- 1Division of Virology, Institute of Medical Science, University of Tokyo, Tokyo, Japan
- 2The Research Center for Global Viral Diseases, National Center for Global Health and Medicine Research Institute, Tokyo, Japan
- 3Otsu Nutraceuticals Research Institute, Nutraceuticals Division, Otsuka Pharmaceutical Co., Ltd., Shiga, Japan
- 4Influenza Research Institute, Department of Pathobiological Sciences, School of Veterinary Medicine, University of Wisconsin-Madison, Madison, WI, United States
- 5The University of Tokyo, Pandemic Preparedness, Infection and Advanced Research Center, Tokyo, Japan
Severe acute respiratory syndrome coronavirus 2 (SARS-CoV-2) is responsible for the ongoing coronavirus pandemic. Besides vaccines and antiviral drugs, probiotics have attracted attention for prevention of SARS-CoV-2 infection. Here, we examined the efficacy of heat-killed Lactiplantibacillus pentosus ONRICb0240 (b240) against SARS-CoV-2 infection in mice. We observed that oral intake of heat-killed b240 did not affect virus titers in the respiratory organs of SARS-CoV-2-infected mice, but did provide partial protection against SARS-CoV-2 infection. In addition, heat-killed b240 treatment suppressed the expression of IL-6, a key proinflammatory cytokine, on Day 2 post-infection. Our results highlight the promising protective role of heat-killed b240 and suggest a possible mechanism by which heat-killed b240 partially protects against SARS-CoV-2 infection by modulating host responses.
Introduction
Coronavirus disease 2019 (COVID-19), which is caused by severe acute respiratory syndrome coronavirus 2 (SARS-CoV-2), emerged in China at the end of 2019 and has continued to spread throughout the world. The World Health Organization (WHO) reported that as of September 2022, about 600 million cases of COVID-19 and 6.4 million associated deaths have occurred. Vaccination against COVID-19 is currently the most-effective first line of defense against severe disease and death; however, the antigenicity of circulating SARS-CoV-2 variants affects the efficacy of the COVID-19 vaccines. Therapeutic monoclonal antibodies and antiviral drugs are available for the treatment of COVID-19 (1, 2); however, the risk of emerging escape or resistant viruses drives the need for alternative approaches.
Probiotics are defined as live microorganisms that provide health benefits to the host when administered in adequate amounts, (3); they include several genera of bacteria and yeast such as Lactobacillus, Bifidobacterium, Leuconostoc, Pediococcus, and Enterococcus (4, 5). Probiotics play an important role in balancing the intestinal microflora, which leads to modulation of the immune system. Previous studies have shown that probiotics have antiviral activity against respiratory viruses such as rhinovirus, influenza virus, respiratory syncytial virus, and SARS-CoV-2 (6–8). Although probiotics provide physiological benefits to the host, their safety profiles remain controversial, because they are live strains (9). Therefore, there is increasing interest in non-viable microorganisms or microbial cell extracts to avoid the risks of using live microorganisms. Lactiplantibacillus pentosus ONRICb0240 (b240) is an anaerobic, non-sporulating, Gram-positive bacterium originally isolated from fermented tea leaves. Clinical trials have demonstrated that heat-killed b240 enhances salivary IgA secretion, reduces the incidence of the common cold, and alleviates allergic symptoms (10–12). In addition, we and other groups have previously reported that oral intake of heat-killed b240 modulates mucosal immunity, which provides protection against influenza virus, Streptococcus pneumoniae, and Salmonella infection (13–16). Here, we evaluated the protective efficacy of heat-killed b240 against SARS-CoV-2 infection in mice.
Materials and methods
Cells
VeroE6/TMPRSS2 (JCRB 1819) cells (17) were propagated in the presence of 1 mg/ml geneticin (G418; In vivogen) and 5 μg/ml plasmocin prophylactic (In vivogen) in Dulbecco’s modified Eagle’s medium (DMEM) containing 10% Fetal Calf Serum (FCS). VeroE6/TMPRSS2 cells were maintained at 37 °C with 5% CO2 and regularly tested for mycoplasma contamination by using PCR, and confirmed to be mycoplasma-free.
Viruses
Mouse-adapted SARS-CoV-2 was generated by serial passages of SARS-CoV-2 (gamma: hCoV-19/Japan/TY7-501/2021) (18) in BALB/c mice. The detailed methods of mouse adaptation are currently unpublished (manuscript in preparation). Mouse-adapted SARS-CoV-2 was propagated in VeroE6/TMPRSS2 cells in VP-SFM (Thermo Fisher Scientific).
All experiments with SARS-CoV-2 were performed in enhanced biosafety level 3 (BSL3) containment laboratories at the University of Tokyo and the National Institute of Infectious Diseases, Japan, which are approved for such use by the Ministry of Agriculture, Forestry, and Fisheries, Japan.
