- 1Centre for Biological Threats and Special Pathogens, Robert Koch Institute, Berlin, Germany
- 2Institute of Novel and Emerging Infectious Diseases, Friedrich-Loeffler-Institut, Greifswald, Germany
- 3Department of Virology, Laboratoire National d’Appui au Développement Agricole, Bingerville, Côte d’Ivoire
Introduction: For more than 40 years, outbreaks of ebolavirus disease have been documented, but the natural reservoir(s) of ebolaviruses remain unknown. However, recent studies provide evidence that the Angolan free-tailed bat (Mops condylurus), an insectivorous bat belonging to the family Molossidae, is a likely ebolavirus reservoir. Being a heterothermic species, M. condylurus bats are highly tolerant to variations in ambient temperatures, and therefore are capable of living under a broad range of climatic and environmental conditions by using adaptive thermoregulation. Body core temperatures as low as 12.0°C have been measured during winter, while increased body temperatures were observed in their hot roosts or during flight, reaching temperatures typical of fever in most other mammalian species.
Methods: Here, we investigated the impact of temperature fluctuations between 27°C and 42°C on Ebola virus (EBOV) survival and replication kinetics in cells from M. condylurus using qRT-PCR.
Results: We found that primary cells derived from M. condylurus, similar to the bats in their natural environment, were highly tolerant to temperature variations. EBOV replication was temperature-dependent, showing a strong reduction of replication efficiency at low temperature.
Discussion: We therefore conclude, that heterothermy might be involved in balancing the level of EBOV replication and thereby be a key factor for tolerating EBOV infections in vivo.
Introduction
Over the past 50 million years and within the very diverse order “Chiroptera”, bats have evolved various mechanisms of temperature tolerance, such as dropping their body temperature during torpor or adaptation to high ambient temperatures. Bats of one Africa-based species, M. condylurus (Angolan free-tailed bat) are capable of enduring a broad range of climatic and environmental (semi-arid to mesic) conditions using adaptive thermoregulation (1). Torpor, historically viewed as an energy-saving technique of bats in temperate and subarctic climates, is also common in several tropical bat families (2). M. condylurus bats were shown to be thermolabile with daily bouts of torpor during winter and summer, with body temperatures closely conforming to ambient temperatures, and core temperature measured to be as low as 12.0°C during winter (3). At night, these bats generally remain euthermic with mean body temperatures between 30-37°C at ambient temperatures between 15-35°C (4). During the day, torpor is commonly employed at lower ambient temperatures with body temperatures being as low as 20°C at 15°C ambient temperature (4). At this temperature (15°C), energy expenditure of torpid bats during the day was shown to be only 17% of nontorpid M. condylurus bats at night (4). Reduced body temperatures and metabolic rates may suppress immune responses and reduce virus replication rates, thereby delaying virus clearance from bat populations (5, 6).
Mean temperatures in roosts of M. condylurus bats were shown to exceed 40°C for over six hours with maximal temperatures above 60°C for up to two hours (7). This bat species uses hot roosts with ambient temperatures that are lethal to many other bats (4), allowing their body temperature to rise in order to save energy, that would otherwise be spent on cooling mechanisms (8). High ambient temperatures were shown to result in increased body temperatures up to 43°C (4).
The minimum flight temperature of M. condylurus bats was determined to be approximately 35°C (7). All individuals raise their body temperature endogenously in the late afternoon in preparation for nocturnal foraging activity with mean body temperatures of bats emerging from the roost of 40.5 ± 1.1°C (7). These body temperatures during bat flight reach the temperature typical of fever in most non-bat mammals (38°C-41°C), resulting in increased metabolic rates, estimated to be 15–16-fold higher than the resting metabolic rate, and possibly stronger immune responses (9). In M. condylurus bats, viruses are exposed to low temperatures during torpor as well as high temperatures during the day in hot roosts and during flight at night. Viruses and their biochemical processes must withstand these temperature extremes without resultant destruction or elimination.
So far, few studies have provided data about the impact of varying temperatures on viruses and their replication, such as adenovirus type 5 (Ad5) (10), highly pathogenic avian influenza virus (HPAIV) (11) and EBOV, which was shown to replicate at 41°C in different bat cells (12). Low virus replication rates were reported for Japanese encephalitis virus (JEV) and rabies virus (RABV) at low incubation temperatures (13–15), while the impact of low incubation temperatures on bat cells and EBOV replication kinetics have not been described.
Outbreak investigations and several epidemiological studies provide evidence that bats are most likely the natural reservoir hosts for ebolaviruses (16, 17). For several outbreaks, there is anecdotal evidence of index patients coming into contact with bats prior to disease (16, 18, 19). Various species of wild-caught bats have been tested for EBOV seroreactivity, with positive results in 307 individual bats from at least 17 species in Africa and Asia (16, 20–28). In contrast to fruit bats, insectivorous bats have received sparse attention in ebolavirus research (29). The discovery of a new ebolavirus, Bombali virus (BOMV), in M. condylurus bats in Sierra Leone (30) and repeated detection of BOMV RNA in M. condylurus bats in Kenia (31) and Guinea (32) indicate, that further investigation into the role of this microbat in the ecology of ebolaviruses is needed. Over the years, there has been a limited number of studies providing evidence that bats of the species M. condylurus might not only be a natural reservoir for BOMV, but might also be a reservoir host for EBOV (18, 22, 33). M. condylurus bats inoculated with EBOV show high and disseminated viral replication and infectious virus shedding, without any clinical disease, while other filoviruses fail to establish productive infections (34). Additionally, a placental-specific tissue tropism in M. condylurus bats and a unique ability of EBOV to traverse the placenta, infect and persist in fetal tissues, which results in distinct genetic signatures of adaptive evolution, was evidenced. Persistent EBOV infection for 150 days in lung primary cells derived from M. condylurus, without resultant selective pressure leading to virus mutation, indicated the intrinsic ability of EBOV to persist in this bat species (35). These findings together demonstrate plausible routes of horizontal and vertical transmission and potential viral persistence in M. condylurus bats, which are expectant of reservoir hosts.
Depending on the cell type, several different factors e.g. C-type lectins, heparan sulfate, TIM-1 or Axl are involved in filovirus attachment to their host cells (36). A key component of the filovirus entry process is the integral membrane protein Niemann-Pick C1 (NPC1), found in late endosomes/lysosomes (36) and utilized in the viral entry process by the glycoproteins (GP) of EBOV (37–39), Marburg virus (MARV) (40) and Měnglà virus (MLAV) (41). M. condylurus-derived cell cultures typically display low NPC1 expression levels compared to cells from a highly symptomatic host, such as humans (42), and infection with EBOV revealed a correlation between NPC1 receptor expression levels and virus titers (35, 42).
In this study we investigated the impact of temperature fluctuations at these extreme limits in cells from M. condylurus bats on NPC1 receptor expression levels and EBOV survival and replication. We found that primary cells from M. condylurus bats tolerated low and high temperatures in contrast to other mammalian cell cultures. NPC1 receptor expression levels were notably higher at low temperatures and EBOV replication rates were temperature-dependent, showing a strong reduction of EBOV replication efficiency at low temperature. Thus, heterothermy of M. condylurus bats might be involved in balancing the level of virus replication, and thereby may be a potential key factor in this conceivable reservoir species for tolerating EBOV infections in vivo.
Materials and methods
Ethics statement
Animal work was performed with the permission of the Laboratoire Central Vétérinair, Laboratoire National d’Appui au Développement Agricole (LANADA), Bingerville, Ivory Coast (No. 05/virology/2016). The animal care and use protocol adhered with the Ethics Committee of LANADA and National Ethics Committee for the Research (CNER). Consent existed to measure the temperature in the bat roost from the owners of the residence in Koffikro Village.
Measuring ambient temperature in bat roost
Ambient temperature variations inside and outside a roost of M. condylurus bats were determined measuring the temperature every 30 min. An iButton logger (Maxim Integrated) was placed for five days in a bat roost under the roof of a house in Koffikro village, Ivory Coast (geographic coordinates: N 05° 19.340´; W 003° 49.431´) in November 2016.
Cell culture
All used cell cultures were summarized in Table 1. The establishment of microbat cell cultures, media and culture conditions were previously described in detail in Bokelmann et al. (42). Control cells (HEK293, HeLa and Vero E6) were cultured in DMEM (Sigma-Aldrich, D6546) containing 5% (for infection experiments) or 15% FBS (Biochrom, 1318D), 2 mM L-glutamine (Gibco™, 25030-081), 50 U/ml penicillin and 50 µg/ml streptomycin (Pen-Strep, Gibco™, 15140122).
