- 1Archaeal Virology Unit, Institut Pasteur, Paris, France
- 2Institut de Biologie de l’École Normale Supérieure, CNRS, INSERM, Paris, France
Viruses are highly evolvable biological entities capable of wreaking havoc on our society. Therefore, a better understanding of virus evolution is important for two main reasons: (i) it will lead to better management of current diseases and prevention of future ones, and (ii) it will contribute to a better understanding of evolutionary processes and their dynamics. In order to understand the evolution of viruses as a whole, it is necessary to consider different elements that shape virus evolution. In this review, we give a general overview of the most relevant factors that determine the evolution of plant viruses. We will focus on mutation rates, epistasis, robustness, recombination, genome organization, virus-host interactions, transmission, community interactions and abiotic factors. Since this review gives a summarized overview of the most important factors in virus evolution it can be a useful starting material for anyone interested in approaching (plant) virus evolution.
Introduction
Viruses can rapidly tune the efficiencies of their replication, intra-host movement and between-host transmission to maximize their fitness (1). The consequent virus adaptation and propagation through host populations impacts the infected hosts’ health as well as the ecosystem (2). The study of virus evolution provides useful knowledge about specific evolutionary parameters that can be targeted to disrupt virus populations and prevent or mitigate viral diseases (3). Furthermore, studying viruses allows us to address evolutionary questions in a relatively short period of time (4).
The dimensions of plant virus evolution
The evolution of viruses is shaped by numerous factors (5, 6). In this minireview we will use plant viruses as an exemplar to outline the most important factors described so far. In order to better address this, we have classified each component affecting virus evolution into different levels (Figure 1). In the following sections, we will summarize each one.
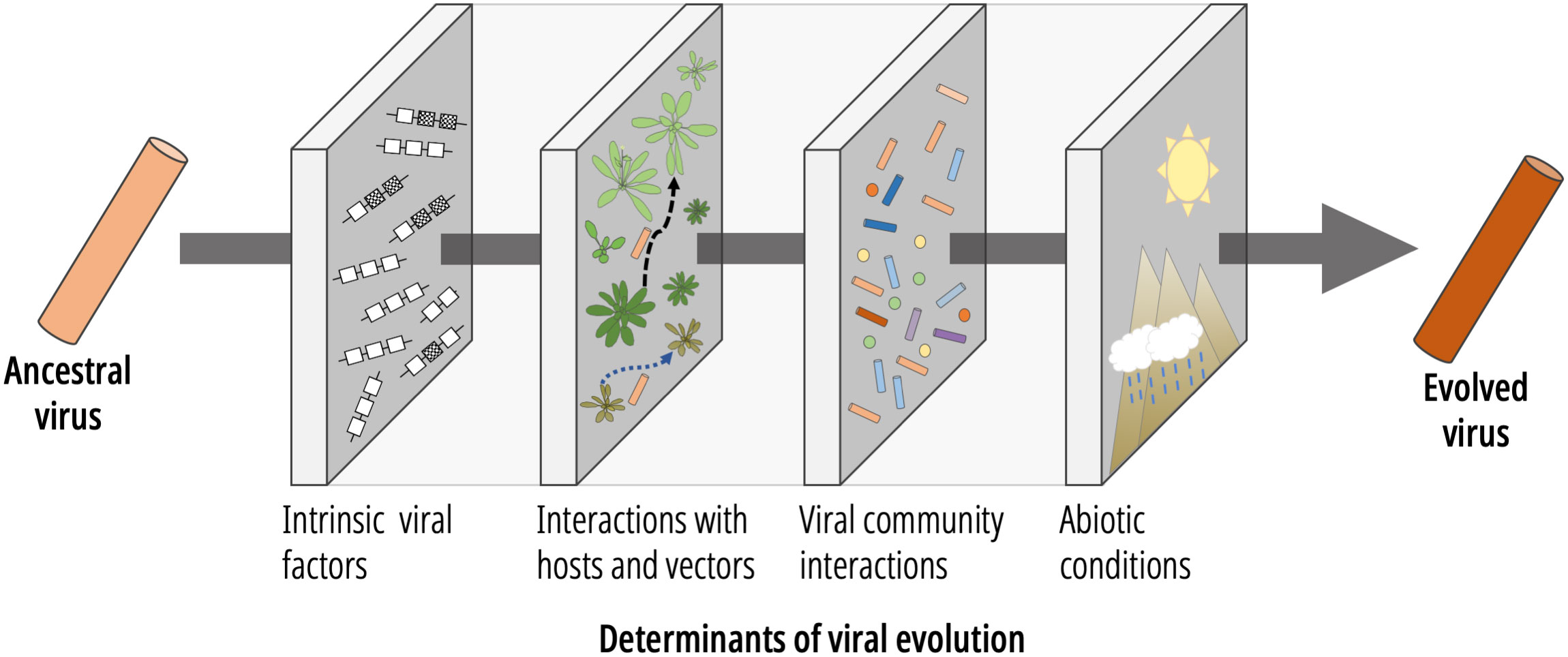
Figure 1 Representation of some of the most relevant factors affecting plant virus evolution, from an ancestral to the evolved virus.
Intrinsic viral factors
Plant viruses can have DNA or RNA genomes of different sizes, architectures, and with different mutation rates.
Sources of genetic variation
The main drivers of genetic variation in viruses are mutation, recombination and reassortment. Generally, single stranded RNA and reverse transcribing (RT) viruses have higher mutation rates (10-6 – 10-4 substitutions per nucleotide per cell infection) compared to double or single stranded DNA viruses (10-8 – 10-6) (7–11). The mutation rate is higher in RNA and RT viruses due to the high-error prone RNA-dependent RNA polymerase and RNA-dependent DNA polymerase (retrotranscriptases, RT) that lack the proof-reading ability or base excision repair (12). Viral mutation rates can be measured as per strand copying or per cell infection estimated rate. These two definitions stem from two different modes of replication: (i) linear replication (mutation rates defined as per strand copying), where multiple copies of the genome are made from the same template or (ii) geometric replication (mutation rates defined as per cell infection), where progeny strands can also be used as templates leading to a geometrical increase in the number of molecules and mutations (8). The actual replication mode of viruses can alternate between the two mechanisms (13). Some viruses can have lower mutation rates and generate less diversity due to their persistent or acute lifestyle (14). Other factors affecting mutation rates in plant viruses are mutagens, host antiviral enzymes and spontaneous chemical reactions (15). RNA viruses tend to have smaller genomes since the probability to accumulate detrimental mutations is smaller (16–19). Although there are exceptions to this rule, for example, closteroviruses that infect plants with genome sizes of up to 20kb (the usual size is 4-12 kb) (20). These large closterovirus genomes can be explained by the acquisition of new genes, such as heat shock protein 70, and the presence of duplicated genes (21). It appears 20kb is the size limit for an RNA virus without a proofreading activity (20). Compared to animal viruses, plant viruses have lower mutation rates and are genetically more stable since they are under weaker immune selection, strong stabilizing selection, strong bottlenecks or different replication modes (21–33). Mutations generate diversity and novelty upon which natural selection can act, thus giving rise to new variants in the virus population (15). A higher mutation rate does not confer greater adaptability, instead high mutation rates of viruses (especially RNA) are in line with the hypothesis in which faster replication is favored at the cost of fidelity (11, 34, 35). This type of replication generates a large proportion of detrimental mutations, and viruses live in an error-threshold or mutation-selection balance. Still, with so many new variants generated, the chances that a beneficial mutation will appear in the population will also increase. This is important in adaptation to new environments and evasion of host defenses (36–39).
A way that viruses can mitigate the detrimental effects of large mutation rates and possibly adapt better to new environments is through epistasis, the interaction between mutations resulting in a lower or higher fitness of the virus. Epistasis seems to be the main driving force behind across-host fitness trade-offs and adaptive processes since it determines the effect of mutations (40). Most studies on epistasis in plant viruses focused on RNA viruses and showed a prevalence of antagonistic epistasis, where two mutations combined have higher fitness than each of the single mutations. This antagonistic epistasis is highly dependent on the host background (41–45). In RNA viruses the effect of epistasis is large due to their small genome size, presence of secondary structures, overlapping genes and multifunctional proteins where disruption of one function affects many others (38). Another important phenomenon that affects the fitness of viruses in their hosts is antagonistic pleiotropy, where mutations that are beneficial in one host can be detrimental in another (46–48). Because of that, antagonistic pleiotropy can lead to viruses capable of crossing host or species barriers that provoke novel diseases.