Animal experiments and approvals
Animal studies were carried out in accordance with the recommendations in the Guide for the Care and Use of Laboratory Animals of the National Institutes of Health. The protocols were approved by the Animal Experiment Committee of the Institute of Medical Science, the University of Tokyo (approval number PA19-72). All animals were housed under specific pathogen-free conditions in a temperature control environment with a 12 h: 12h light: dark cycle, with 50% humidity and ad libitum access to water and standard laboratory chow. Virus inoculations were performed under anesthesia, and all efforts were made to minimize animal suffering.
Experimental infection of mice
Six-week-old female BALB/c mice (Japan SLC Inc., Shizuoka, Japan) were used in the study. Oral administration of heat-killed b240 was initiated in mice at six weeks of age. Mice were orally administered heat-killed b240 every day at a dose of 10 mg/mouse, which corresponds to 1010 cell counts of heat-killed microbe, in 200 μl of buffered saline for 5 weeks. The control group received saline. The b240 dose was determined on the basis of previous studies (14, 15).
On Day 21 of heat-killed b240 administration, mice were intranasally infected with PBS, or with 0.3 or 0.6 MLD50 of mouse-adapted SARS-CoV-2 [MLD50 = 103.3 plaque forming units (PFU).] under isoflurane anesthesia. To determine the effects of oral administration of heat-killed b240 on mouse mortality, mice were infected with 0.3 or 0.6 MLD50 of SARS-CoV-2 and their body weight and survival were monitored daily for 10 days post-infection (n = 20 for 0.3 MLD50; n = 10 for 0.6 MLD50). To investigate the effects of oral administration of heat-killed b240 on viral replication and host immune responses, the animals infected with 0.6 MLD50 of mouse-adapted SARS-CoV-2 were euthanized on Days 2 and 5 post-infection, and the virus titers in the nasal turbinates and lungs were determined by using plaque assays on VeroE6/TMPRSS2 cells.
Pathology
Excised animal tissues were fixed in 4% paraformaldehyde in PBS and processed for paraffin embedding. The paraffin blocks were cut into 3-µm-thick sections and mounted on silane-coated glass slides for histopathological examination. The sections were stained with hematoxylin and eosin.
Cell preparation and flow cytometry
To isolate single cells from lungs, lung tissue was minced, and fragments were digested in 5 ml of DMEM containing collagenase D (Roche, Basel, Switzerland)) for 30 min at 37°C. The single-cell suspension was filtered through a 70-µm cell strainer and washed twice with 5 ml of RPMI 1640. Leukocytes were enriched by centrifugation (14 min, 700 ×g) on a 33% Percoll gradient (Cytiva, Marlborough, MA, USA) in HBSS, and red blood cells (RBCs) were lysed by RBC lysis buffer (pluriSelect Life Science UG & Co.KG, Leipzig, Germany). Cells were then incubated with anti-CD16/32 Ab (93) to block Fc receptors and stained with antibodies specific to CD3 (17A2), CD45 (30-F11), CD4 (RM4-5), CD11b (M1/70), CD8a (53–6.7), CD11c (N418), I-A/I-E (M5/114.15.2), Ly6G (1A8), and Ly6C (HK1.4) from Biolegend or eBioscience (San Diego, CA, USA) and Live/Dead fixable aqua (Thermo Fisher Scientific, Waltham, MA, USA).
Data were acquired with CytoFLEX S (Beckman Coulter Inc., Brea, CA, USA) and data analysis was performed using FlowJo software (FlowJo, Ashland, OR, USA).
Cytokine and chemokine measurement
Under isoflurane anesthesia, twelve mice per group were infected with 0.6 MLD50 of mouse-adapted SARS-CoV-2 on Day 21 of heat-killed b240 administration. On Day 0 (pre) prior to the infection, and Days 2 and 5 post-infection, animals were euthanized and their lungs were collected. For cytokine and chemokine measurements, homogenates of mouse lungs were processed with the Bio-Plex Mouse Cytokine 23-Plex (Bio-Rad Laboratories).
Reagent availability
All materials are available from the authors or from commercially available sources.
Statistical analysis
GraphPad Prism software was used to analyze the data. Statistical analysis included unpaired Student’s t-tests, Mann-Whitney tests, the Log-rank (Mantel-Cox) test, and ANOVA with post-hoc tests. Differences among groups were considered significant for P values < 0.05.
Results
To evaluate the prophylactic effects of heat-killed b240 against SARS-CoV-2, we orally administered heat-killed b240 (10 mg/mouse) to Balb/c mice once daily for 21 days before intranasal infection with 0.3 or 0.6 MLD50 (50% mouse lethal dose) of mouse-adapted SARS-CoV-2. Thereafter, heat-killed b240 was administered once daily for 10 days. When mice were infected with 0.3 MLD50, the heat-killed b240 showed statistically significant improvement in body weight changes and survival (Figure 1A). In mice infected with 0.6 MLD50, although no significant differences in body weight or survival were observed between the animals that were treated with heat-killed b240 and those treated with saline (control), we observed smaller body weight reductions and better survival compared with 0.6 MLD50 infection of saline-treated mice (Figure 1B). Overall, these results suggest that heat-killed b240 may partially protect against SARS-CoV-2 infection. For detailed analyses, we chose the higher dose (i.e., 0.6 MLD50) to compare virus replication and host responses under more severe conditions.