Cell growth and viability at different temperatures
All cells were grown in six-well plates and incubated for 96 h at 27°C, 37°C or 42°C with 5% CO2, whereby 37°C served as a control temperature generally used for mammalian cell lines. For incubation at 17°C we operated an incubator (Small incubator, Hartmann) inside a cooling chamber (4°C). Morphological changes and adherence of cells were monitored using phase contrast microscopy (EVOS™ XL Core, Life Technologies or Eclipse TS100, Nikon/DS-Fi2 Microscope Camera, Nikon). Cell growth and viability were investigated for 96 h at 27°C, 37°C or 42°C for the same microbat cell cultures and HEK293 cells as control. For this, the cell number and viability were determined by counting triplicate wells for each cell isolate for each condition after 48 and 96 h, using 0.4% trypan blue stain and an automated cell counter (EVE™, NanoEnTek), and presented as geometric means with geometric standard deviations.
Infection and viral RNA quantitation
Infectious work with Ebola virus (EBOV; strain Makona, C05; adaptation: 2 passages on MoKi cells) and Marburg virus (MARV; strain Musoke) was performed in the biosafety level 4 (BSL4) facility at the Robert Koch Institute, according to standard operating protocols (SOPs). HEK293, MoKi, MoBra Prim and MoLu Prim cells were seeded in six-well plates in triplicates and one plate per cell culture and per temperature (12 plates in total) was incubated over night at 27°C, 37°C or 42°C with 5% CO2. Cells were infected with EBOV with 0.6 TCID50/cell or with MARV with 0.02 TCID50/cell for 1 h at 27°C, 37°C or 42°C. Cells were washed twice with PBS, before 3 ml cell culture medium was added. For EBOV and MARV quantitation at different constant temperatures, 140 µl of supernatant per well was collected in AVL Buffer (19073, Qiagen) after 96 h incubation at 27°C, 37°C or 42°C. For temperature change experiments with MoLu Prim and MoBra Prim cells, incubation temperature was changed to 37°C after seven days of incubation at 27°C, while control cells were incubated continually at 27°C or 37°C, respectively. Supernatants were collected in AVL Buffer on day 0, 4, 7, 9, 11, 14, 16, 18 and 21. For cells with changing incubation temperature, sampling started on day 7. In an independent temperature change experiment with MoLu Prim cells over 21 days, temperature was changed from 27°C to 37°C (day 4), 37°C to 27°C (day 7), 27°C to 42°C (day 11), 42°C to 27°C (day 14) and 27°C to 37°C (day 18), including three washes with PBS on day 7 and 14, and the same sampling regimen as described above for temperature change experiments. In further infection experiments, we compared EBOV replication kinetics in MoLu Prim and MoBra Prim cells at 42°C with sampling on day 4 and 21, and MoKi_LT cells (MoKi Low Temperature, MoKi cells after 147 days at 27°C) and MoKi cells at 27°C, with sampling 1 h and 96 h after inoculation. Samples in AVL buffer were mixed with an equal volume of 100% ethanol before removing from BSL4 according to SOPs. Viral RNA was extracted using the QIAamp Kit (Qiagen) according to the manufacturer’s instructions. Viral RNAs were quantified by qRT-PCR (Applied Biosystems™ 7500) using the AgPath-ID™ One-Step RT-PCR Kit (4387391, Thermo Fisher). EBOV primers/probe and cycling conditions were described previously (42).
The nucleotide sequence of the L gene of MARV, strain Musoke, has been determined (43). The L gene has a single long open reading frame encoding a polypeptide of 2330 amino acids that represents the viral RNA-dependent RNA polymerase. For MARV qRT-PCR, primers/probe targeted the L gene
(forward: AAGCATTCCCTAGCAACATGATGGT; reverse: GTGAGGAGGGCTATAAAAGTCACTGACATG; probe: FAM-CCTATGCTTGCTGAATTGTGGTGCCA-BHQ1). For each PCR, 3 µl of RNA was added to 22 µl of master mix containing: 160 nM MARV-FW, 252 nM MARV-RV and 68 nM MARV-probe, 1 µl detection enhancer, 1 x RT-PCR buffer and 1 x RT-PCR enzyme mix. Samples were incubated for 15 min at 45°C, 10 min at 95°C followed by 45 cycles of 20 sec at 94°C and 30 sec at 59°C. Viral copy numbers for each sample were calculated from a standard curve, which was produced using EBOV/MARV in vitro transcripts (concentrations ranging from 101-107 copies).
Statistics
For statistical analyses we used the software R (version 4.1.2) and GraphPad Prism (version 9.1.0). The latter was used for figure visualization. Differences in replication rates were calculated as geometric mean with geometric standard deviations of triplicates in log10(x). Data normality was evaluated by visual inspection (e.g. qqplots) and the Shapiro-Wilk test. Data was log10(x) transformed and either Welch t-tests or Welch’s ANOVA were used to compare sample distribution of two or more independent groups (respectively). Games-Howell test was used as post-hoc analysis using Bonferroni for multiple comparison adjustment and reported as p-adjusted symbols. For paired samples, repeated-measures t-tests were used.
Confocal microscopy
NPC1 receptor expression levels in MoKi and MoKi_LT cells were characterized using confocal microscopy as described before (42).
Results
Wide fluctuations of ambient temperature in M. condylurus roost
To determine which temperatures are relevant to investigate the influence on cell growth and EBOV replication, temperatures inside the bat roosts were measured. In November 2016, ambient temperatures in south-eastern Ivory Coast fluctuated between 32°C during the day and 23°C during the night. Measured ambient temperatures were confirmed using weather history data (44). The temperatures in the roost of the bats, that the original cell cultures were derived from, varied between 42°C during the day and 25°C during the night, at 57-77% relative humidity (Figure 1). The corrugated metal roof likely contributed to a strong temperature increase in the bat roost during the day.
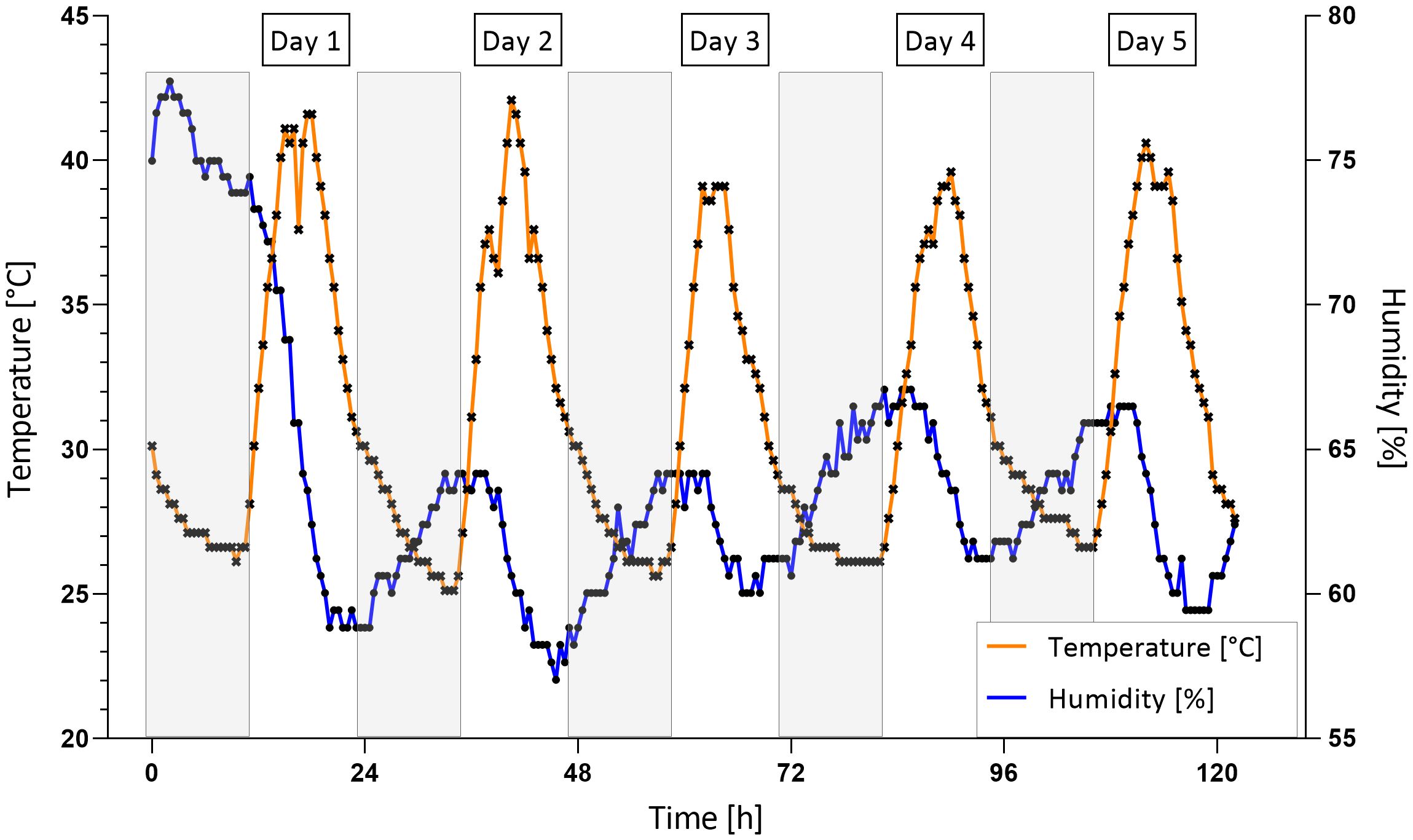
Figure 1. Ambient temperature and humidity in bat roost. Temperature (orange curve) and humidity (blue curve) were measured every 30 min for 5 days in a bat roost in Koffikro village, Ivory Coast. Grey boxes: Night phases (8 pm to 8 am).