Viruses can also buffer the effects of large mutation rates through robustness, or the constancy of the phenotype in the face of heritable perturbations (genetic or epigenetic) or non-heritable perturbations such as external stressors (for example heat, light changes or developmental noise) (49). Robustness acts on the population level and helps viruses deal with genome instability by increasing their tolerance to mutations (50, 51). Due to their nature (high mutational pressures, small genomes that maximize the impact of mutations, and constantly changing environmental conditions) and the fact that they constantly face changing and unpredictable environments (host switches, environmental perturbations, and changes in the physiological state and the immune responses of the host), robustness plays an important role in RNA viruses (52–57). Genetic robustness and environmental robustness have been described in an RNA plant virus (51), confirming the theoretical postulates (50). Generally, plant viruses that are more robust will have more chances of survival and further propagation in unpredictable environments.
Recombination is an event in which two RNA or DNA viruses coinfecting a single host cell exchange parts of their genome (15, 58–63). Recombination is frequent in (+) RNA, ssDNA and reverse transcribing viruses and rare in (-) RNA viruses (37, 62, 64–69). While reassortment is exclusive to segmented RNA and ssDNA viruses, where different viruses exchange genome segments and pack heterologous molecules into a single virion (70–74). These differences in recombination rates can be explained by the evident differences in the biology of (-) RNA viruses compared to (+) RNA, ssDNA and reverse transcribing viruses. In (-) RNA viruses the genomes are quickly bound to the nucleocapsid subunit which limits the recombination events (70). Many theories have emerged about the evolutionary advantages of recombination, explaining it as: (i) a sort of sexual reproduction, (ii) a derivate of the processivity of the RNA polymerase, or (iii) a ‘recombination dependent replication’ mechanism in geminiviruses, where fragments of the genome are recovered to create recombinant viruses (70, 75, 76). While the most plausible theory regarding the existence of reassortment in segmented RNA viruses and ssDNA (begomoviruses and nanoviruses) describes this process as a by-product of the segmented genome structure (77). Various studies have shown that recombination plays an important role in RNA and ssDNA virus evolution and epidemiology by affecting virulence, pathogenesis, host range, vector transmission, and evasion of host immunity (62, 78). Changes in virus genomes caused by recombination or reassortment can lead to the emergence of new virus diseases and cause significant changes in symptomatology (79–81).
Genome architecture
A considerable proportion of known plant viral species are multipartite and their genome architecture is composed of several segments which are packaged in different viral particles that are independently transmitted (82). A multipartite genome allows viruses to rapidly tune their gene expression by adjusting their relative copy number (83). The adjustments in the proportion of virus segments can regulate the expression of viral genes, thus possibly having a consequential impact on viral fitness and infectivity. This rapid adjustment is particularly useful under environmental changes, as it allows the multipartite virus to have an immediate adaptive response (84). The genome formula of a virus is adaptive and it changes in different hosts (85). Furthermore, even if infection starts with different relative abundances of each segment, their final abundance will evolve to a host-specific segment ratio equilibrium (86). This frequency-dependent selection may happen at the replicative level or during encapsidation. However, it has been shown that not all segments of a multipartite virus need to infect and replicate within the same host cell since viral proteins can be exchanged between host cells, ensuring the availability of all viral proteins needed to assemble new virus particles (87). This phenomenon implies that each fragment may be exposed to slightly different conditions depending on the status of the particular cell they are infecting.
Virus interactions with hosts and vectors
Interactions with hosts
In order to successfully infect a cell of a new host, viruses need to first overcome the host defense response. Plants have evolved mechanisms to restrict virus replication and movement in order to resist infection or tolerate it (88–94), while viruses evolved mechanisms to fight the host defense response (95, 96). This ongoing arms race between plant defense mechanisms and viruses drives co-evolutionary events in both of them. So far, multiple studies described how individuals with different degrees of susceptibility and tolerance to virus infection impact the evolution of the virus, where more permissive hosts select for less virulent and specialized viruses, while more restrictive hosts select for more virulent and generalist pathogens (97). Laboratory experiments show that deficiencies in different host immunity mechanisms determine the rate of evolution of a plant RNA virus, its genetic adaptations, and the degree of specialization of the evolved virus (98). Virus adaptation to certain host defenses can be highly complex, since virus evolution is influenced by the host’s genetic background (48). At the host population level, the defenses vary between individuals. This heterogeneity of plant defense responses (in tolerance or susceptibility to a virus) plays an important role in shaping the patterns of virus evolution (99, 100). For some viruses, the age of the host may influence and lead to an increased virulence of a virus (101). The age of the host can result in viruses with altered infectivity rates, that evolve at different rates and acquire different mutations (102). Notably, even when a virus adapts to a specific new host, the newly acquired adaptive mutations may be hampering the virus’ fitness on their original host (103–106).
The evolution of a virus in a host is in part also shaped by the host factors that are necessary for virus replication and transmission. Virus not only needs to adapt to the host to overcome its defenses but also to efficiently hijack the cell, manipulating it for its benefit (107, 108). The host factors required by a virus for successful infection are specific to each host and virus and vary depending on the step of the infection (109).
Transmission
In order to spread to a new host and ensure its survival in a host population, a plant virus needs to be transmitted from the infected plant to a naïve one. This transmission can occur in two different manners: (i) vertically – a way of transmission where plant viruses can reach the often virus-free reproductive tissues of the same plant, accumulate in the seeds and infect the offspring (110, 111), and (ii) horizontally – where a virus is transmitted from an infected plant to a healthy one by physical contact. This mechanical transmission can be caused by the use of agricultural machinery, grazing animals, plants touching due to winds, exposure to infected plant-derived products, etc. (112–114). A key element in virus horizontal transmissions is vectors. Vectors are living organisms that can acquire and disseminate plant viruses by causing mechanical damage to the plant host, disrupting its mechanical barriers and allowing the virus to passively penetrate the plant cuticle and cell wall. In order to be horizontally transmitted by a vector, a virus needs to be efficiently acquired and transmitted by its vector. If a virus is acquired but inefficiently transmitted, the spread of the virus through a host population will be hampered (115). In addition, viruses may evolve different ways to manipulate their plant hosts to generate compounds or morphological phenotypes that attract vectors (116, 117), which will increase the chances of the virus being transmitted to a new host. The nature of the manipulation of the vector’s choice and the success of the viral spread is complex (118, 119) and has deep evolutionary consequences (120), especially since the feeding preference of the vector affects the types of hosts a virus encounters, and therefore shapes the evolution of a virus toward a specialist or a generalist pathogen (121).
The transmission mode can also shape virus evolution. Experimental evolution has shown that adaptation to vertical transmission can result in less virulent viruses with a lower viral load (110, 122). Apart from epidemiological traits, the mode of transmission can also affect the nucleotide diversity of virus populations, where an RNA virus had higher nucleotide diversity when vertically transmitted (via tubers) in comparation with horizontal transmission (via vector or mechanic) (123). The horizontal transmission inflicts a stronger pressure on viruses as this mode of transmission constrains virus population in each of the different steps of the transmission (124) and the interactions with vectors seem to impose a stronger selective pressure on certain viral proteins than the interactions with hosts or other viruses (125). During the horizontal transmission of the virus and its posterior systemic movement through the new host, the virus population goes through bottleneck events. In these bottleneck events only a reduced fraction of the virus population finds a new host, thus limiting the genetic variation of the population and potentially leading to genetic drift (25, 27, 29). Narrow bottlenecks that impose a strong burden on the genetic variation of a virus population are frequent, yet still some viruses may be under wider bottlenecks (126). Soft bottlenecks may even be beneficial for the virus population as they allow the exploration of rugged genotypic spaces with multiple fitness peaks (127).
Viral community interactions
When a virus infects a new host, it might have to interact with other pathogens that are infecting the same host. In this section we will focus on viral interactions with other viruses and the evolutionary consequences of these interactions (128), still, there are complex interactions with other non-viral pathogens that will not be discussed here (129).