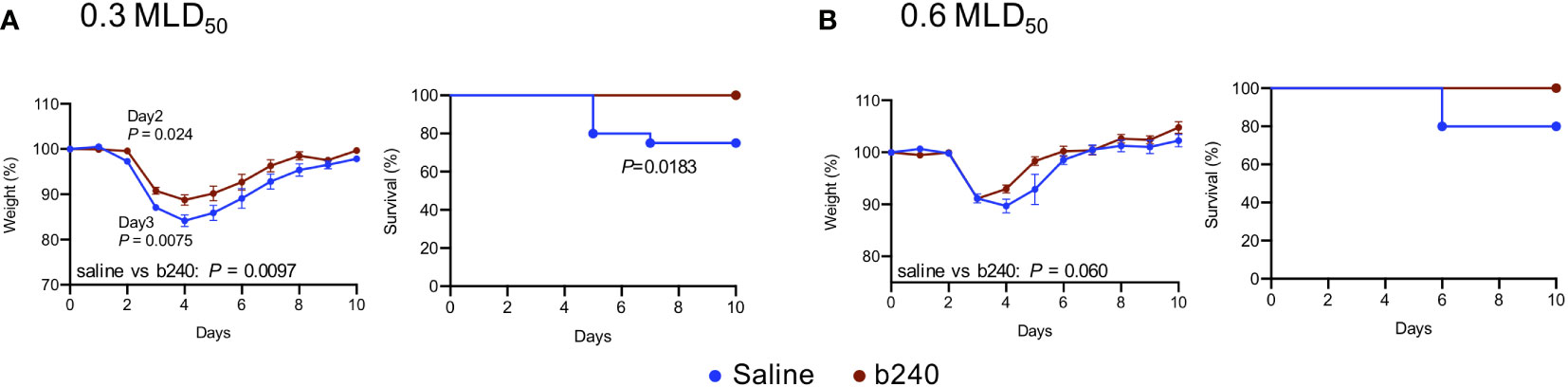
Figure 1 Efficacy of oral intake of heat-killed b240 in SARS-CoV-2-infected mice. Mice were administered heat-killed b240 at a dose of 10 mg/mouse daily for 21 days prior to infection and for 14 days after infection. Mice in the control group were administered saline. Mice were then intranasally infected with 0.3 (A) or 0.6 MLD50 (B) of mouse-adapted SARS-CoV-2 on Day 21 of heat-killed b240 administration. Body weight (left panels) and survival (right panels) were monitored daily for 10 days. The data are presented as the mean percentages of the starting weight ± s.e.m. Weight data were analyzed by using a two-way ANOVA followed by Dunnett’s test. Survival data were analyzed by using the Log-rank (Mantel-Cox) test. n = 20 for 0.3 MLD50, n = 10 for 0.6 MLD50.
We next assessed the effect of heat-killed b240 on virus replication in the respiratory tract of mice infected with the higher dose (0.6 MLD50) of mouse-adapted SARS-CoV-2. No obvious differences in virus titers in the nasal turbinates and lungs were found between the animals that received heat-killed b240 and those that received saline on Days 2 and 5 post-infection (Figure 2A). Furthermore, there were no differences in lung histology between heat-killed b240-treated mice and control mice on Day 5 post-infection (Figure 2B).
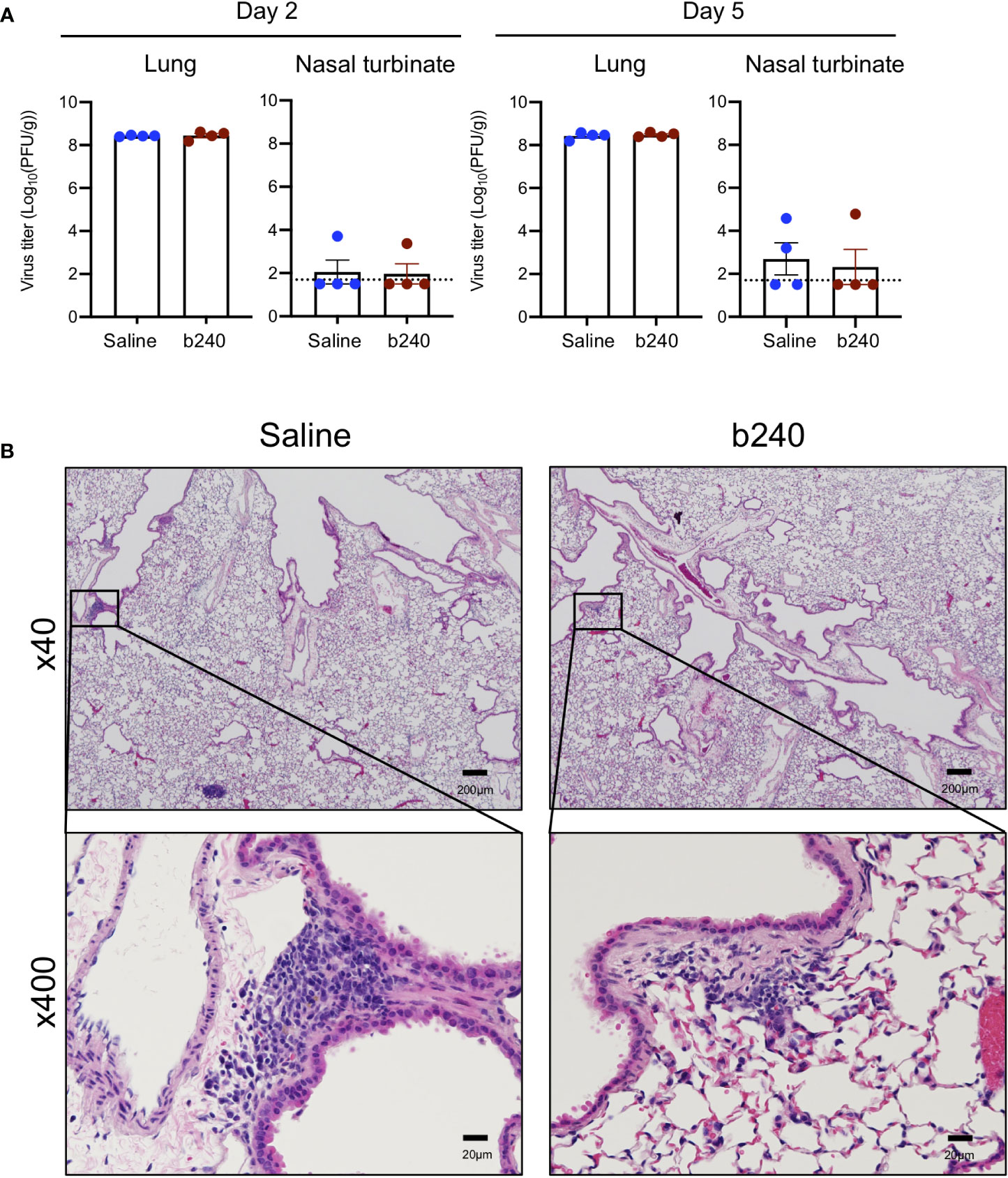
Figure 2 Virologic effect of oral intake of heat-killed b240 in SARS-CoV-2-infected mice. Mice were infected with 0.6 MLD50 of mouse-adapted SARS-CoV-2 on Day 21 of heat-killed b240 administration and euthanized on Days 2 and 5 post-infection. (A) Virus burdens in the lungs and nasal turbinates were determined by