Based on the local temperature in Ivory Coast, the measured temperatures in the bat roost and the special thermoregulation of M. condylurus bats, 27°C and 42°C were selected as relevant temperatures for our studies.
M. condylurus cells tolerate a broad range of temperatures
For cell growth and infection experiments with EBOV and MARV, we selected kidney, trachea, lung and brain cell cultures (MoKi, MoTra Prim, MoLu Prim and MoBra Prim) from M. condylurus, as well as HEK293, HeLa and Vero E6 cells as controls (Table 1).
All M. condylurus cells (Figure 2, red box) tolerated temperatures ranging from 27°C to 42°C without detachment. At 42°C, cells were enlarged in comparison to cells grown at 37°C. At 27°C only MoKi cells showed an altered morphology, with rounded cells. Temperature tolerance of MoTra Prim cells was tested at 17°C: After 96 h at this temperature, accumulations of cytoplasmic vesicles appeared (Supplementary Figure S1), while cell viability was determined to 91%. After passaging and incubation at 37°C for another 24 h, these intracellular vesicles disappeared.
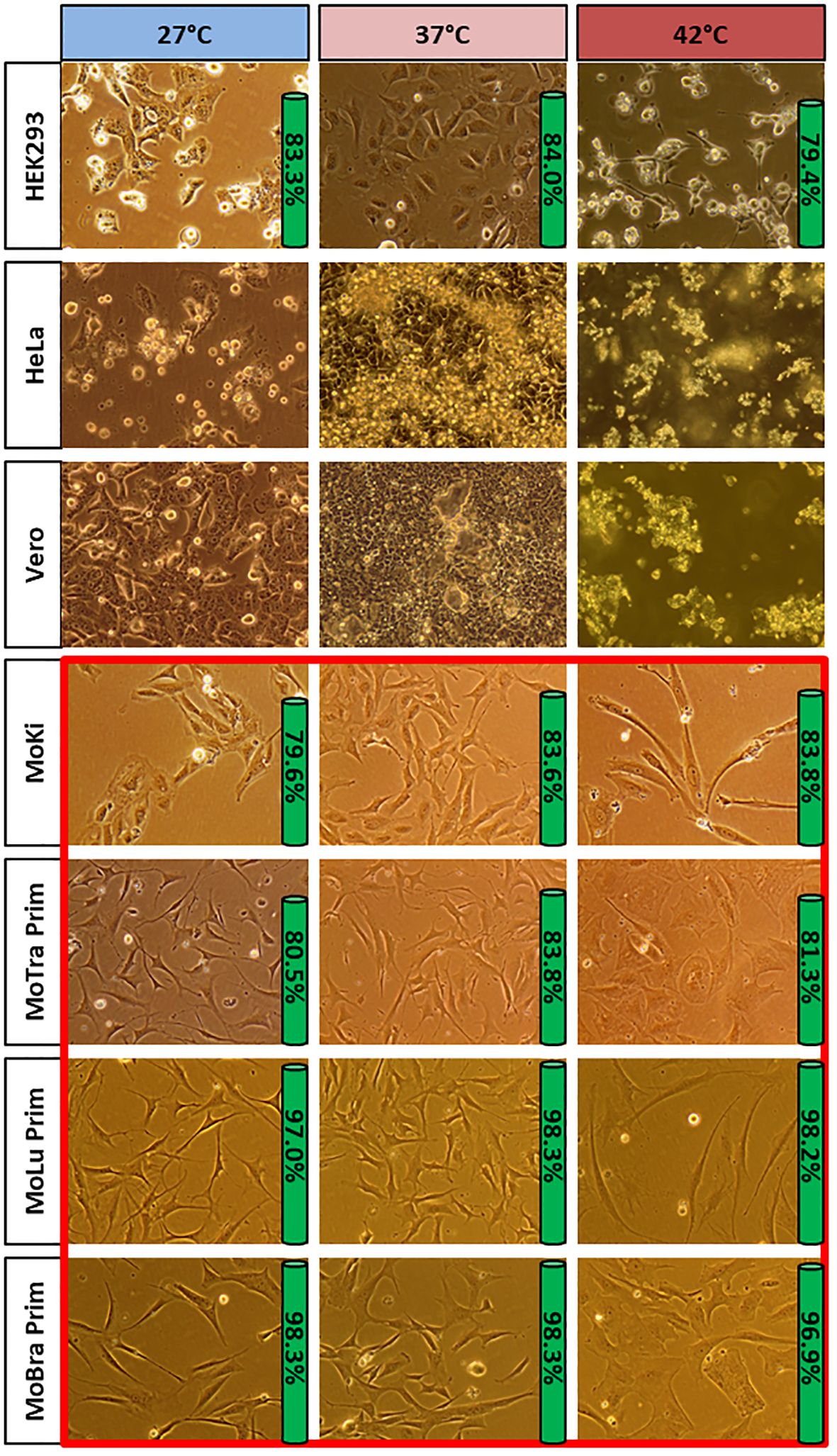
Figure 2. Cell growth of different cells at 27°C, 37°C and 42°C. All selected M. condylurus cells (red box) tolerated temperatures ranging from 27°C to 42°C without detachment. None of the selected control cells from other mammalians (HEK293, HeLa, Vero) tolerated 27°C or 42°C unaltered. Phase contrast microscopy: 400 x; 96 h incubation at tested temperature. Green bar: Viability of cell cultures (geometric means calculated from three wells).
None of the other mammalian cells (HEK293, HeLa and Vero) tolerated 27°C or 42°C unaltered: the majority of non-bat cells detached from the cell culture surface, and only few areas with adherent cells could be examined (Figure 2). In HeLa and Vero cell cultures, no adherent cells were observed after 96 h at 42°C. Vero cells did not detach at 27°C, but formed multinucleated syncytia.
Cell growth and viability of microbat and human cells at 27°C, 37°C and 42°C were observed for 96 h (Supplementary Figure S2). Most HEK293 cells were detached after 72 h at 27°C and 42°C. Therefore, also the HEK293 cells in suspension were sampled for determination of cell number and viability. Within 96 h, all cell cultures showed cell division at 27°C, 37°C and 42°C, with viability values between 80-97%. MoLu Prim and MoBra Prim cells showed the highest viability of all cell cultures at all temperatures. Although HEK293 cells lost adherence, they were viable within the tested period of time, and proliferated in suspension.
In summary, primary and immortalized cells from M. condylurus showed higher tolerance to lower and higher incubation temperatures than HEK293, HeLa and Vero cells. Cells remained adherent and showed high viability at 27°C, 37°C and 42°C.
Long-term adaptation and NPC1 receptor expression level changes at 27°C
Cloned MoKi cells were chosen to investigate possible temperature adaptations, because these cells show consistent and reproducible characteristics (42) without changes of NPC1 receptor expression levels through passaging. The cells were incubated at 27°C for 147 days, reached 100% confluence every 10-14 days and were passaged eleven times in total. The temperature-adapted cells were designated MoKi_LT (MoKi Low Temperature) shown in Supplementary Figure S3.
NPC1 receptor expression levels of MoKi and MoKi_LT cells were compared using confocal microscopy (Figure 3). MoKi_LT cells showed notably higher NPC1 receptor expression levels than MoKi cells, with highest amounts of NPC1 receptor in the regions of the nuclei (Figures 3C, D).