Hosts can be co-infected with more than one virus at the same time (130). It has been shown that a considerable proportion of both symptomatic and asymptomatic wild plants are coinfected by multiple virus species or strains (131). In mixed infections viruses interact with each other which can result in neutral interactions, a range of competitive interactions that are detrimental for at least one of the competitors (132), or beneficial interactions. There is an increased interest (and therefore more reported cases) in beneficial interactions, where at least one virus aids another virus’ replication and propagation (133).
The structure of a viral community can be host-dependent (134). Therefore, a virus can reach different viral loads and be transmitted with different efficiencies depending on the host that is being infected with multiple viruses (135). This host effect on co-infection and the way viruses interact with their hosts and their vectors will impact the virus transmission (136, 137). Furthermore, it will influence the fitness of the virus and affect the evolution of all viral populations interacting in the co-infected host. The competition between two viruses can occur within the host but also within the vector. Examples have been found where a virus that was a weaker competitor in the host became the dominant competitor in the vector (138). This suggests that evolutive pressures a virus has to face during competition are context-dependent. Within the conditions that influence viral communities, abiotic environmental factors can also play a key role by modifying the diversity of a virus population and the already complex virus-virus interactions (139, 140).
Abiotic environmental conditions
The environment plays a key role in virus evolution and it affects all other factors mentioned previously. As described in section 1, high mutation rate, recombination, robustness and segmented genomes would be favored in variable environments, since they promote a rapid response of the virus to changing conditions. At the host level, abiotic conditions modify the physiological, hormonal and transcriptional state of the plant (141–144). This change in the plant status affects the plant - virus interaction (145), especially considering that abiotic stressors and viral infection activate the same signaling pathways in the plant and often interfere with one another (146). Therefore, many abiotic conditions (such as drought, high temperatures, elevated salinity, CO2 levels, etc.) have been described as having an effect on the course of plant virus infection. These environmental stressors change the plant - virus interaction by inhibiting plant host defense responses and promoting plant susceptibility to viruses, which in turn aids viral infection and symptom development although reverse effects have also been observed (147–160). Effects of certain abiotic stressors on plant virus infection are becoming increasingly important in the wake of climate change, in particular, temperature increase (161). For example, seasonality (that is directly correlated with temperature) negatively affected an RNA virus accumulation during winter (162). Environmental conditions can also modify virus transmission. Higher light intensity, temperature, CO2 and water levels, changed the level of aphid propagation and seed transmission of plant viruses by affecting plant resistance or aphid behavior (155, 163–173).
In summary, the optimal fitness of a virus is highly dependent on the environment (174). However, environmental impact on virus epidemiology may also vary depending on the host’s genetics (175). Notably, under certain stressful environments virus-infected plant hosts can even have higher fitness and survival compared to non-infected plants (176–179). This environmental-induced change in the host-virus interaction can have consequences on the nature of their relationship; for example, switching it from a parasitic relationship to a mutualistic one (180).
Conclusions
The study of the factors that shape the evolution of viruses is extremely important from both the evolutionary and epidemic point of view. In order to unveil these factors and their impacts, plant viruses have proven to be a great practical complex adaptive system used in experimental studies because of (i) their rapid evolutionary rate, (ii) lesser ethical concerns regarding plant hosts, (iii) the lower costs and requirements of plant maintenance compared to animal maintenance, making it feasible to perform experiments on a large number of hosts, and (iv) the safer side of plant virus research compared to animal research. However, there are many aspects characteristic to plant-virus pathosystems one has to keep in mind when doing plant virus evolutionary experiments: the existence of multipartite genomes, reassortment, specific modes of transport through the host, vector transmission, and a host that lacks an adaptive immune system. All in all, the particularities of plant viruses are highly interesting from the evolutionary point of view. The interesting traits of plant viruses as a system to study evolution should further heighten the interest of scientists (and funders).
Author contributions
AB and RG collected and reviewed the published literature, wrote, and revised the manuscript. All authors contributed to the article and approved the submitted version.
Funding
AB research is currently funded by an FRM Fellowship (SPF202110014092) and RG by an EMBO Postdoctoral Fellowship (ALTF 311-2021).
Acknowledgments
We apologize to colleagues whose work was not cited in this minireview due to the word limit. We would like to thank Reviewers for their valuable input, which improved the quality of the manuscript. We also thank Christina Toft for critically reading the manuscript. AB research is currently funded by an FRM Fellowship (SPF202110014092) and RG by an EMBO Postdoctoral Fellowship (ALTF 311-2021).
Conflict of interest
The authors declare that the research was conducted in the absence of any commercial or financial relationships that could be construed as a potential conflict of interest.
Publisher’s note
All claims expressed in this article are solely those of the authors and do not necessarily represent those of their affiliated organizations, or those of the publisher, the editors and the reviewers. Any product that may be evaluated in this article, or claim that may be made by its manufacturer, is not guaranteed or endorsed by the publisher.
References
1. Lefeuvre P, Martin DP, Elena SF, Shepherd DN, Roumagnac P, Varsani A. Evolution and ecology of plant viruses. Nat Rev Microbiol (2019) 17:632–44. doi: 10.1038/s41579-019-0232-3
2. French RK, Holmes EC. An ecosystems perspective on virus evolution and emergence. Trends Microbiol (2020) 28:165–75. doi: 10.1016/j.tim.2019.10.010
3. Acosta-Leal R, Duffy S, Xiong Z, Hammond RW, Elena SF. Advances in plant virus evolution: Translating evolutionary insights into better disease management. Phytopathol (2011) 101:1136–48. doi: 10.1094/PHYTO-01-11-0017
4. Manrubia S, Lazaro E. Viral evolution. Phys Life Rev (2006) 3:65–92. doi: 10.1016/j.plrev.2005.11.002