performing plaque assays. The values are means ± s.e.m. (n = 4). Points indicate data from individual mice. The lower limit of detection is indicated by the horizontal dashed line. Statistical significance was determined with a two-tailed Student’s t-test (lung) or the Mann-Whitney test (nasal turbinate). (B) Histopathologic examination of the lungs of infected mice (n = 3/group) on Day 5 post-infection. Representative images of infected mice are shown.
Probiotics have regulatory effects on host innate and adaptive immune responses (19, 20). Therefore, to assess whether heat-killed b240 can alter immune cell recruitment to the lungs following infection with SARS-CoV-2 in mice, heat-killed b240-treated mice were intranasally inoculated with 0.6 MLD50 of mouse-adapted SARS-CoV-2, and whole lungs were harvested pre-infection (Day 0) and on Days 2 and 5 post-infection. Flow cytometry analysis revealed no differences in CD4+T and CD8+T cell numbers in the lungs between heat-killed b240- and control vehicle-treated mice at any timepoints (Figures 3A, B). A rapid increase in neutrophil recruitment was observed in the lungs of infected mice treated with heat-killed b240 and the control mice on Day 2 post-infection, although no statistically significant difference in the percentage of neutrophils was observed between the two groups (Figures 3C, D). We also saw no difference in the percentage of Ly6chiCD11b+ or Ly6c+CD11b+ monocytes between the two groups (Figure 3D). Interestingly, however, the percentage of dendritic cells (DCs) was significantly higher for infected mice treated with heat-killed b240 compared with the infected control mice on Day 2 post-infection (Figure 3D).