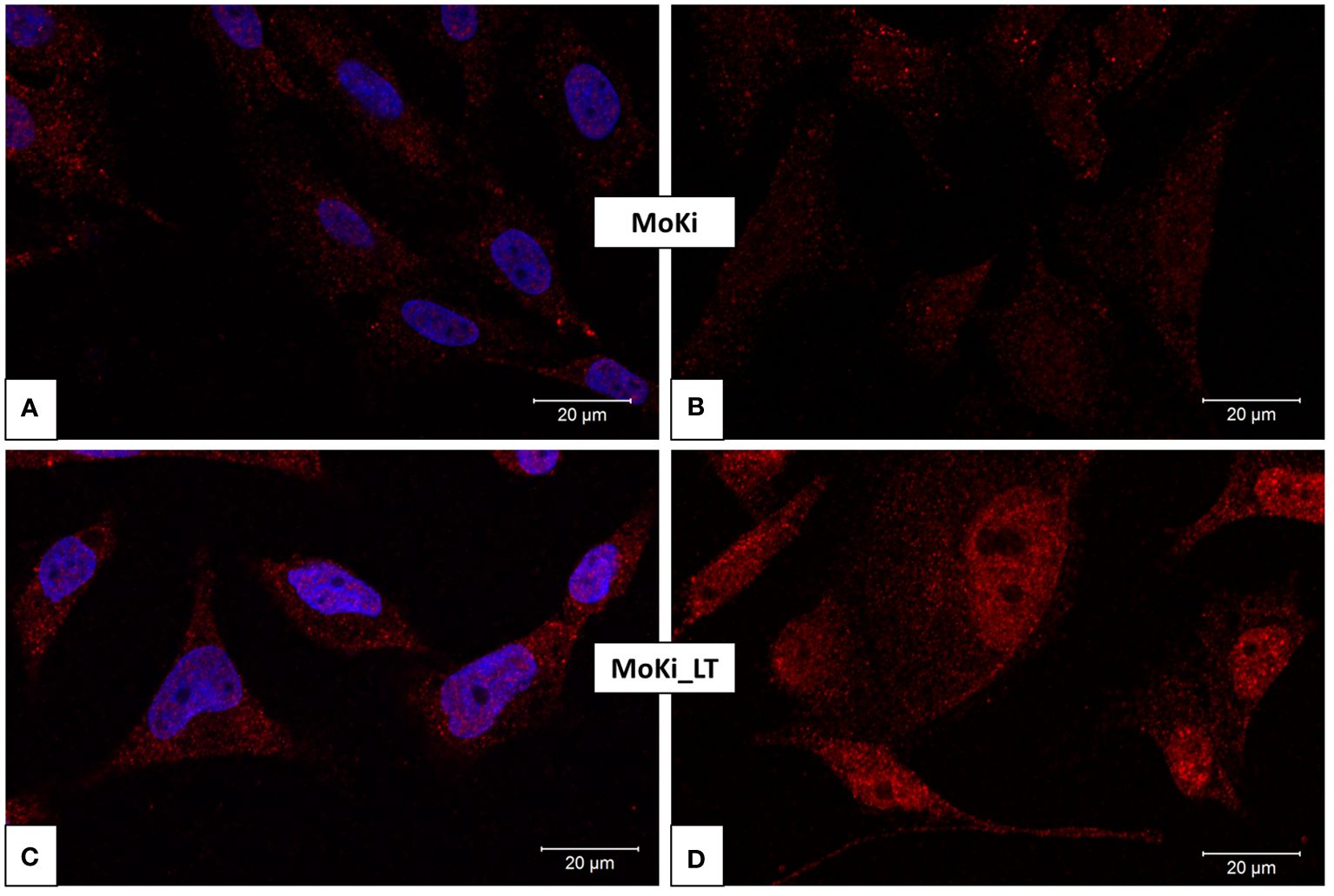
Figure 3. NPC1 receptor expression levels of MoKi and MoKi_LT cells. NPC1 receptor expression in MoKi cells (A, B) and MoKi cells after 147 days at 27°C (MoKi_LT, C, D). Stained NPC1 receptor (red) using primary mouse monoclonal antibodies against NPC1 (ab55706, abcam). Stained cell nuclei (blue) using ROTI Mount FluorCare DAPI (HP20.1, Carl Roth). B and D: without stained nuclei.
EBOV replication rates at constant temperatures of 27°C, 37°C or 42°C
HEK293, MoKi, MoBra Prim and MoLu Prim cells were incubated at 27°C, 37°C or 42°C after infection with EBOV for 96 h. To investigate, whether potential temperature effects on virus replication are also observed for other filoviruses, MoKi cells were infected with MARV.
The impact of temperature on filovirus replication is shown in Figure 4. Virus replication of EBOV and MARV was notably lower at 27°C compared to 37°C in all tested cell cultures. EBOV replication in HEK293 cells was significantly lower at 27°C and 42°C compared to 37°C. The replication at 42°C in MoKi cells was significantly higher than in HEK293 cells. Incubation temperatures of 42°C only led to a small reduction of EBOV replication efficiency in MoKi and MoBra Prim cells compared to HEK293 cells. EBOV replication in MoLu Prim cells at 42°C was on a similar low level as at 27°C or 37°C. Interestingly, the virus replication of MARV in MoKi cells at 42°C was significantly lower than for EBOV.
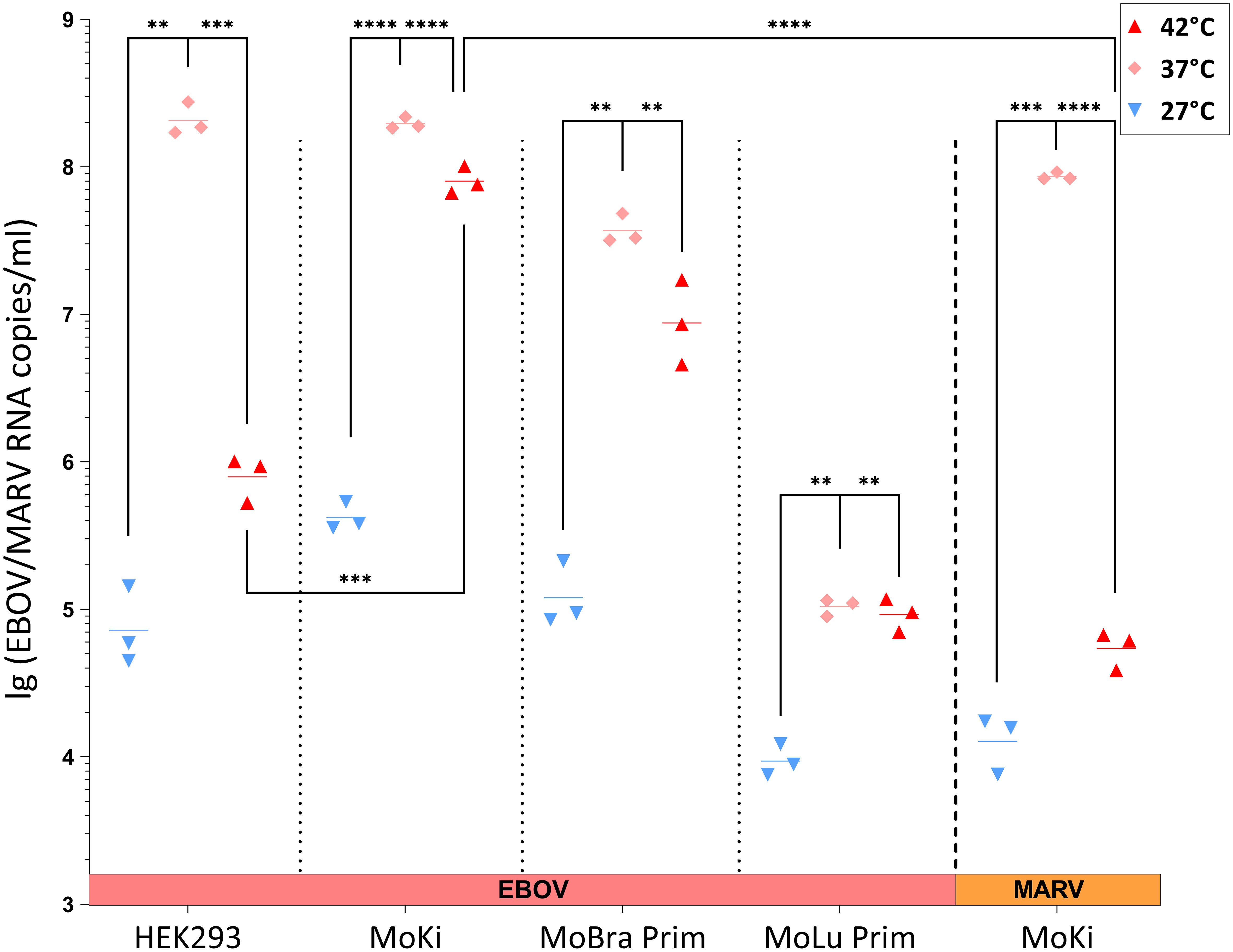
Figure 4. EBOV and MARV replication at different constant temperatures. Log-transformed viral RNA copy numbers/ml in supernatants of infected cell cultures after 96 h of incubation at 27°C (blue), 37°C (light red) or 42°C (dark red) determined by qRT-PCR. Infection with EBOV: HEK293, MoKi, MoBra Prim and MoLu Prim. Infection with MARV: MoKi. Horizontal lines indicate the geometric mean calculated from three replicates. Statistical analysis: Welch’s ANOVA followed by Games-Howell posthoc tests were used to compare EBOV replication in different temperature conditions per cell line. Welch two sample t-tests were used to compare replication at 42°C of EBOV (HEK293- and MoKi cells) and MARV (MoKi cells). Significant differences shown (**** = p ≤ 0.0001, *** = p ≤ 0.001, ** = p ≤ 0.01).