5. Roossinck MJ. Mechanisms of plant virus evolution. Annu Rev Phytopathol (1997) 35:191–209. doi: 10.1146/annurev.phyto.35.1.191
6. Roossinck MJ ed. Plant virus evolution. Berlin, Heidelberg: Springer Berlin Heidelberg (2008). doi: 10.1007/978-3-540-75763-4
7. Drake JW, Holland JJ. Mutation rates among RNA viruses. Proc Natl Acad Sci USA (1999) 96:13910–3. doi: 10.1073/pnas.96.24.13910
8. Sanjuán R, Nebot MR, Chirico N, Mansky LM, Belshaw R. Viral mutation rates. J Virol (2010) 84:9733–48. doi: 10.1128/JVI.00694-10
9. Sanjuán R, Domingo-Calap P. Mechanisms of viral mutation. Cell Mol Life Sci (2016) 73:4433–48. doi: 10.1007/s00018-016-2299-6
10. Tromas N, Elena SF. The rate and spectrum of spontaneous mutations in a plant RNA virus. Genetics (2010) 185:983–9. doi: 10.1534/genetics.110.115915
11. Duffy S. Why are RNA virus mutation rates so damn high? PloS Biol (2018) 16:e3000003. doi: 10.1371/journal.pbio.3000003
12. Steinhauer DA, Domingo E, Holland JJ. Lack of evidence for proofreading mechanisms associated with an RNA virus polymerase. Gene (1992) 122:281–8. doi: 10.1016/0378-1119(92)90216-C
13. Martínez F, Sardanyés J, Elena SF, Daròs J-A. Dynamics of a plant RNA virus intracellular accumulation: Stamping machine vs. geometric replication. Genetics (2011) 188:637–46. doi: 10.1534/genetics.111.129114
14. Roossinck MJ. Lifestyles of plant viruses. Phil Trans R Soc B (2010) 365:1899–905. doi: 10.1098/rstb.2010.0057
15. Duffy S, Shackelton LA, Holmes EC. Rates of evolutionary change in viruses: patterns and determinants. Nat Rev Genet (2008) 9:267–76. doi: 10.1038/nrg2323
16. Nowak MA. What is a quasispecies? Trends Ecol Evol (1992) 7:118–21. doi: 10.1016/0169-5347(92)90145-2
17. Jenkins GM, Rambaut A, Pybus OG, Holmes EC. Rates of molecular evolution in RNA viruses: A quantitative phylogenetic analysis. J Mol Evol (2002) 54:156–65. doi: 10.1007/s00239-001-0064-3
18. Holmes EC. Error thresholds and the constraints to RNA virus evolution. Trends Microbiol (2003) 11:543–6. doi: 10.1016/j.tim.2003.10.006
19. Belshaw R, Pybus OG, Rambaut A. The evolution of genome compression and genomic novelty in RNA viruses. Genome Res (2007) 17:1496–504. doi: 10.1101/gr.6305707
20. Lauber C, Goeman JJ, Parquet M, del C, Thi Nga P, Snijder EJ, et al. The footprint of genome architecture in the largest genome expansion in RNA viruses. PloS Pathog (2013) 9:e1003500. doi: 10.1371/journal.ppat.1003500
21. Rodríguez-Cerezo E, Elena SF, Moya A, García-Arenal F. High genetic stability in natural populations of the plant RNA virus tobacco mild green mosaic virus. J Mol Evol (1991) 32:328–32. doi: 10.1007/BF02102191
22. Fraile A, Escriu F, Aranda MA, Malpica JM, Gibbs AJ, García-Arenal F. A century of tobamovirus evolution in an Australian population of nicotiana glauca. J Virol (1997) 71:8316–20. doi: 10.1128/jvi.71.11.8316-8320.1997
23. García-Arenal F, Fraile A, Malpica JM. Variability and genetic structure of plant virus populations. Annu Rev Phytopathol (2001) 39:157–86. doi: 10.1146/annurev.phyto.39.1.157
24. Hall JS, French R, Hein GL, Morris TJ, Stenger DC. Three distinct mechanisms facilitate genetic isolation of sympatric wheat streak mosaic virus lineages. Virol (2001) 282:230–6. doi: 10.1006/viro.2001.0841
25. Sacristán S, Malpica JM, Fraile A, García-Arenal F. Estimation of population bottlenecks during systemic movement of tobacco mosaic virus in tobacco plants. J Virol (2003) 77:9906–11. doi: 10.1128/JVI.77.18.9906-9911.2003
26. French R, Stenger DC. Evolution of wheat streak mosaic virus: Dynamics of population growth within plants may explain limited variation. Annu Rev Phytopathol (2003) 41:199–214. doi: 10.1146/annurev.phyto.41.052002.095559
27. Li H, Roossinck MJ. Genetic bottlenecks reduce population variation in an experimental RNA virus population. J Virol (2004) 78:10582–7. doi: 10.1128/JVI.78.19.10582-10587.2004
28. Marco CF, Aranda MA. Genetic diversity of a natural population of cucurbit yellow stunting disorder virus. J Gen Virol (2005) 86:815–22. doi: 10.1099/vir.0.80584-0
29. Ali A, Li H, Schneider WL, Sherman DJ, Gray S, Smith D, et al. Analysis of genetic bottlenecks during horizontal transmission of cucumber mosaic virus. J Virol (2006) 80:8345–50. doi: 10.1128/JVI.00568-06
30. Moury B, Fabre F, Senoussi R. Estimation of the number of virus particles transmitted by an insect vector. Proc Natl Acad Sci USA (2007) 104:17891–6. doi: 10.1073/pnas.0702739104
31. Betancourt M, Fereres A, Fraile A, García-Arenal F. Estimation of the effective number of founders that initiate an infection after aphid transmission of a multipartite plant virus. J Virol (2008) 82:12416–21. doi: 10.1128/JVI.01542-08
32. Herranz MC, Al Rwahnih M, Sánchez-Navarro JA, Elena SF, Choueiri E, Myrta A, et al. Low genetic variability in the coat and movement proteins of American plum line pattern virus isolates from different geographic origins. Arch Virol (2008) 153:367–73. doi: 10.1007/s00705-007-1100-4
33. Sardanyés J, Solé RV, Elena SF. Replication mode and landscape topology differentially affect RNA virus mutational load and robustness. J Virol (2009) 83:12579–89. doi: 10.1128/JVI.00767-09
34. Elena SF, Sanjuán R. Adaptive value of high mutation rates of RNA viruses: Separating causes from consequences. J Virol (2005) 79:11555–8. doi: 10.1128/JVI.79.18.11555-11558.2005
35. Furió V, Moya A, Sanjuán R. The cost of replication fidelity in an RNA virus. Proc Natl Acad Sci USA (2005) 102:10233–7. doi: 10.1073/pnas.0501062102
36. Domingo EJJH, Holland JJ. RNA Virus mutations and fitness for survival. Annu Rev Microbiol (1997) 51:15. doi: 10.1146/annurev.micro.51.1.151
37. Holmes EC. The evolution and emergence of RNA viruses. Oxford, United Kingdom: Oxford University Press (2009).
38. Elena SF, Fraile A, García-Arenal F. Evolution and emergence of plant viruses. Adv Virus Res (2014) 88:161–91. doi: 10.1016/B978-0-12-800098-4.00003-9
39. Hoyer JS, Wilkins OW, Munshi A, Wiese E, Dubey D, Renard S, et al. Rapid multilocus adaptation of clonal cabbage leaf curl virus populations to arabidopsis thaliana. Phytobio J (2022) PBIOMES-12-21-0077-R. doi: 10.1094/PBIOMES-12-21-0077-R
40. Whitlock MC, Phillips PC, Moore FBG, Tonsor SJ. Multiple fitness peaks and epistasis. Annu Rev Ecol Evol Syst (1995) 26:601–29. doi: 10.1146/annurev.es.26.110195.003125
41. Sanjuán R, Elena SF. Epistasis correlates to genomic complexity. Proc Natl Acad Sci USA (2006) 103:14402–5. doi: 10.1073/pnas.0604543103
42. Lalić J, Elena SF. Magnitude and sign epistasis among deleterious mutations in a positive-sense plant RNA virus. Heredity (2012) 109:71–7. doi: 10.1038/hdy.2012.15
43. Lalić J, Elena SF. Epistasis between mutations is host-dependent for an RNA virus. Biol Lett (2013) 9:20120396. doi: 10.1098/rsbl.2012.0396
44. Lalić J, Elena SF. The impact of high-order epistasis in the within-host fitness of a positive-sense plant RNA virus. J Evol Biol (2015) 28:2236–47. doi: 10.1111/jeb.12748
45. Bernet GP, Elena SF. Distribution of mutational fitness effects and of epistasis in the 5’ untranslated region of a plant RNA virus. BMC Evol Biol (2015) 15:274. doi: 10.1186/s12862-015-0555-2