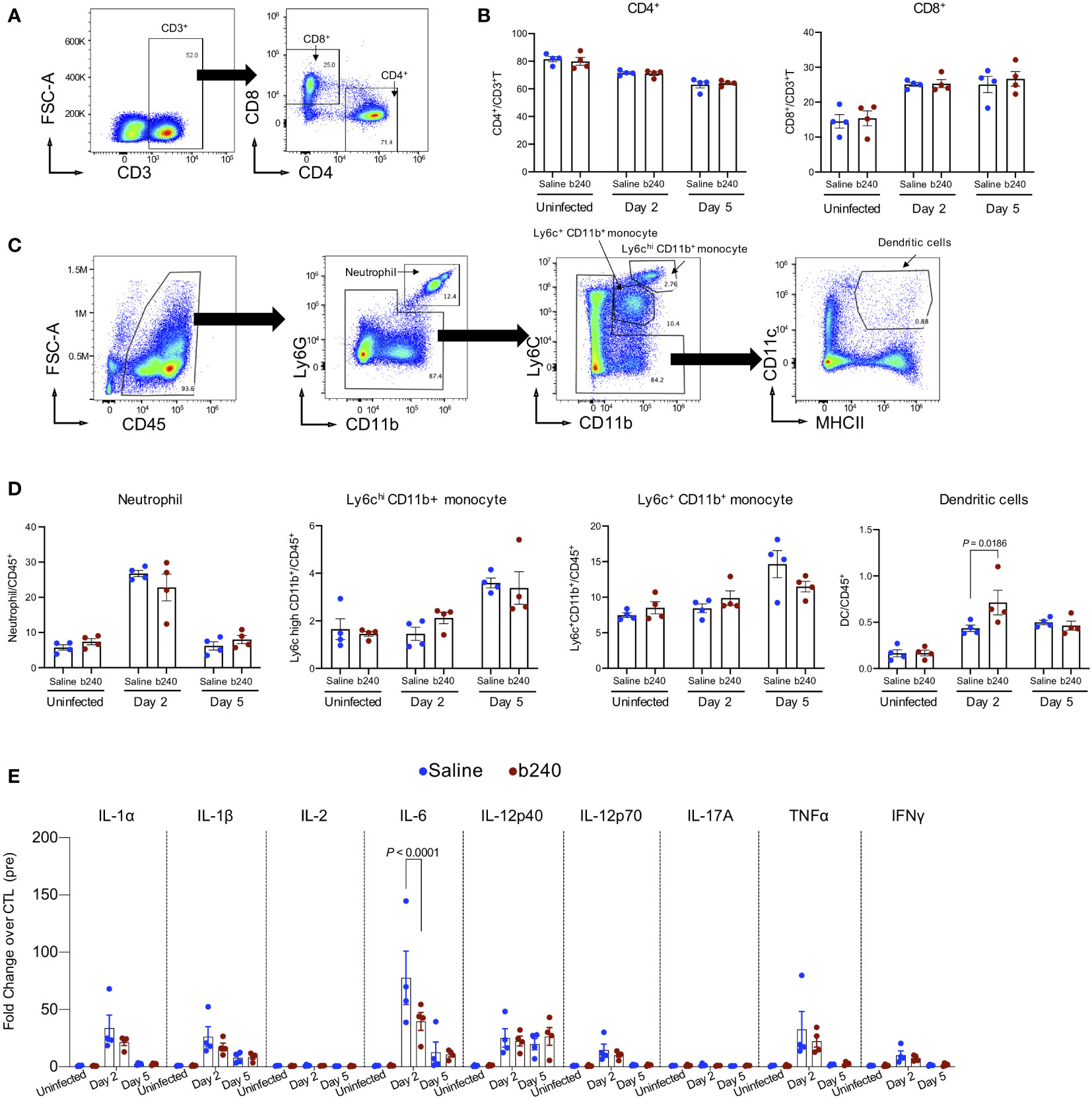
Figure 3 Immunologic effect of oral intake of heat-killed b240 in SARS-CoV-2-infected mice. Mice were infected with 0.6 MLD50 of mouse-adapted SARS-CoV-2 on Day 21 of heat-killed b240 administration. On Day 0 (pre) prior to the infection and Days 2 and 5 post-infection, mice were euthanized and their lungs were harvested. (A–D) Frequency of immune cells in lungs examined by use of flow cytometry. Representative gating strategies used to identify CD4+T and CD8+T cells (A), and neutrophil, monocytes, and dendritic cells (C) are shown. Cell frequency data are shown (n = 4/group) (B, D). The values are means ± s.e.m. (n = 4). Points indicate data from individual mice. Statistical significance was determined with a two-way ANOVA followed by Tukey’s multiple comparisons test. (E) The expression of proinflammatory cytokines in mouse lungs is shown. Vertical bars show the mean ± s.e.m (n = 4). Points indicate data from individual mice. Data were analyzed by using a two-way ANOVA with Tukey’s multiple comparisons test. All values were normalized to the mean value of the saline-treated mice on Day 0 (pre) prior to the infection.
Proinflammatory cytokines (21, 22), which are the central host mediators of innate immunity, are essential to recruit immune cells to sites of infection. These mediators are also associated with pulmonary inflammation and lung damage. Elevated levels of proinflammatory cytokines such as IL-6 and TNFα have been reported in patients with severe COVID-19 (21, 23, 24). We therefore examined the effects of oral administration of heat-killed b240 on the expression levels of pro-inflammatory cytokines (i.e., IL-1α, IL-1β, IL-2, IL-6, IL-12p40, IL-12p70, IL-17A, TNFα, and IFNγ) in mice infected with SARS-CoV-2 (Figure 3E). Consistent with our previous report (14), no significant differences in cytokine levels in the lungs were found between non-infected mice that were treated with heat-killed b240 and those given the control vehicle, indicating that oral administration of heat-killed b240 does not induce inflammatory responses (Figure 3E). The expression levels of proinflammatory cytokines in the lungs of infected mice treated with heat-killed b240 were similar to those in infected mice given the control vehicle, except for IL-6 on Day 2 post-infection; the IL-6 expression level was significantly lower for the former than for the latter (Figure 3E). These results suggest that heat-killed b240 may reduce the early host inflammatory responses including IL-6-mediated proinflammatory signaling caused by SARS-CoV-2 infection, leading to partial protection against SARS-CoV-2 infection.