To investigate, whether temperature adaptations in MoKi_LT cells have an effect on EBOV replication, virus replication in these cells was compared to replication in MoKi cells (Supplementary Figure S4). EBOV replication rates in MoKi_LT cells at 27°C were slightly higher than in MoKi cells.
High impact of temperature changes on EBOV replication kinetics
We further investigated in MoLu Prim and MoBra Prim cells, whether a temperature change to 37°C after seven days incubation at 27°C results in increased EBOV replication. Temperature changes had a strong impact on EBOV replication (Figure 5). Virus replication in MoLu Prim cells incubated at 27°C was markedly lower than at 37°C, with 2.9 x 106 compared to 1.7 x 108 EBOV RNA copies/ml, respectively, at day 21 post-infection. Virus replication in MoBra Prim cells was generally higher than in MoLu Prim cells, reaching a similar level of 5.7 x 108 EBOV RNA copies/ml for both temperatures between day 16 and 18. A change of incubation temperature from 27°C to 37°C on day 7 resulted in increases of virus replication in MoLu Prim and MoBra Prim cells, compared to control cells, incubated continuously at 27°C (Figure 5, dashed line).
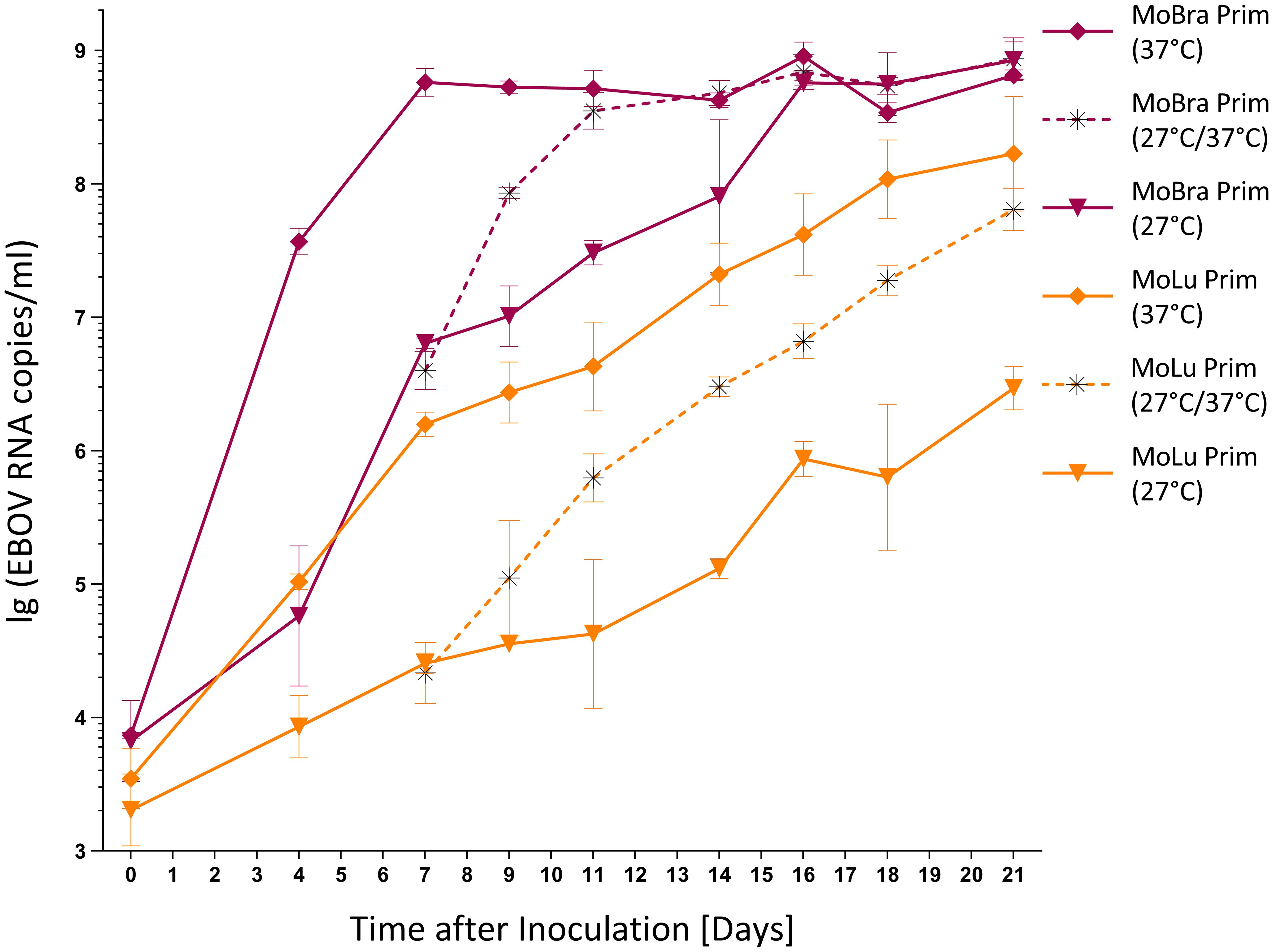
Figure 5. Impact of temperature changes on EBOV replication kinetics. Log-transformed viral RNA copy numbers/ml in supernatants of infected cells determined by qRT-PCR (geometric means with geometric standard deviations calculated from three wells). Incubation of MoLu Prim (orange) and MoBra Prim cells (violet): continuously at 27°C (triangle), continuously at 37°C (rhombus), temperature change from 27°C to 37°C on day 7. (asterisk, dashed line).
For comparison of virus replication rates the fold-amplification of viral RNAs between day 7 and 11 (96 h) post-infection was determined (Table 2).
At a constant incubation temperature of 27°C, the fold-amplification of EBOV RNA copies was log10(x)=0.22 in MoLu Prim cells and log10(x)=0.68 in MoBra Prim cells. The increase of incubation temperature to 37°C resulted in a fold-amplification to log10(x)=1.46 for MoLu Prim and log10(x)=1.95 for MoBra Prim cells.
The increase in incubation temperature from 27°C to 37°C also resulted in increased virus replication rates in EBOV- as well as MARV-infected MoKi cells (data not shown). However, MARV-infected MoKi cells did not tolerate the temperature switch as well as EBOV-infected cells, and detached completely from the cell culture surface. HEK293 cells did not tolerate incubation at 27°C, and most cells detached by day 4 post-infection with EBOV. After the temperature switch from 27°C to 37°C, all HEK293 cells detached and an increase of virus replication could not be observed.
In contrast, MoLu Prim and MoBra Prim cells cultured for 21 days post-infection with EBOV at 27°C, 37°C (Figure 5) or 42°C (Supplementary Figure S5), remained adherent and virus replication could be measured at all temperatures. Even after changing incubation temperatures five times (Supplementary Figure S6), cells remained adherent and divided even after day 21 in culture.
Discussion
M. condylurus bats are highly tolerant to variations in ambient temperatures and are therefore capable of utilizing a broad range of climatic and environmental conditions using adaptive thermoregulation (1, 3, 4). This temperature tolerance in vivo translated to cell cultures derived from M. condylurus in vitro. In contrast to other mammalian cells, M. condylurus cells tolerated temperatures from 27°C to 42°C for up to 21 days, without morphological changes in primary cells at 27°C, and a slight change towards enlarged cells at 42°C. The cells remained adherent and showed uniformly high viabilities. Cell enumeration and viability testing of HEK293 cells revealed, that cells were still viable and proliferated until the end of the experiment. However, detachment of cells and disruption of cell-matrix interactions induces apoptosis (anoikis) (45–47), so that cell death of HEK293 cells in suspension can be assumed. Vero cells remained adherent at 27°C, but formed multinucleated syncytia, and detached at 42°C, showing that these cells were not tolerant to the same temperature range as M. condylurus cells.
Few studies have reported experimental data with bat cells incubated at temperatures above 37°C. In the HypNi/1.1 cell line derived from Hypsignathus monstrosus, the mean percentage of viable cells declined to below 20% after 72 h at 41°C, while the REO5 cell line from Rousettus aegyptiacus tolerated this incubation temperature (12).
