.1016/0005-7916(94)00072-t
TurboSquid (2019). 3d Models for Professionals. [Dataset]. Available at: https://www.turbosquid.com/(Accessed December 4, 2019).
Usoh, M., Catena, E., Arman, S., and Slater, M. (2000). Using Presence Questionnaires in Reality. Presence: Teleoperators Virtual Environ. 9, 497–503. doi:10.1162/105474600566989
Wentura, D., and Degner, J. (2010). Automatic Evaluation Isn’t that Crude! Moderation of Masked Affective Priming by Type of Valence. Cogn. Emot. 24, 609–628. doi:10.1080/02699930902854587
Wilson, C. J., and Soranzo, A. (2015). The Use of Virtual Reality in Psychology: A Case Study in Visual Perception. Comput. Math. Methods Med. 2015, 1–7. doi:10.1155/2015/151702
Keywords: virtual reality, approach-avoidance, attitude-behavior research, empirical studies, human-computer interaction
Citation: Degner J, Steep L, Schmidt S and Steinicke F (2021) Assessing Automatic Approach-Avoidance Behavior in an Immersive Virtual Environment. Front. Virtual Real. 2:761142. doi: 10.3389/frvir.2021.761142
Received: 19 August 2021; Accepted: 22 November 2021;
Published: 20 December 2021.
Edited by:
Maria Limniou, University of Liverpool, United KingdomReviewed by:
Pieter Van Dessel, Ghent University, BelgiumCopyright © 2021 Degner, Steep, Schmidt and Steinicke. This is an open-access article distributed under the terms of the Creative Commons Attribution License (CC BY). The use, distribution or reproduction in other forums is permitted, provided the original author(s) and the copyright owner(s) are credited and that the original publication in this journal is cited, in accordance with accepted academic practice. No use, distribution or reproduction is permitted which does not comply with these terms.
*Correspondence: Juliane Degner, anVsaWFuZS5kZWduZXJAdW5pLWhhbWJ1cmcuZGU=; Frank Steinicke, ZnJhbmsuc3RlaW5pY2tlQHVuaS1oYW1idXJnLmRl
Disclaimer: All claims expressed in this article are solely those of the authors and do not necessarily represent those of their affiliated organizations, or those of the publisher, the editors and the reviewers. Any product that may be evaluated in this article or claim that may be made by its manufacturer is not guaranteed or endorsed by the publisher.
Research integrity at Frontiers
Learn more about the work of our research integrity team to safeguard the quality of each article we publish.