Discussion
Our previous study showed that oral administration of heat-killed b240 enhanced protection against a lethal influenza A(H1N1) pdm virus in a mouse model (14). In the present study, we found that the oral intake of heat-killed b240 partially protects mice from SARS-CoV-2 infection. The heat-killed b240 treatment did not affect the virus titers in the respiratory organs of the mice infected with SARS-CoV-2; however, this treatment suppressed the expression of proinflammatory cytokines in the lungs. Previous studies have reported that heat-killed b240 inhibits the production of pro-inflammatory cytokines such as IL-6 and TNF-α after Streptococcus pneumoniae infection in mice (10) and that probiotics such as Bifidobacterium longum MM-2 and Lactobacillus plantarum 06CC2 inhibit the production of pro-inflammatory cytokines such as IL-6 and TNF-α after influenza virus infection in mice (25, 26). In addition, we previously showed that heat-killed b240 modulates the expression levels of genes involved in metabolism and antiviral responses in mice, which may result in the partial protection of pdmH1N1 influenza virus-infected mice by heat-killed b240 (14). Recent studies indicated that tightly regulated microbiota-host interplay influences the establishment of the immune system, which affect the outcome after pathogen-infection (27–30). Therefore, it is possible that heat-killed b240 could have altered the microbiota-host interaction, leading to the inhibition of IL-6 production in SARS-CoV-2-infected animals. Further investigation is needed to assess how heat-killed b240 treatment leads to the suppression of IL-6 production in virus-infected mice. Overall, these findings suggest that oral administration of heat-killed b240 may modulate the host immune responses in lungs infected with respiratory viruses such as influenza virus and SARS-CoV-2. Recently, several studies have reported improved outcomes in COVID-19 patients who received probiotics in clinical trials, suggesting promising beneficial effects of probiotics as part of COVID-19 management (31). It would be interesting to extend our study and examine the effect of b240 in COVID-19 patients.
We found that DCs were slightly but significantly increased in heat-killed b240-treated mice on Day 2 post-infection. Previous studies have demonstrated that DCs induce cytotoxic T lymphocytes (CTL)-mediated antiviral immunity (32, 33), which suggest that increased levels of DCs may contribute to protection upon SARS-CoV-2 infection. How DCs are recruited or induced in the lungs of SARS-CoV-2-infected mice upon oral administration of heat-killed b240 should be examined in a future study.
In conclusion, our data suggest that oral intake of heat-killed Lactiplantibacillus pentosus ONRICb0240 promotes the survival of SARS-CoV-2-infected mice.
Data availability statement
The raw data supporting the conclusions of this article will be made available by the authors, without undue reservation.
Ethics statement
The animal study was reviewed and approved by Animal Experiment Committee of the Institute of Medical Science, the University of Tokyo.
Author contributions
MK, RU, YKo, MIm, NK, and Y.Ka conceived and designed the research. Y.Ko and NK contributed reagents. MK, RU, MIt, and SY performed the experiments and analyzed the data. RU, MIm, and YKa wrote the initial draft, with all other authors providing editorial comments. All authors contributed to the article and approved the submitted version.
Funding
This work was supported by the Japan Program for Infectious Diseases Research and Infrastructure (JP22wm0125002) and the Japan Initiative for World-leading Vaccine Research and Development Centers (JP223fa627001) from the Japan Agency for Medical Research and Development.
Acknowledgments
We thank Susan Watson for scientific editing. We also thank Rie Onoue, Madoka Yoshikawa, Naoko Mizutani, and Kengo Kajiyama for technical assistance, and Sept.Sapie Co., Ltd. for pathological analyses of the lungs of the mice.
Conflict of interest
YKa is a co-founder of FluGen and has received related funding support from Otsuka Pharmaceutical Co., Ltd. as well as unrelated funding support from Daiichi Sankyo Pharmaceutical, Toya-ma Chemical, Tauns Laboratories, Inc., Shionogi & Co. LTD, KM Biolog-ics, Kyoritsu Seiyaku, Shinya Corporation, and Fuji Rebio. YKo and NK are employees of Otsuka Pharmaceutical Co., Ltd.
Publisher’s note
All claims expressed in this article are solely those of the authors and do not necessarily represent those of their affiliated organizations, or those of the publisher, the editors and the reviewers. Any product that may be evaluated in this article, or claim that may be made by its manufacturer, is not guaranteed or endorsed by the publisher.
References
1. Ho C, Lee PC. COVID-19 treatment-current status, advances, and gap. Pathogens (2022) 11(10):1201. doi: 10.3390/pathogens11101201
2. Robinson PC, Liew DFL, Tanner HL, Grainger JR, Dwek RA, Reisler RB, et al. COVID-19 therapeutics: Challenges and directions for the future. Proc Natl Acad Sci USA (2022) 119(15):e2119893119. doi: 10.1073/pnas.2119893119
3. FAO/WHO. Probiotics in Food: Health and Nutritional Properties and Guidelines for Evaluation. Report of a Joint FAO/WHO Expert Consultation on Evaluation of Health and Nutritional Properties of Probiotics in Food Including Powder Milk with Live Lactic Acid Bacteria. Rome (2006).