While HEK293 cells showed significantly lower EBOV replication rates at 42°C as compared to 37°C, little or no reduction of EBOV replication was observed for all tested cell cultures from M. condylurus. Upon infection with EBOV and incubation at 37°C or 41°C respectively, only small differences in virus replication rates had also been observed in HypNi/1.1 and RE06 cells (12). Another study showed, that incubating several human cell types at a febrile temperature of 39.5°C markedly reduced viral replication of adenovirus type 5 (Ad5) (10). The tolerance of bat cells, but not human cells, to high temperatures might explain these observations. At 96 h post-infection of HEK293 cells, most cells were detached and possibly several cellular components and processes that are essential for virus replication were lacking.
Also, EBOV replicated constantly at higher temperatures for periods of up to 21 days. For HPAIV, heat inactivation of the viral RNA polymerase was observed at temperatures above 38°C, although avian viruses are adapted to higher temperatures (11). Compared to this, the viral RNA polymerase and other viral components required for EBOV replication were highly tolerant to high temperatures. In contrast, the MARV replication rate at 42°C in MoKi cells was significantly lower than the EBOV replication rate, so that a lower temperature tolerance of MARV (at least in MoKi cells) may be assumed. In M. condylurus as a potential natural reservoir, EBOV would frequently be exposed to high body temperatures in vivo: in the hot roosts during the day and during flight at night. Although not observed in vitro, the fever-like temperatures for humans during flight and in the hot roosts of M. condylurus bats in vivo (7) might contribute to controlled virus replication by increased metabolic rates and immune responses (9). Because reservoir hosts and their viruses coevolve, tolerance to high temperatures of all cell cultures from M. condylurus and temperature tolerance of EBOV, but not MARV, provides additional evidence, that EBOV and M. condylurus bats coevolved and that this bat species is likely to be a natural reservoir for ebolaviruses.
The role of torpor in infection dynamics of pathogens is largely unstudied (48). At low body temperatures during torpor or hibernation in bats, low virus replication rates of Japanese encephalitis virus (JEV) and rabies virus (RABV) were observed (13–15). For HPAIV, a significant inhibition of virus replication was detected for temperatures below 34°C in chicken fibroblast cells (11). The impact of low temperatures on virus replication kinetics of filoviruses has not been described previously. Virus replication rates of EBOV and MARV in all cell cultures at 27°C were noticeably lower than at 37°C. M. condylurus bats are thermolabile, with daily bouts of torpor during winter and summer, with body temperatures closely conforming to ambient temperatures (3). An incubation temperature of 27°C can be assumed as bat body temperature for ambient temperatures between 20-25°C (4). At a 27°C body temperature or lower, we expect reduced virus replication rates in these bats. Most biological reactions proceed with a temperature coefficient (Q10) ∼ 2 or 3, so that with every 10°C increase in temperature, the reaction rate approximately doubles or triples (49). The reduction of body temperature from 37°C to 27°C presumably results in significant reductions of metabolic rates in M. condylurus, which can also have broad effects on the immune functions of the bats (50). Reduced body temperatures and metabolic rates may suppress immune responses and delay viral clearance from bats (5, 6).
The significance of NPC1 in the endolysosomal membrane of MoKi cells for EBOV infection was recently confirmed (51). In MoKi cells adapted to 27°C for 21 weeks (MoKi_LT) we observed higher NPC1 receptor expression levels compared to MoKi cells at 37°C. At low temperatures, many animals reduce the cholesterol content of the plasma membrane (52), to ensure fluidity for cellular functions (53, 54). The intracellular cholesterol transport between plasma membranes, the endoplasmic reticulum (ER), Golgi apparatus and other organelles is complex and mediated by carrier-mediated diffusion, transport vesicles or membrane contacts (55). Cholesterol can be internalized from the plasma membrane, reach endosomes by endocytosis, transported to the ER or back to the plasma membrane (56). With the current data we can only speculate about the accumulation of NPC1 in the nuclear region. One hypothesis is that higher NPC1 receptor expression levels in MoKi_LT cells, especially in the nuclear regions, might indicate an enhanced cholesterol transport to the ER via the endo-lysosomal pathway at lower temperatures. Pharmacologically induced endolysosomal cholesterol imbalance was recently shown to impair EBOV infection in MoKi cells (51). Another hypothesis is, that increased NPC1 receptor expression levels at 27°C and potentially changing cholesterol levels in the endolysosomal system might also be evolved mechanisms in M. condylurus cells to facilitate a sufficient level of EBOV replication when bat’s body temperatures are low. Although NPC1 receptor expression levels in MoKi_LT cells were noticeably increased, only a small increase in EBOV replication compared to MoKi cells was detected, which might be reasoned with generally low EBOV replication at 27°C. How quick NPC1 receptor expression levels might adapt to changing temperatures and potentially influence EBOV replication kinetics, should be addressed in future studies.
Upon experimental inoculation of RABV in insectivorous bats of the species Tadarida brasiliensis and Myotis lucifugus, little or no virus replication was detected at low body temperatures (at 5°C or 10°C ambient temperature) (15). When inoculated animals were transferred to 29°C, virus replicated and reached detectable levels in several tissues (15). To investigate, if EBOV can persist for longer periods at low temperatures and if temperature-dependent reactivations with high virus replication rates can be observed, we performed experiments with changing incubation temperatures. At 27°C, the EBOV replication was detectable, but low. Increasing the temperature from 27°C to 37°C resulted in temperature-dependent increases of EBOV replication in all cell cultures from M. condylurus. Heterothermy of M. condylurus with varying body temperatures might result in temporarily limited virus replication capacities in these bats. Torpor typically reduces virus replication rates, lengthens incubation periods and provides an efficient mechanism for overwintering of some infections (48). With recorded body temperatures in M. condylurus bats as low as 12°C in winter (3), presumably no, or only very little, virus replication can occur in some seasons of the year. At 17°C, we observed the formation of large amounts of intracellular vesicles in primary trachea cells from M. condylurus, which disappeared after passaging and incubation at 37°C. Further investigations have to unravel the nature and function of these vesicles. The presence of many vesicles can result in a reduced volume of cytoplasm and thereby increase the cell-surface-to-volume ratio, which might help cells to survive under stress conditions (57). If EBOV inclusion bodies, which are the cytoplasmic sites of nucleocapsid formation and RNA replication in the virus life cycle (58–60), can form under these conditions or if the high quantity of intracellular vesicles prevents EBOV replication completely at 17°C has to be investigated in future studies.
In summary, M. condylurus cells were highly tolerant to a range of incubation temperatures, while other mammalian cells did not tolerate such a broad temperature range beyond 37°C, and predominantly detached from the cell culture surface. We observed a high impact of temperature on filovirus replication kinetics with a strong reduction of EBOV replication efficiency in all cells at 27°C. We further observed higher NPC1 receptor expression levels in cells from M. condylurus incubated long-term at lower temperatures, which was shown to correlate with higher EBOV replication rates. Temperature tolerance and relatively high replication rates for EBOV, but not MARV, provides additional evidence, that EBOV and M. condylurus bats coevolved and that this bat species is a reservoir host for ebolaviruses, but not MARV. Fluctuations in the body temperature of M. condylurus bats could be a potential key factor for tolerance to EBOV infection in vivo and might be involved in balancing the level of virus replication and establishing viral persistence in these bats. To investigate the significance of these temperature effects in vivo and to determine the general role of these bats for the ecology of ebolaviruses, experimental infections of M. condylurus bats are required.
Data availability statement
The original contributions presented in the study are included in the article/Supplementary material. Further inquiries can be directed to the corresponding authors.
Ethics statement
Ethical approval was not required for the studies on humans in accordance with the local legislation and institutional requirements because only commercially available established cell lines were used. The animal study was approved by Laboratoire Central Vétérinair, Laboratoire National d’Appui au Développement Agricole (LANADA), Bingerville, Côte d’Ivoire (No. 05/virology/2016). The animal care and use protocol adhered with the Ethics Committee of LANADA and National Ethics Committee for the Research (CNER). Consent existed to measure the temperature in the bat roost from the owners of the residence in Koffikro Village. The study was conducted in accordance with the local legislation and institutional requirements.
Author contributions
MB: Conceptualization, Formal analysis, Funding acquisition, Investigation, Methodology, Visualization, Writing – original draft, Writing – review & editing. SR-S: Investigation, Formal analysis, Methodology, Writing – review & editing. AL: Investigation, Methodology, Writing – review & editing. AW: Investigation, Methodology, Writing – review & editing. MG: Resources, Writing – review & editing. AB-B: Resources, Writing – review & editing. EC-H: Resources, Writing – review & editing. JP: Conceptualization, Formal analysis, Investigation, Methodology, Writing – original draft, Writing – review & editing. AK: Conceptualization, Formal analysis, Funding acquisition, Investigation, Methodology, Project administration, Resources, Supervision, Visualization, Writing – original draft, Writing – review & editing.