46. Rico P, Ivars P, Elena SF, Hernández C. Insights into the selective pressures restricting pelargonium flower break virus genome variability: Evidence for host adaptation. J Virol (2006) 80:8124–32. doi: 10.1128/JVI.00603-06
47. Elena SF, Agudelo-Romero P, Lalic J. The evolution of viruses in multi-host fitness landscapes. TOVJ (2009) 3:1–6. doi: 10.2174/1874357900903010001
48. Montarry J, Doumayrou J, Simon V, Moury B. Genetic background matters: a plant-virus gene-for-gene interaction is strongly influenced by genetic contexts. Mol Plant Pathol (2011) 12:911–20. doi: 10.1111/j.1364-3703.2011.00724.x
49. Visser JAGM, Hermisson J, Wagner GP, Meyers LA, Bagheri‐Chaichian H, Blanchard JL, et al. Perspective: evolution and detection of genetic robustness. Evolution (2003) 57:1959–72. doi: 10.1111/j.0014-3820.2003.tb00377.x
50. Ancel LW, Fontana W. Plasticity, evolvability, and modularity in RNA. J Exp Zool (2000) 288:242–83. doi: 10.1002/1097-010x(20001015)288:3<242::aid-jez5>3.0.co;2-o
51. Butković A, González R, Cobo I, Elena SF. Adaptation of turnip mosaic potyvirus to a specific niche reduces its genetic and environmental robustness. Virus Evol (2020) 6:veaa041. doi: 10.1093/ve/veaa041
52. Montville R, Froissart R, Remold SK, Tenaillon O, Turner PE. Evolution of mutational robustness in an RNA virus. PloS Biol (2005) 3:e381. doi: 10.1371/journal.pbio.0030381
53. Codoñer FM, Darós J-A, Solé RV, Elena SF. The fittest versus the flattest: Experimental confirmation of the quasispecies effect with subviral pathogens. PloS Pathog (2006) 2:e136. doi: 10.1371/journal.ppat.0020136
54. Sanjuán R, Cuevas JM, Furió V, Holmes EC, Moya A. Selection for robustness in mutagenized RNA viruses. PloS Genet (2007) 3:e93. doi: 10.1371/journal.pgen.0030093
55. Stern A, Bianco S, Yeh MT, Wright C, Butcher K, Tang C, et al. Costs and benefits of mutational robustness in RNA viruses. Cell Rep (2014) 8:1026–36. doi: 10.1016/j.celrep.2014.07.011
56. Thyagarajan B, Bloom JD. The inherent mutational tolerance and antigenic evolvability of influenza hemagglutinin. eLife (2014) 3:e03300. doi: 10.7554/eLife.03300
57. Visher E, Whitefield SE, McCrone JT, Fitzsimmons W, Lauring AS. The mutational robustness of influenza a virus. PloS Pathog (2016) 12:e1005856. doi: 10.1371/journal.ppat.1005856
58. Lebeurier G, Hirth L, Hohn B, Hohn T. In vivo recombination of cauliflower mosaic virus DNA. Proc Natl Acad Sci USA (1982) 79:2932–6. doi: 10.1073/pnas.79.9.2932
59. Chenault KD, Melcher U. Phylogenetic relationships reveal recombination among isolates of cauliflower mosaic virus. J Mol Evol (1994) 39:496–505. doi: 10.1007/BF00173419
60. Briddon RW, Bedford ID, Tsai JH, Markham PG. Analysis of the nucleotide sequence of the treehopper-transmitted geminivirus, tomato pseudo-curly top virus, suggests a recombinant origin. Virol (1996) 219:387–94. doi: 10.1006/viro.1996.0264
61. Padidam M, Sawyer S, Fauquet CM. Possible emergence of new geminiviruses by frequent recombination. Virol (1999) 265:218–25. doi: 10.1006/viro.1999.0056
62. Martin DP, Biagini P, Lefeuvre P, Golden M, Roumagnac P, Varsani A. Recombination in eukaryotic single stranded DNA viruses. Viruses (2011) 3:1699–738. doi: 10.3390/v3091699
63. Pérez-Losada M, Arenas M, Galán JC, Palero F, González-Candelas F. Recombination in viruses: Mechanisms, methods of study, and evolutionary consequences. Infect Genet Evol (2015) 30:296–307. doi: 10.1016/j.meegid.2014.12.022
64. Jetzt AE, Yu H, Klarmann GJ, Ron Y, Preston BD, Dougherty JP. High rate of recombination throughout the human immunodeficiency virus type 1 genome. J Virol (2000) 74:1234–40. doi: 10.1128/JVI.74.3.1234-1240.2000
65. García-Andrés S, Accotto GP, Navas-Castillo J, Moriones E. Founder effect, plant host, and recombination shape the emergent population of begomoviruses that cause the tomato yellow leaf curl disease in the Mediterranean basin. Virol (2007) 359:302–12. doi: 10.1016/j.virol.2006.09.030
66. Varsani A, Shepherd DN, Monjane AL, Owor BE, Erdmann JB, Rybicki EP, et al. Recombination, decreased host specificity and increased mobility may have driven the emergence of maize streak virus as an agricultural pathogen. J Gen Virol (2008) 89:2063–74. doi: 10.1099/vir.0.2008/003590-0
67. Lefeuvre P, Lett J-M, Varsani A, Martin DP. Widely conserved recombination patterns among single-stranded DNA viruses. J Virol (2009) 83:2697–707. doi: 10.1128/JVI.02152-08
68. Lefeuvre P, Moriones E. Recombination as a motor of host switches and virus emergence: geminiviruses as case studies. Curr Opin Virol (2015) 10:14–9. doi: 10.1016/j.coviro.2014.12.005
69. Grigoras I, Ginzo AI, del C, Martin DP, Varsani A, Romero J, et al. Genome diversity and evidence of recombination and reassortment in nanoviruses from Europe. J Gen Virol (2014) 95:1178–91. doi: 10.1099/vir.0.063115-0
70. Simon-Loriere E, Holmes EC. Why do RNA viruses recombine? Nat Rev Microbiol (2011) 9:617–26. doi: 10.1038/nrmicro2614
71. Pita JS, Fondong VN, Sangaré A, Otim-Nape GW, Ogwal S, Fauquet CM. Recombination, pseudorecombination and synergism of geminiviruses are determinant keys to the epidemic of severe cassava mosaic disease in Uganda. J Gen Virol (2001) 82:655–65. doi: 10.1099/0022-1317-82-3-655
72. Briddon RW, Patil BL, Bagewadi B, Nawaz-ul-Rehman MS, Fauquet CM. Distinct evolutionary histories of the DNA-a and DNA-b components of bipartite begomoviruses. BMC Evol Biol (2010) 10:97. doi: 10.1186/1471-2148-10-97
73. Silva FN, Lima AT, Rocha CS, Castillo-Urquiza GP, Alves-Júnior M, Zerbini FM. Recombination and pseudorecombination driving the evolution of the begomoviruses tomato severe rugose virus (ToSRV) and tomato rugose mosaic virus (ToRMV): two recombinant DNA-a components sharing the same DNA-b. Virol J (2014) 11:66. doi: 10.1186/1743-422X-11-66
74. Hu J-M, Fu H-C, Lin C-H, Su H-J, Yeh H-H. Reassortment and concerted evolution in banana bunchy top virus genomes. J Virol (2007) 81:1746–61. doi: 10.1128/JVI.01390-06
75. Jeske H. DNA Forms indicate rolling circle and recombination-dependent replication of abutilon mosaic virus. EMBO J (2001) 20:6158–67. doi: 10.1093/emboj/20.21.6158
76. Preiss W, Jeske H. Multitasking in replication is common among geminiviruses. J Virol (2003) 77:2972–80. doi: 10.1128/JVI.77.5.2972-2980.2003
77. McDonald SM, Nelson MI, Turner PE, Patton JT. Reassortment in segmented RNA viruses: mechanisms and outcomes. Nat Rev Microbiol (2016) 14:448–60. doi: 10.1038/nrmicro.2016.46
78. Wang H, Cui X, Cai X, An T. Recombination in positive-strand RNA viruses. Front Microbiol (2022) 13:870759. doi: 10.3389/fmicb.2022.870759
79. Vijaykrishna D, Mukerji R, Smith GJD. RNA Virus reassortment: An evolutionary mechanism for host jumps and immune evasion. PloS Pathog (2015) 11:e1004902. doi: 10.1371/journal.ppat.1004902
80. Bentley K, Evans DJ. Mechanisms and consequences of positive-strand RNA virus recombination. J Gen Virol (2018) 99:1345–56. doi: 10.1099/jgv.0.001142
81. Zanardo LG, Trindade TA, Mar TB, Barbosa TMC, Milanesi DF, Alves MS, et al. Experimental evolution of cowpea mild mottle virus reveals recombination-driven reduction in virulence accompanied by increases in diversity and viral fitness. Virus Res (2021) 303:198389. doi: 10.1016/j.virusres.2021.198389