4. Sundararaman A, Ray M, Ravindra PV, Halami PM. Role of probiotics to combat viral infections with emphasis on COVID-19. Appl Microbiol Biotechnol (2020) 104(19):8089–104. doi: 10.1007/s00253-020-10832-4
5. El Hage R, Hernandez-Sanabria E, Van de Wiele T. Emerging trends in “Smart probiotics”: Functional consideration for the development of novel health and industrial applications. Front Microbiol (2017) 8:1889. doi: 10.3389/fmicb.2017.01889
6. Darbandi A, Asadi A, Ghanavati R, Afifirad R, Darb Emamie A, Kakanj M, et al. The effect of probiotics on respiratory tract infection with special emphasis on COVID-19: Systemic review 2010-20. Int J Infect Dis (2021) 105:91–104. doi: 10.1016/j.ijid.2021.02.011
7. Luoto R, Ruuskanen O, Waris M, Kalliomaki M, Salminen S, Isolauri E. Prebiotic and probiotic supplementation prevents rhinovirus infections in preterm infants: a randomized, placebo-controlled trial. J Allergy Clin Immunol (2014) 133(2):405–13. doi: 10.1016/j.jaci.2013.08.020
8. Wischmeyer PE, Tang H, Ren Y, Bohannon L, Ramirez ZE, Andermann TM, et al. Daily lactobacillus probiotic versus placebo in COVID-19-Exposed household contacts (PROTECT-EHC): A randomized clinical trial. medRxiv (2022). doi: 10.1101/2022.01.04.21268275
9. Pique N, Berlanga M, Minana-Galbis D. Health benefits of heat-killed (Tyndallized) probiotics: An overview. Int J Mol Sci (2019) 20(10):2534. doi: 10.3390/ijms20102534
10. Kotani Y, Shinkai S, Okamatsu H, Toba M, Ogawa K, Yoshida H, et al. Oral intake of lactobacillus pentosus strain b240 accelerates salivary immunoglobulin a secretion in the elderly: A randomized, placebo-controlled, double-blind trial. Immun Ageing (2010) 7:11. doi: 10.1186/1742-4933-7-11
11. Shinkai S, Toba M, Saito T, Sato I, Tsubouchi M, Taira K, et al. Immunoprotective effects of oral intake of heat-killed lactobacillus pentosus strain b240 in elderly adults: a randomised, double-blind, placebo-controlled trial. Br J Nutr (2013) 109(10):1856–65. doi: 10.1017/S0007114512003753
12. Saito T, Hamuro K, Saito H, Sakoda T, Natsume C, Hamamoto K, et al. Heat–killed lactobacillus pentosus B240 improves overall face scale score FollowingJapanese cedar pollen exposure in an environmental exposure Unit–A randomized, double–blind, placebo–controlled clinical trial. Jpn Pharmacol Ther (2019) 47(11):1901–12.
13. Tanaka A, Seki M, Yamahira S, Noguchi H, Kosai K, Toba M, et al. Lactobacillus pentosus strain b240 suppresses pneumonia induced by streptococcus pneumoniae in mice. Lett Appl Microbiol (2011) 53(1):35–43. doi: 10.1111/j.1472-765X.2011.03079.x
14. Kiso M, Takano R, Sakabe S, Katsura H, Shinya K, Uraki R, et al. Protective efficacy of orally administered, heat-killed lactobacillus pentosus b240 against influenza a virus. Sci Rep (2013) 3:1563. doi: 10.1038/srep01563
15. Kobayashi N, Saito T, Uematsu T, Kishi K, Toba M, Kohda N, et al. Oral administration of heat-killed lactobacillus pentosus strain b240 augments protection against influenza virus infection in mice. Int Immunopharmacol (2011) 11(2):199–203. doi: 10.1016/j.intimp.2010.11.019
16. Ishikawa H, Kutsukake E, Fukui T, Sato I, Shirai T, Kurihara T, et al. Oral administration of heat-killed lactobacillus plantarum strain b240 protected mice against salmonella enterica serovar typhimurium. Biosci Biotechnol Biochem (2010) 74(7):1338–42. doi: 10.1271/bbb.90871
17. Matsuyama S, Nao N, Shirato K, Kawase M, Saito S, Takayama I, et al. Enhanced isolation of SARS-CoV-2 by TMPRSS2-expressing cells. Proc Natl Acad Sci U S A (2020) 117(13):7001–3. doi: 10.1073/pnas.2002589117
18. Imai M, Halfmann PJ, Yamayoshi S, Iwatsuki-Horimoto K, Chiba S, Watanabe T, et al. Characterization of a new SARS-CoV-2 variant that emerged in Brazil. Proc Natl Acad Sci U.S.A. (2021) 118(27):e2106535118. doi: 10.1073/pnas.2106535118
19. Yan F, Polk DB. Probiotics and immune health. Curr Opin Gastroenterol (2011) 27(6):496–501. doi: 10.1097/MOG.0b013e32834baa4d