Funding
The author(s) declare financial support was received for the research, authorship, and/or publication of this article. This publication was financially supported by the RKI. Funding was partially also provided by Elsa-Neumann Scholarship from the state of Berlin, Germany (MB).
Acknowledgments
We thank Uwe Vogel, Katharina Hansen-Kant and Norman Kirchoff (RKI) for technical assistance. The authors are very grateful to Allison Groseth and Thomas Hoenen (FLI) for helpful discussions.
Conflict of interest
The authors declare that the research was conducted in the absence of any commercial or financial relationships that could be construed as a potential conflict of interest.
Publisher’s note
All claims expressed in this article are solely those of the authors and do not necessarily represent those of their affiliated organizations, or those of the publisher, the editors and the reviewers. Any product that may be evaluated in this article, or claim that may be made by its manufacturer, is not guaranteed or endorsed by the publisher.
Supplementary material
The Supplementary Material for this article can be found online at: https://www.frontiersin.org/articles/10.3389/fviro.2024.1392583/full#supplementary-material
References
1. Roxburgh L, Raimondo D, Page-Nicholson S, Relton C. The red list of mammals of South Africa, swaziland and Lesotho. South Africa: South African National Biodiversity Institute and Endangered Wildlife Trust (2016).
2. Czenze Z, Dunbar M. Hot bats go cold: Heterothermy in neotropical bats. Can J Zool. (2017) 95:909–12. doi: 10.1139/cjz-2016-0318
3. Vivier L, van der Merwe M. The incidence of torpor in winter and summer in the Angolan free-tailed bat, Mops condylurus (Microchiroptera: Molossidae), in a subtropical environment, Mpumulanga, South Africa. Afr Zool. (2007) 42:50–8. doi: 10.3377/1562-7020(2007)42[50:TIOTIW]2.0.CO;2
4. Maloney SK, Bronner GN, Buffenstein R. Thermoregulation in the Angolan free-tailed bat Mops condylurus: A small mammal that uses hot roosts. Physiol Biochem Zool. (1999) 72:385–96. doi: 10.1086/316677
5. Han HJ, Wen HL, Zhou CM, Chen FF, Luo LM, Liu JW, et al. Bats as reservoirs of severe emerging infectious diseases. Virus Res. (2015) 205:1–6. doi: 10.1016/j.virusres.2015.05.006
6. Wang LF, Walker PJ, Poon LL. Mass extinctions, biodiversity and mitochondrial function: are bats ‘special’ as reservoirs for emerging viruses? Curr Opin Virol. (2011) 1:649–57. doi: 10.1016/j.coviro.2011.10.013
7. Bronrier GN, Maloney SK, Buffenstein R. Survival tactics within thermally-challenging roosts: heat tolerance and cold sensitivity in the Angolan free-tailed bat, Mops condylurus. S Afr J Zool. (1999) 34:1–10. doi: 10.1080/02541858.1999.11448481
8. Monadjem ATPJ, Cotterill FPD, Schoeman MC. Bats of southern and central africa - A biogeographic and taxonomic synthesis. Johannesburg: Wits University Press (2010).
9. O’Shea TJ, Cryan PM, Cunningham AA, Fooks AR, Hayman DT, Luis AD, et al. Bat flight and zoonotic viruses. Emerg Infect Dis. (2014) 20:741–5. doi: 10.3201/eid2005.130539
10. Thorne SH, Brooks G, Lee Y-L, Au T, Eng LF, Reid T. Effects of febrile temperature on adenoviral infection and replication: implications for viral therapy of cancer. J Virol. (2005) 79:581. doi: 10.1128/JVI.79.1.581-591.2005
11. Scholtissek C, Rott R. Effect of temperature on the multiplication of an Influenza virus. J Gen Virol. (1969) 5:283–90. doi: 10.1099/0022-1317-5-2-283
12. Miller MR, McMinn RJ, Misra V, Schountz T, Muller MA, Kurth A, et al. Broad and temperature independent replication potential of filoviruses on cells derived from old and new world bat species. J Infect Dis. (2016) 214(Suppl 3):S297–302. doi: 10.1093/infdis/jiw199
13. George DB, Webb CT, Farnsworth ML, O’Shea TJ, Bowen RA, Smith DL, et al. Host and viral ecology determine bat rabies seasonality and maintenance. Proc Natl Acad Sci U S A. (2011) 108:10208–13. doi: 10.1073/pnas.1010875108
14. La Motte LCJ. Japanese B encephalitis in bats during simulated hibernation 1. Am J Epidemiol. (1958) 67:101–8. doi: 10.1093/oxfordjournals.aje.a119912
15. Sulkin SE, Allen R, Sims R, Krutzsch PH, Kim C. Studies on the pathogenesis of rabies in insectivorous bats: II. Influence of environmental temperature. J Exp Med. (1960) 112:595–617. doi: 10.1084/jem.112.4.595
16. Schuh AJ, Amman BR, Towner JS. Filoviruses and bats. Microbiol Aust. (2017) 38:12–6. doi: 10.1071/MA17005
17. Olson SH, Reed P, Cameron KN, Ssebide BJ, Johnson CK, Morse SS, et al. Dead or alive: animal sampling during Ebola hemorrhagic fever outbreaks in humans. Emerg Health Threats J. (2012) 5. doi: 10.3402/ehtj.v5i0.9134
18. Mari Saez A, Weiss S, Nowak K, Lapeyre V, Zimmermann F, Dux A, et al. Investigating the zoonotic origin of the West African Ebola epidemic. EMBO Mol Med. (2015) 7:17–23. doi: 10.15252/emmm.201404792
19. Arata AA, Johnson B. Approaches towards studies on potential reservoirs of viral haemorrhagic fever in southern Sudan (1977). Elsevier/Netherland Biomedical (1978).
20. Leroy EM, Kumulungui B, Pourrut X, Rouquet P, Hassanin A, Yaba P, et al. Fruit bats as reservoirs of Ebola virus. Nature. (2005) 438:575–6. doi: 10.1038/438575a
21. Pourrut X, Delicat A, Rollin PE, Ksiazek TG, Gonzalez JP, Leroy EM. Spatial and temporal patterns of Zaire ebolavirus antibody prevalence in the possible reservoir bat species. J Infect Dis. (2007) 196 Suppl 2:S176–83. doi: 10.1086/520541
22. Pourrut X, Souris M, Towner JS, Rollin PE, Nichol ST, Gonzalez JP, et al. Large serological survey showing cocirculation of Ebola and Marburg viruses in Gabonese bat populations, and a high seroprevalence of both viruses in Rousettus aEgyptiacus. BMC Infect Dis. (2009) 9:159. doi: 10.1186/1471-2334-9-159
23. Hayman DT, Yu M, Crameri G, Wang LF, Suu-Ire R, Wood JL, et al. Ebola virus antibodies in fruit bats, Ghana, West Africa. Emerg Infect Dis. (2012) 18:1207–9. doi: 10.3201/eid1807.111654
24. Hayman DT, Emmerich P, Yu M, Wang LF, Suu-Ire R, Fooks AR, et al. Long-term survival of an urban fruit bat seropositive for Ebola and Lagos bat viruses. PloS One. (2010) 5:e11978. doi: 10.1371/journal.pone.0011978
25. Ogawa H, Miyamoto H, Nakayama E, Yoshida R, Nakamura I, Sawa H, et al. Seroepidemiological prevalence of multiple species of filoviruses in fruit bats (Eidolon helvum) migrating in africa. J Infect Dis. (2015) 212(Suppl. 2):S101–8. doi: 10.1093/infdis/jiv063
26. Yuan J, Zhang Y, Li J, Zhang Y, Wang LF, Shi Z. Serological evidence of ebolavirus infection in bats, China. Virol J. (2012) 9:236. doi: 10.1186/1743-422X-9-236
27. Olival KJ, Islam A, Yu M, Anthony SJ, Epstein JH, Khan SA, et al. Ebola virus antibodies in fruit bats, Bangladesh. Emerg Infect Dis. (2013) 19:270–3. doi: 10.3201/eid1902.120524
28. Jayme SI, Field HE, de Jong C, Olival KJ, Marsh G, Tagtag AM, et al. Molecular evidence of Ebola Reston virus infection in Philippine bats. Virol J. (2015) 12:107. doi: 10.1186/s12985-015-0331-3
29. Leendertz SA, Gogarten JF, Dux A, Calvignac-Spencer S, Leendertz FH. Assessing the evidence supporting fruit bats as the primary reservoirs for ebola viruses. EcoHealth. (2015) 13:18–25. doi: 10.1007/s10393-015-1053-0