82. Michalakis Y, Blanc S. The curious strategy of multipartite viruses. Annu Rev Virol (2020) 7:203–18. doi: 10.1146/annurev-virology-010220-063346
83. Zwart MP, Elena SF. Modeling multipartite virus evolution: the genome formula facilitates rapid adaptation to heterogeneous environments. Virus Evol (2020) 6:veaa022. doi: 10.1093/ve/veaa022
84. Lucía-Sanz A, Manrubia S. Multipartite viruses: adaptive trick or evolutionary treat? NPJ Syst Biol Appl (2017) 3:34. doi: 10.1038/s41540-017-0035-y
85. Sicard A, Yvon M, Timchenko T, Gronenborn B, Michalakis Y, Gutierrez S, et al. Gene copy number is differentially regulated in a multipartite virus. Nat Commun (2013) 4:2248. doi: 10.1038/ncomms3248
86. Wu B, Zwart MP, Sánchez-Navarro JA, Elena SF. Within-host evolution of segments ratio for the tripartite genome of alfalfa mosaic virus. Sci Rep (2017) 7:5004. doi: 10.1038/s41598-017-05335-8
87. Sicard A, Pirolles E, Gallet R, Vernerey M-S, Yvon M, Urbino C, et al. A multicellular way of life for a multipartite virus. eLife (2019) 8:e43599. doi: 10.7554/eLife.43599
88. de Ronde D, Butterbach P, Kormelink R. Dominant resistance against plant viruses. Front Plant Sci (2014) 5:307. doi: 10.3389/fpls.2014.00307
89. Moon JY, Park JM. Cross-talk in viral defense signaling in plants. Front Microbiol (2016) 07:2068. doi: 10.3389/fmicb.2016.02068
90. Verchot J. Plant virus infection and the ubiquitin proteasome machinery: Arms race along the endoplasmic reticulum. Viruses (2016) 8:314. doi: 10.3390/v8110314
91. Lewsey M, Palukaitis P, Carr JP. “Plant-virus interactions: Defence and counter-defence,”. In: Roberts JA, editor. Annual plant reviews online. Chichester, UK: John Wiley & Sons, Ltd (2018). p. 134–76. doi: 10.1002/9781119312994.apr0366
92. Paudel DB, Sanfaçon H. Exploring the diversity of mechanisms associated with plant tolerance to virus infection. Front Plant Sci (2018) 9:1575. doi: 10.3389/fpls.2018.01575
93. Zhao S, Li Y. Current understanding of the interplays between host hormones and plant viral infections. PloS Pathog (2021) 17:e1009242. doi: 10.1371/journal.ppat.1009242
94. Lopez-Gomollon S, Baulcombe DC. Roles of RNA silencing in viral and non-viral plant immunity and in the crosstalk between disease resistance systems. Nat Rev Mol Cell Biol (2022). doi: 10.1038/s41580-022-00496-5
95. Cheng X, Wang A. Multifaceted defense and counter-defense in co-evolutionary arms race between plants and viruses. Commun Integr Biol (2017) 10:e1341025. doi: 10.1080/19420889.2017.1341025
96. Wu X, Valli A, García J, Zhou X, Cheng X. The tug-of-War between plants and viruses: Great progress and many remaining questions. Viruses (2019) 11:203. doi: 10.3390/v11030203
97. Elena SF. Evolutionary transitions during RNA virus experimental evolution. Phil Trans R Soc B (2016) 371:20150441. doi: 10.1098/rstb.2015.0441
98. Navarro R, Ambrós S, Butković A, Carrasco JL, González R, Martínez F, et al. Defects in plant immunity modulate the rates and patterns of RNA virus evolution. Virus Evol (2022) 8:veac059. doi: 10.1093/ve/veac059
99. González R, Butković A, Elena SF. Role of host genetic diversity for susceptibility-to-infection in the evolution of virulence of a plant virus. Virus Evol (2019) 5:vez024. doi: 10.1093/ve/vez024
100. Montes N, Vijayan V, Pagán I. Host population structure for tolerance determines the evolution of plant–virus interactions. New Phytol (2021) 231:1570–85. doi: 10.1111/nph.17466
101. Huang Y, Hong H, Xu M, Yan J, Dai J, Wu J, et al. Developmentally regulated arabidopsis thaliana susceptibility to tomato spotted wilt virus infection. Mol Plant Pathol (2020) 21:985–98. doi: 10.1111/mpp.12944
102. Melero I, González R, Elena SF. Host age-dependent evolution of a plant RNA virus. bioRxiv [Preprint] (2022). doi: 10.1101/2022.06.28.497762
103. Wallis CM, Stone AL, Sherman DJ, Damsteegt VD, Gildow FE, Schneider WL. Adaptation of plum pox virus to a herbaceous host (Pisum sativum) following serial passages. J Gen Virol (2007) 88:2839–45. doi: 10.1099/vir.0.82814-0
104. Agudelo-Romero P, de la Iglesia F, Elena SF. The pleiotropic cost of host-specialization in tobacco etch potyvirus. Infect Genet Evol (2008) 8:806–14. doi: 10.1016/j.meegid.2008.07.010
105. Bedhomme S, Lafforgue G, Elena SF. Multihost experimental evolution of a plant RNA virus reveals local adaptation and host-specific mutations. Mol Biol Evol (2012) 29:1481–92. doi: 10.1093/molbev/msr314
106. Calvo M, Malinowski T, García JA. Single amino acid changes in the 6K1-CI region can promote the alternative adaptation of prunus - and nicotiana -propagated plum pox virus c isolates to either host. MPMI (2014) 27:136–49. doi: 10.1094/MPMI-08-13-0242-R
107. Pallas V, García JA. How do plant viruses induce disease? interactions and interference with host components. J Gen Virol (2011) 92:2691–705. doi: 10.1099/vir.0.034603-0
108. Medina-Puche L, Lozano-Duran R. Tailoring the cell: a glimpse of how plant viruses manipulate their hosts. Curr Opin Plant Biol (2019) 52:164–73. doi: 10.1016/j.pbi.2019.09.007
109. Wang A. Dissecting the molecular network of virus-plant interactions: The complex roles of host factors. Annu Rev Phytopathol (2015) 53:45–66. doi: 10.1146/annurev-phyto-080614-120001
110. Cobos A, Montes N, López-Herranz M, Gil-Valle M, Pagán I. Within-host multiplication and speed of colonization as infection traits associated with plant virus vertical transmission. J Virol (2019) 93:e01078–19. doi: 10.1128/JVI.01078-19
111. Pagán I. Transmission through seeds: The unknown life of plant viruses. PloS Pathog (2022) 18:e1010707. doi: 10.1371/journal.ppat.1010707
112. McDaniel L, Maratos M, Farabaugh J. Infection of plants by tobacco mosaic virus. Am Biol Teach (1998) 60:434–9. doi: 10.2307/4450516
113. Fageria M, Nie X, Gallagher A, Singh M. Mechanical transmission of potato virus y (PVY) through seed cutting and plant wounding. Am J Potato Res (2015) 92:143–7. doi: 10.1007/s12230-014-9418-4
114. Ranawaka B, Hayashi S, Waterhouse PM, de Felippes FF. Homo sapiens: The superspreader of plant viral diseases. Viruses (2020) 12:1462. doi: 10.3390/v12121462
115. Ryckebusch F, Peterschmitt M, Granier M, Sauvion N. Alfalfa leaf curl virus is efficiently acquired by its aphid vector aphis craccivora but inefficiently transmitted. J Gen Virol (2021) 102:001516. doi: 10.1099/jgv.0.001516
116. Mauck KE. Variation in virus effects on host plant phenotypes and insect vector behavior: what can it teach us about virus evolution? Curr Opin Virol (2016) 21:114–23. doi: 10.1016/j.coviro.2016.09.002
117. Vasquez DF, Borrero-Echeverry F, Rincon DF. Vector manipulation by a semi-persistent plant virus through disease symptom expression. BioRxiv [Preprint] (2020). doi: 10.1101/2020.08.19.258178
118. Cunniffe NJ, Taylor NP, Hamelin FM, Jeger MJ. Epidemiological and ecological consequences of virus manipulation of host and vector in plant virus transmission. PloS Comput Biol (2021) 17:e1009759. doi: 10.1371/journal.pcbi.1009759
119. Porath-Krause A, Campbell R, Shoemaker L, Sieben A, Strauss AT, Shaw AK, et al. Pliant pathogens: Estimating viral spread when confronted with new vector, host, and environmental conditions. Ecol Evol (2021) 11:1877–87. doi: 10.1002/ece3.7178