20. Raheem A, Liang L, Zhang G, Cui S. Modulatory effects of probiotics during pathogenic infections with emphasis on immune regulation. Front Immunol (2021) 12:616713. doi: 10.3389/fimmu.2021.616713
21. Costela-Ruiz VJ, Illescas-Montes R, Puerta-Puerta JM, Ruiz C, Melguizo-Rodriguez L. SARS-CoV-2 infection: The role of cytokines in COVID-19 disease. Cytokine Growth Factor Rev (2020) 54:62–75. doi: 10.1016/j.cytogfr.2020.06.001
22. Dinarello CA. Proinflammatory cytokines. Chest (2000) 118(2):503–8. doi: 10.1378/chest.118.2.503
23. Chu H, Chan JF, Wang Y, Yuen TT, Chai Y, Hou Y, et al. Comparative replication and immune activation profiles of SARS-CoV-2 and SARS-CoV in human lungs: An ex vivo study with implications for the pathogenesis of COVID-19. Clin Infect Dis (2020) 71(6):1400–9. doi: 10.1093/cid/ciaa410
24. Karki R, Sharma BR, Tuladhar S, Williams EP, Zalduondo L, Samir P, et al. Synergism of TNF-alpha and IFN-gamma triggers inflammatory cell death, tissue damage, and mortality in SARS-CoV-2 infection and cytokine shock syndromes. Cell (2021) 184(1):149–68 e17. doi: 10.1016/j.cell.2020.11.025
25. Kawahara T, Takahashi T, Oishi K, Tanaka H, Masuda M, Takahashi S, et al. Consecutive oral administration of bifidobacterium longum MM-2 improves the defense system against influenza virus infection by enhancing natural killer cell activity in a murine model. Microbiol Immunol (2015) 59(1):1–12. doi: 10.1111/1348-0421.12210
26. Takeda S, Takeshita M, Kikuchi Y, Dashnyam B, Kawahara S, Yoshida H, et al. Efficacy of oral administration of heat-killed probiotics from Mongolian dairy products against influenza infection in mice: Alleviation of influenza infection by its immunomodulatory activity through intestinal immunity. Int Immunopharmacol (2011) 11(12):1976–83. doi: 10.1016/j.intimp.2011.08.007
27. Blander JM, Longman RS, Iliev ID, Sonnenberg GF, Artis D. Regulation of inflammation by microbiota interactions with the host. Nat Immunol (2017) 18(8):851–60. doi: 10.1038/ni.3780
28. Maslowski KM, Mackay CR. Diet, gut microbiota and immune responses. Nat Immunol (2011) 12(1):5–9. doi: 10.1038/ni0111-5
29. Thaiss CA, Zmora N, Levy M, Elinav E. The microbiome and innate immunity. Nature (2016) 535(7610):65–74. doi: 10.1038/nature18847
30. Schmidt TSB, Raes J, Bork P. The human gut microbiome: From association to modulation. Cell (2018) 172(6):1198–215. doi: 10.1016/j.cell.2018.02.044
31. Brahma S, Naik A, Lordan R. Probiotics: A gut response to the COVID-19 pandemic but what does the evidence show? Clin Nutr ESPEN (2022) 51:17–27. doi: 10.1016/j.clnesp.2022.08.023
32. Ludewig B, Ehl S, Karrer U, Odermatt B, Hengartner H, Zinkernagel RM. Dendritic cells efficiently induce protective antiviral immunity. J Virol (1998) 72(5):3812–8. doi: 10.1128/JVI.72.5.3812-3818.1998
Keywords: SARS-CoV-2, probiotics, Lactiplantibacillus pentosus ONRICb0240, mouse model, host responses
Citation: Kiso M, Uraki R, Ito M, Yamayoshi S, Kotani Y, Imai M, Kohda N and Kawaoka Y (2023) Oral intake of heat-killed Lactiplantibacillus pentosus ONRICb0240 partially protects mice against SARS-CoV-2 infection. Front. Virol. 3:1137133. doi: 10.3389/fviro.2023.1137133
Received: 04 January 2023; Accepted: 20 February 2023;
Published: 06 March 2023.
Edited by:
Keita Matsuno, Hokkaido University, JapanReviewed by:
Kei Miyakawa, National Institute of Infectious Diseases (NIID), JapanElahe Abdolalipour, Pasteur Institute of Iran, Iran
Copyright © 2023 Kiso, Uraki, Ito, Yamayoshi, Kotani, Imai, Kohda and Kawaoka. This is an open-access article distributed under the terms of the Creative Commons Attribution License (CC BY). The use, distribution or reproduction in other forums is permitted, provided the original author(s) and the copyright owner(s) are credited and that the original publication in this journal is cited, in accordance with accepted academic practice. No use, distribution or reproduction is permitted which does not comply with these terms.
*Correspondence: Yoshihiro Kawaoka, eW9zaGloaXJvLmthd2Fva2FAd2lzYy5lZHU=