30. Goldstein T, Anthony SJ, Gbakima A, Bird BH, Bangura J, Tremeau-Bravard A, et al. The discovery of Bombali virus adds further support for bats as hosts of ebolaviruses. Nat Microbiol. (2018) 3:1084–89. doi: 10.1038/s41564-018-0227-2
31. Forbes KM, Webala PW, Jaaskelainen AJ, Abdurahman S, Ogola J, Masika MM, et al. Bombali virus in mops condylurus bat, Kenya. Emerg Infect Dis. (2019) 25:955–7. doi: 10.3201/eid2505.181666
32. Karan LS, Makenov MT, Korneev MG, Sacko N, Boumbaly S, Yakovlev SA, et al. Bombali virus in mops condylurus bats, Guinea. Emerg Infect Dis. (2019) 25:1774–5. doi: 10.3201/eid2509.190581
33. Swanepoel R, Leman PA, Burt FJ, Zachariades NA, Braack LE, Ksiazek TG, et al. Experimental inoculation of plants and animals with Ebola virus. Emerg Infect Dis. (1996) 2:321–5. doi: 10.3201/eid0204.960407
34. Riesle-Sbarbaro SA, Wibbelt G, Düx A, Kouakou V, Bokelmann M, Hansen-Kant K, et al. Selective replication and vertical transmission of Ebola virus in experimentally infected Angolan free-tailed bats. Nat Commun. (2024) 15:925. doi: 10.1038/s41467-024-45231-0
35. Bokelmann M, Vogel U, Debeljak F, Düx A, Riesle-Sbarbaro S, Lander A, et al. Tolerance and persistence of ebola virus in primary cells from mops condylurus, a potential ebola virus reservoir. Viruses. (2021) 13:2186. doi: 10.3390/v13112186
36. Hofmann-Winkler H, Kaup F, Pohlmann S. Host cell factors in filovirus entry: novel players, new insights. Viruses. (2012) 4:3336–62. doi: 10.3390/v4123336
37. Cote M, Misasi J, Ren T, Bruchez A, Lee K, Filone CM, et al. Small molecule inhibitors reveal Niemann-Pick C1 is essential for Ebola virus infection. Nature. (2011) 477:344–8. doi: 10.1038/nature10380
38. Haines KM, Vande Burgt NH, Francica JR, Kaletsky RL, Bates P. Chinese hamster ovary cell lines selected for resistance to ebolavirus glycoprotein mediated infection are defective for NPC1 expression. Virology. (2012) 432:20–8. doi: 10.1016/j.virol.2012.05.018
39. Herbert AS, Davidson C, Kuehne AI, Bakken R, Braigen SZ, Gunn KE, et al. Niemann-pick C1 is essential for ebolavirus replication and pathogenesis in vivo. MBio. (2015) 6:e00565–15. doi: 10.1128/mBio.00565-15
40. Carette JE, Raaben M, Wong AC, Herbert AS, Obernosterer G, Mulherkar N, et al. Ebola virus entry requires the cholesterol transporter Niemann-Pick C1. Nature. (2011) 477:340–3. doi: 10.1038/nature10348
41. Yang XL, Tan CW, Anderson DE, Jiang RD, Li B, Zhang W, et al. Characterization of a filovirus (Mengla virus) from Rousettus bats in China. Nat Microbiol. (2019) 4:390–395. doi: 10.1038/s41564-019-0398-5
42. Bokelmann M, Edenborough K, Hetzelt N, Kreher P, Lander A, Nitsche A, et al. Utility of primary cells to examine NPC1 receptor expression in Mops condylurus, a potential Ebola virus reservoir. PloS Negl Trop Dis. (2020) 14:e0007952. doi: 10.1371/journal.pntd.0007952
43. Mühlberger E, Sanchez A, Randolf A, Will C, Kiley MP, Klenk HD, et al. The nucleotide sequence of the L gene of Marburg virus, a filovirus: homologies with paramyxoviruses and rhabdoviruses. Virology. (1992) 187:534–47. doi: 10.1016/0042-6822(92)90456-Y
44. timeanddate. Past Weather in Abidjan, Cote d’Ivoire (Ivory Coast) — November 2016 (2022). Available online at: https://www.timeanddate.com.
45. Frisch SM, Francis H. Disruption of epithelial cell-matrix interactions induces apoptosis. J Cell Biol. (1994) 124:619–26. doi: 10.1083/jcb.124.4.619
46. Bretland AJ, Lawry J, Sharrard RM. A study of death by anoikis in cultured epithelial cells. Cell Prolif. (2001) 34:199–210. doi: 10.1046/j.1365-2184.2001.00198.x
47. Ma X-K, Wang L, Li Y, Yang X-M, Zhao P, Hao T, et al. HAb18G/CD147 cell-cell contacts confer resistance of a HEK293 subpopulation to anoikis in an E-cadherin-dependent manner. BMC Cell Biol. (2010) 11:27–. doi: 10.1186/1471-2121-11-27
48. Hayman DTS, Bowen RA, Cryan PM, McCracken GF, O’Shea TJ, Peel AJ, et al. Ecology of zoonotic infectious diseases in bats: current knowledge and future directions. Zoonoses Public Health. (2013) 60:2–21. doi: 10.1111/zph.12000
49. Reyes BA, Pendergast JS, Yamazaki S. Mammalian peripheral circadian oscillators are temperature compensated. J Biol Rhythms. (2008) 23:95–8. doi: 10.1177/0748730407311855
50. Stockmaier S, Dechmann DK, Page RA, O’Mara MT. No fever and leucocytosis in response to a lipopolysaccharide challenge in an insectivorous bat. Biol Lett. (2015) 11. doi: 10.1098/rsbl.2015.0576
51. Kummer S, Lander A, Goretzko J, Kirchoff N, Rescher U, Schloer S. Pharmacologically induced endolysosomal cholesterol imbalance through clinically licensed drugs itraconazole and fluoxetine impairs Ebola virus infection in vitro. Emerg Microbes Infect. (2022) 11:195–207. doi: 10.1080/22221751.2021.2020598
52. Hassett RP, Crockett EL. Habitat temperature is an important determinant of cholesterol contents in copepods. J Exp Biol. (2009) 212:71–7. doi: 10.1242/jeb.020552
53. Crockett EL. Cholesterol function in plasma membranes from ectotherms: Membrane-specific roles in adaptation to temperature. Am Zool. (1998) 38:291–304. doi: 10.1093/icb/38.2.291
54. Cooper GM. The cell: A molecular approach - structure of the plasma membrane. 2nd edition. Sunderland, USA: Sinauer Associates Inc (2000). 689 p.
55. Maxfield FR, Wustner D. Intracellular cholesterol transport. J Clin Invest. (2002) 110:891–8. doi: 10.1172/JCI200216500
56. Simons K, Ikonen E. How cells handle cholesterol. Science. (2000) 290:1721–6. doi: 10.1126/science.290.5497.1721
57. Pfeifer F. Distribution, formation and regulation of gas vesicles. Nat Rev Microbiol. (2012) 10:705–15. doi: 10.1038/nrmicro2834
58. Miyake T, Farley CM, Neubauer BE, Beddow TP, Hoenen T, Engel DA. Ebola virus inclusion body formation and RNA synthesis are controlled by a novel domain of nucleoprotein interacting with VP35. J Virol. (2020) 94. doi: 10.1128/JVI.02100-19
59. Hoenen T, Shabman RS, Groseth A, Herwig A, Weber M, Schudt G, et al. Inclusion bodies are a site of ebolavirus replication. J Virol. (2012) 86:11779–88. doi: 10.1128/JVI.01525-12
Keywords: Ebola virus, reservoir host, bat, temperature, virus replication, tolerance
Citation: Bokelmann M, Riesle-Sbarbaro SA, Lander A, Wahlbrink A, Groschup MH, Balkema-Buschmann A, Couacy-Hymann E, Prescott J and Kurth A (2024) Temperature as a potential regulator for Ebola virus replication in primary cells from Mops condylurus. Front. Virol. 4:1392583. doi: 10.3389/fviro.2024.1392583
Received: 27 February 2024; Accepted: 22 July 2024;
Published: 09 August 2024.
Edited by:
Jesus Miguel Torres Flores, National Polytechnic Institute, MexicoReviewed by:
Vishal Srivastava, Cleveland Clinic, United StatesMaia Kavanagh Williamson, LifeArc, United Kingdom
Copyright © 2024 Bokelmann, Riesle-Sbarbaro, Lander, Wahlbrink, Groschup, Balkema-Buschmann, Couacy-Hymann, Prescott and Kurth. This is an open-access article distributed under the terms of the Creative Commons Attribution License (CC BY). The use, distribution or reproduction in other forums is permitted, provided the original author(s) and the copyright owner(s) are credited and that the original publication in this journal is cited, in accordance with accepted academic practice. No use, distribution or reproduction is permitted which does not comply with these terms.
*Correspondence: Marcel Bokelmann, Qm9rZWxtYW5uTUBya2kuZGU=; Andreas Kurth, S3VydGhBQHJraS5kZQ==