120. Gandon S. Evolution and manipulation of vector host choice. Am Nat (2018) 192:23–34. doi: 10.1086/697575
121. Dietzgen R, Mann K, Johnson K. Plant virus–insect vector interactions: Current and potential future research directions. Viruses (2016) 8:303. doi: 10.3390/v8110303
122. Pagán I, Montes N, Milgroom MG, García-Arenal F. Vertical transmission selects for reduced virulence in a plant virus and for increased resistance in the host. PloS Pathog (2014) 10:e1004293. doi: 10.1371/journal.ppat.1004293
123. da Silva W, Kutnjak D, Xu Y, Xu Y, Giovannoni J, Elena SF, et al. Transmission modes affect the population structure of potato virus y in potato. PloS Pathog (2020) 16:e1008608. doi: 10.1371/journal.ppat.1008608
124. Gallet R, Michalakis Y, Blanc S. Vector-transmission of plant viruses and constraints imposed by virus–vector interactions. Curr Opin Virol (2018) 33:144–50. doi: 10.1016/j.coviro.2018.08.005
125. Chare ER, Holmes EC. Selection pressures in the capsid genes of plant RNA viruses reflect mode of transmission. J Gen Virol (2004) 85:3149–57. doi: 10.1099/vir.0.80134-0
126. Monsion B, Froissart R, Michalakis Y, Blanc S. Large Bottleneck size in cauliflower mosaic virus populations during host plant colonization. PloS Pathog (2008) 4:e1000174. doi: 10.1371/journal.ppat.1000174
127. Zwart MP, Elena SF. Matters of size: Genetic bottlenecks in virus infection and their potential impact on evolution. Annu Rev Virol (2015) 2:161–79. doi: 10.1146/annurev-virology-100114-055135
128. Alcaide C, Rabadán MP, Moreno-Pérez MG, Gómez P. Implications of mixed viral infections on plant disease ecology and evolution. Adv Virus Res (2020) 106:145–69. doi: 10.1016/bs.aivir.2020.02.001
129. Harth JE, Ferrari MJ, Helms AM, Tooker JF, Stephenson AG. Zucchini yellow mosaic virus infection limits establishment and severity of powdery mildew in wild populations of cucurbita pepo. Front Plant Sci (2018) 9:792. doi: 10.3389/fpls.2018.00792
130. Tugume AK, Mukasa SB, Valkonen JPT. Mixed infections of four viruses, the incidence and phylogenetic relationships of sweet potato chlorotic fleck virus (Betaflexiviridae) isolates in wild species and sweetpotatoes in Uganda and evidence of distinct isolates in East Africa. PloS One (2016) 11:e0167769. doi: 10.1371/journal.pone.0167769
131. Susi H, Filloux D, Frilander MJ, Roumagnac P, Laine A-L. Diverse and variable virus communities in wild plant populations revealed by metagenomic tools. PeerJ (2019) 7:e6140. doi: 10.7717/peerj.6140
132. Dutt A, Andrivon D, Le May C. Multi-infections, competitive interactions, and pathogen coexistence. Plant Pathol (2022) 71:5–22. doi: 10.1111/ppa.13469
133. Sanjuán R. The social life of viruses. Annu Rev Virol (2021) 8:183–99. doi: 10.1146/annurev-virology-091919-071712
134. Sallinen S, Norberg A, Susi H, Laine A-L. Intraspecific host variation plays a key role in virus community assembly. Nat Commun (2020) 11:5610. doi: 10.1038/s41467-020-19273-z
135. Wintermantel WM, Cortez AA, Anchieta AG, Gulati-Sakhuja A, Hladky LL. Co-Infection by two criniviruses alters accumulation of each virus in a host-specific manner and influences efficiency of virus transmission. Phytopathol (2008) 98:1340–5. doi: 10.1094/PHYTO-98-12-1340
136. Peñaflor MFGV, Mauck KE, Alves KJ, De Moraes CM, Mescher MC. Effects of single and mixed infections of bean pod mottle virus and soybean mosaic virus on host-plant chemistry and host–vector interactions. Funct Ecol (2016) 30:1648–59. doi: 10.1111/1365-2435.12649
137. Ontiveros I, López-Moya JJ, Díaz-Pendón JA. Coinfection of tomato plants with tomato yellow leaf curl virus and tomato chlorosis virus affects the interaction with host and whiteflies. Phytopathol (2022) 112:944–52. doi: 10.1094/PHYTO-08-21-0341-R
138. Bello VH, Ghosh S, Krause-Sakate R, Ghanim M. Competitive interactions between whitefly- and aphid-transmitted poleroviruses within the plant host and the insect vectors. Phytopathol (2021) 111:1042–50. doi: 10.1094/PHYTO-08-20-0369-R
139. Alcaide C, Sardanyés J, Elena SF, Gómez P. Increasing temperature alters the within-host competition of viral strains and influences virus genetic variability. Virus Evol (2021) 7:veab017. doi: 10.1093/ve/veab017
140. Sardanyés J, Alcaide C, Gómez P, Elena SF. Modelling temperature-dependent dynamics of single and mixed infections in a plant virus. Appl Math Model (2022) 102:694–705. doi: 10.1016/j.apm.2021.10.008
141. Suzuki N, Rivero RM, Shulaev V, Blumwald E, Mittler R. Abiotic and biotic stress combinations. N Phytol (2014) 203:32–43. doi: 10.1111/nph.12797
142. Pandey P, Ramegowda V, Senthil-Kumar M. Shared and unique responses of plants to multiple individual stresses and stress combinations: physiological and molecular mechanisms. Front Plant Sci (2015) 6:723. doi: 10.3389/fpls.2015.00723
143. Ramegowda V, Senthil-Kumar M. The interactive effects of simultaneous biotic and abiotic stresses on plants: Mechanistic understanding from drought and pathogen combination. J Plant Physiol (2015) 176:47–54. doi: 10.1016/j.jplph.2014.11.008
144. Zhang H, Sonnewald U. Differences and commonalities of plant responses to single and combined stresses. Plant J (2017) 90:839–55. doi: 10.1111/tpj.13557
145. Prasad A, Sett S, Prasad M. Plant-virus-abiotic stress interactions: A complex interplay. Environ Exp Bot (2022) 199:104869. doi: 10.1016/j.envexpbot.2022.104869
146. van Munster M. Impact of abiotic stresses on plant virus transmission by aphids. Viruses (2020) 12:216. doi: 10.3390/v12020216
147. Harrison BD. The infectivity of extracts made from leaves at intervals after inoculation with viruses. J Genl Microbiol (1956) 15:210–20. doi: 10.1099/00221287-15-1-210
148. Kassanis B. Effects of changing temperature on plant virus diseases. Adv Virus Res (1957) 4:221–41. doi: 10.1016/S0065-3527(08)60600-4
149. Szittya G, Silhavy D, Molnár A, Havelda Z, Lovas A, Lakatos L, et al. Low temperature inhibits RNA silencing-mediated defence by the control of siRNA generation. EMBO J (2003) 22:633–40. doi: 10.1093/emboj/cdg74
150. Király L, Hafez YM, Fodor J, Király Z. Suppression of tobacco mosaic virus-induced hypersensitive-type necrotization in tobacco at high temperature is associated with downregulation of NADPH oxidase and superoxide and stimulation of dehydroascorbate reductase. J Gen Virol (2008) 89:799–808. doi: 10.1099/vir.0.83328-0
151. Wang Y, Bao Z, Zhu Y, Hua J. Analysis of temperature modulation of plant defense against biotrophic microbes. MPMI (2009) 22:498–506. doi: 10.1094/MPMI-22-5-0498
152. Huang L, Ren Q, Sun Y, Ye L, Cao H, Ge F. Lower incidence and severity of tomato virus in elevated CO 2 is accompanied by modulated plant induced defence in tomato: Elevated CO 2 alters plant resistance to virus. Plant Biol (2012) 14:905–13. doi: 10.1111/j.1438-8677.2012.00582.x
153. Prasch CM, Sonnewald U. Simultaneous application of heat, drought, and virus to arabidopsis plants reveals significant shifts in signaling networks. Plant Physiol (2013) 162:1849–66. doi: 10.1104/pp.113.221044
154. Obrępalska-Stęplowska A, Renaut J, Planchon S, Przybylska A, Wieczorek P, Barylski J, et al. Effect of temperature on the pathogenesis, accumulation of viral and satellite RNAs and on plant proteome in peanut stunt virus and satellite RNA-infected plants. Front Plant Sci (2015) 6:903. doi: 10.3389/fpls.2015.00903
155. Del Toro FJ, Aguilar E, Hernández-Walias FJ, Tenllado F, Chung B-N, Canto T. High temperature, high ambient CO2 affect the interactions between three positive-sense RNA viruses and a compatible host differentially, but not their silencing suppression efficiencies. PloS One (2015) 10:e0136062. doi: 10.1371/journal.pone.0136062
156. Chung BN, Choi KS, Ahn JJ, Joa JH, Do KS, Park K-S. Effects of temperature on systemic infection and symptom expression of turnip mosaic virus in Chinese cabbage (Brassica campestris). Plant Pathol J (2015) 31:363–70. doi: 10.5423/PPJ.NT.06.2015.0107
157. Chung BN, Canto T, Tenllado F, Choi KS, Joa JH, Ahn JJ, et al. The effects of high temperature on infection by potato virus y, potato virus A And potato leafroll virus. Plant Pathol J (2016) 32:321–8. doi: 10.5423/PPJ.OA.12.2015.0259
158. Guo H, Huang L, Sun Y, Honggang G, Ge F. The contrasting effects of elevated CO2 on TYLCV infection of tomato genotypes with and without the resistance gene, mi-1.2. Front Plant Sci (2016) 7:1680. doi: 10.3389/fpls.2016.01680
159. Varela ALN, Oliveira JTA, Komatsu S, Silva RGG, Martins TF, Souza PFN, et al. A resistant cowpea (Vigna unguiculata [L.] walp.) genotype became susceptible to cowpea severe mosaic virus (CPSMV) after exposure to salt stress. J Proteom (2019) 194:200–17. doi: 10.1016/j.jprot.2018.11.015
160. Sinha KV, Das SS, Sanan-Mishra N. Overexpression of a RNA silencing suppressor, B2 protein encoded by flock house virus, in tobacco plants results in tolerance to salt stress. Phytoparasitica (2021) 49:299–316. doi: 10.1007/s12600-020-00847-y
161. Amari K, Huang C, Heinlein M. Potential impact of global warming on virus propagation in infected plants and agricultural productivity. Front Plant Sci (2021) 12:649768. doi: 10.3389/fpls.2021.649768
162. Honjo MN, Emura N, Kawagoe T, Sugisaka J, Kamitani M, Nagano AJ, et al. Seasonality of interactions between a plant virus and its host during persistent infection in a natural environment. ISME J (2020) 14:506–18. doi: 10.1038/s41396-019-0519-4
163. Singh MN, Paul Khurana SM, Nagaich BB, Agrawal HO. Environmental factors influencing aphid transmission of potato virus y and potato leafroll virus. Potato Res (1988) 31:501–9. doi: 10.1007/BF02357888
164. Smyrnioudis IN, Harrington R, Katis N, Clark SJ. The effect of drought stress and temperature on spread of barley yellow dwarf virus (BYDV). Agric For Ent (2000) 2:161–6. doi: 10.1046/j.1461-9563.2000.00064.x
165. Dáder B, Fereres A, Moreno A, Trębicki P. Elevated CO2 impacts bell pepper growth with consequences to myzus persicae life history, feeding behaviour and virus transmission ability. Sci Rep (2016) 6:19120. doi: 10.1038/srep19120
166. Nachappa P, Culkin CT, Saya PM, Han J, Nalam VJ. Water stress modulates soybean aphid performance, feeding behavior, and virus transmission in soybean. Front Plant Sci (2016) 7:552. doi: 10.3389/fpls.2016.00552
167. Hong Y, Yi T, Tan X, Su J, Ge F. Microbes affected the TYLCCNV transmission rate by the q biotype whitefly under high O3. Sci Rep (2017) 7:14412. doi: 10.1038/s41598-017-14023-6
168. Yvon M, Vile D, Brault V, Blanc S, van Munster M. Drought reduces transmission of turnip yellows virus, an insect-vectored circulative virus. Virus Res (2017) 241:131–6. doi: 10.1016/j.virusres.2017.07.009
169. Bosquee E, Boullis A, Bertaux M, Francis F, Verheggen FJ. Dispersion of myzus persicae and transmission of potato virus y under elevated CO 2 atmosphere. Entomol Exp Appl (2018) 166:380–5. doi: 10.1111/eea.12661
170. Montes N, Pagán I. Light intensity modulates the efficiency of virus seed transmission through modifications of plant tolerance. Plants (2019) 8:304. doi: 10.3390/plants8090304
171. Peñalver-Cruz A, Garzo E, Prieto-Ruiz I, Díaz-Carro M, Winters A, Moreno A, et al. Feeding behavior, life history, and virus transmission ability of bemisia tabaci Mediterranean species (Hemiptera: Aleyrodidae) under elevated CO 2. Insect Sci (2020) 27:558–70. doi: 10.1111/1744-7917.12661
172. del Toro FJ, Choi KS, Rakhshandehroo F, Aguilar E, Tenllado F, Canto T. Ambient conditions of elevated temperature and CO2 levels are detrimental to the probabilities of transmission by insects of a potato virus y isolate and to its simulated prevalence in the environment. Virol (2019) 530:1–10. doi: 10.1016/j.virol.2019.02.001
173. van Munster M, Yvon M, Vile D, Dader B, Fereres A, Blanc S. Water deficit enhances the transmission of plant viruses by insect vectors. PloS One (2017) 12:e0174398. doi: 10.1371/journal.pone.0174398
174. Bergès SE, Vile D, Vazquez-Rovere C, Blanc S, Yvon M, Bédiée A, et al. Interactions between drought and plant genotype change epidemiological traits of cauliflower mosaic virus. Front Plant Sci (2018) 9:703. doi: 10.3389/fpls.2018.00703
175. Bergès SE, Vile D, Yvon M, Masclef D, Dauzat M, van Munster M. Water deficit changes the relationships between epidemiological traits of cauliflower mosaic virus across diverse arabidopsis thaliana accessions. Sci Rep (2021) 11:24103. doi: 10.1038/s41598-021-03462-x
176. Hily J, Poulicard N, Mora M, Pagán I, García-Arenal F. Environment and host genotype determine the outcome of a plant–virus interaction: from antagonism to mutualism. New Phytol (2016) 209:812–22. doi: 10.1111/nph.13631
177. Xu P, Chen F, Mannas JP, Feldman T, Sumner LW, Roossinck MJ. Virus infection improves drought tolerance. New Phytol (2008) 180:911–21. doi: 10.1111/j.1469-8137.2008.02627.x
178. González R, Butković A, Elena SF. From foes to friends: Viral infections expand the limits of host phenotypic plasticity. Adv Virus Res (2020) 106:85–121. doi: 10.1016/bs.aivir.2020.01.003
179. Mishra R, Shteinberg M, Shkolnik D, Anfoka G, Czosnek H, Gorovits R. Interplay between abiotic (drought) and biotic (virus) stresses in tomato plants. Mol Plant Pathol (2022) 23:475–88. doi: 10.1111/mpp.13172
Keywords: virus evolution, plant virus, virus interactions, viral community, virus transmission
Citation: Butković A and González R (2022) A brief view of factors that affect plant virus evolution. Front. Virol. 2:994057. doi: 10.3389/fviro.2022.994057
Received: 14 July 2022; Accepted: 31 August 2022;
Published: 20 September 2022.
Edited by:
Rafael Sanjuán, University of Valencia, SpainReviewed by:
Selma Gago-Zachert, Martin Luther University of Halle-Wittenberg, GermanyIsrael Pagan, Polytechnic University of Madrid, Spain
Copyright © 2022 Butković and González. This is an open-access article distributed under the terms of the Creative Commons Attribution License (CC BY). The use, distribution or reproduction in other forums is permitted, provided the original author(s) and the copyright owner(s) are credited and that the original publication in this journal is cited, in accordance with accepted academic practice. No use, distribution or reproduction is permitted which does not comply with these terms.
*Correspondence: Anamarija Butković, YW5hbWFyaWphLmJ1dGtvdmljQHBhc3RldXIuZnI=; Rubén González, cnViZW4uZ29uemFsZXpAYmlvLmVucy5wc2wuZXU=
†These authors have contributed equally to this work