- 1Laboratory for Emerging Viruses, Department of Medical Microbiology Infectious Diseases, University of Manitoba, Winnipeg, MB, Canada
- 2Department of Biochemistry and Medical Genetics, University of Manitoba Winnipeg, MB, Canada
The emergence of SARS-CoV-2 in 2019 has resulted in a global pandemic with devastating human health and economic consequences. The development of multiple vaccines, antivirals and supportive care modalities have aided in our efforts to gain control of the pandemic. However, the emergence of multiple variants of concern and spillover into numerous nonhuman animal species could protract the pandemic. Further, these events also increase the difficulty in simultaneously monitoring viral evolution across multiple species and predicting future spillback potential into the human population. Here, we provide historic context regarding the roles of reservoir and intermediate hosts in coronavirus circulation and discuss current knowledge of these for SARS-CoV-2. Increased understanding of SARS-CoV-2 zoonoses are fundamental for efforts to control the global health and economic impacts of COVID-19.
Introduction
History of Betacoronavirus Emergence
Coronaviruses were first identified in 1937 with the identification of avian infectious bronchitis virus (1). The first human coronaviruses (HCoV) were discovered in 1967, and found to be predominantly associated with mild, self-limiting, cold-like illnesses: HCoV-OC43, a Betacoronavirus of subgenus embecovirus (also known as Betacoronavirus 1), and HCoV-229E, an Alphacoronavirus of subgenus Duvinacovirus (2, 3). For more than three decades, HCoVs were not regarded as emerging global health threats (4). However, the emergence of severe acute respiratory syndrome coronavirus (SARS-CoV) in 2002 rapidly changed this view; however, no cases have been identified since 2004 (5). In 2004, two additional HCoVs were identified - HCoV-NL63, an Alphacoronavirus of subgenus Setrecovirus, was identified and found to primarily infect children, the elderly and immunocompromised patients, followed by the identification of HCoV-HKU1, a Betacoronavirus of subgenus Embecovirus, in a patient admitted to hospital in early 2004 (6, 7). Concerns regarding the global health threat of HCoVs were again fueled by the identification of Middle East respiratory syndrome coronavirus (MERS-CoV) infection in humans in 2012; more than 2500 confirmed infections have subsequently been recorded with a case fatality rate of ~35% (8, 9). At the end of 2019, severe acute respiratory syndrome coronavirus 2 (SARS-CoV-2), a novel Betacoronavirus, was identified from a cluster of patients in Wuhan, Hubei Province, China, and has subsequently resulted in a pandemic with devastating economic and public health impacts (10–13). With three HCoVs that have had profound effects on global health having been identified in the last two decades, this demonstrates the pressing need to better understand the zoonotic origins and circulation patterns of these viruses (14).
The emergence of coronaviruses within the human population are believed to have occurred through spillover events from animal reservoirs, such as bats and rodents, to humans (15–18). Reservoir hosts, wherein a pathogen can be maintained through persistent infection, may experience asymptomatic or mild infections while carrying the virus, the mechanism of which is still not well understood, but appears to be due to immune tolerance by the reservoir host (19).
4Transmission of viruses from a reservoir host to a susceptible host can occur directly or indirectly. Direct transmission occurs through direct contact with the reservoir host carrying the pathogen (20); this was observed during the SARS outbreak of 2003 when butchers at live animal markets in Guangdong province, China, had a higher infection rate than the general population (21). Indirect transmission occurs when the virus is shed by the reservoir host to the environment (e.g. airborne or vehicle) or to a vector (e.g. intermediate host) with the potential to be transmitted to humans (20). For example, during the first MERS outbreak in 2012, it is thought that bats infected with MERS-CoV shed the virus in their feces contaminating nearby water and food of domesticated camels (22). The camels are then thought to have transmitted MERS-CoV to the humans they came in contact with (15, 23). The mechanisms by which these cross-species transmission events, or spillovers, occur varies depending on a number of factors such as intermediate host species, geographical locations, climate, and seasonal patterns (24). Many human activities, including urbanization, can increase contact between humans and reservoir/intermediary species and thus increase the likelihood of future spillover events (25). The coronavirus disease 2019 (COVID-19) pandemic caused by SARS-CoV-2 demonstrated the public health and economic tolls following the emergence of SARS-like-coronaviruses (SL-CoVs). In order to minimize the impact of future Betacoronavirus spillover events, we need to better understand the interactions between virus and reservoir, and subsequent interactions between animal reservoirs and humans (26). This review aims to compile and discuss available data on betacoronaviruses and their reservoir and intermediary hosts to highlight knowledge gaps and the importance of ongoing virus surveillance efforts.
SARS-CoV-2, COVID-19 and the Origins of Coronaviruses
In late December 2019, the first cluster of COVID-19 cases in Wuhan, China, was reported by the World Health Organization (WHO) (27–29). While initially described as an atypical pneumonia and SARS-like illness, viral genome sequencing quickly identified the causative agent as a SARS-like coronavirus, initially termed nCoV-2019 but subsequently named SARS-CoV-2 (10, 27, 30). A unique feature that contributed to the rapid global spread of SARS-CoV-2 is the ability for the virus to spread prior to outward signs or symptoms of COVID-19 (31). Within four months of the initial outbreak, SARS-CoV-2 spread across the globe while governments rapidly instituted mitigation measures, including lockdowns and border closures, in an effort to reduce viral spread (32). Though the speed at which SARS-CoV-2 spread took the world by surprise, viral spillover events have been increasing in frequency in recent decades (33). Early analysis identified similarity of SARS-CoV-2 to bat coronaviruses, suggesting that SARS-CoV-2 may have originated as the result of a natural spillover event from bats to humans in late 2019 (34–36). Genomic analyses were conducted on samples from nine patients exhibiting COVID-19 symptoms in Wuhan China (37). Eight of the nine tested patients had either visited or worked at a wet market in the region, which suggested that infections could have been acquired from contact with animals or food products sold at the market (38). There were two initial lineages of SARS-CoV-2 circulating in the early days of wet market circulation termed lineages A and B (29). Lineage B became the dominant lineage linked to early cases from the Hunan market, environmental samples at the time of identifying the outbreak and eventually spread globally (29). While lineage A was associated with cases at other markets in Hunan Province and other areas of China (29 However, the identification of cases from early December not linked to the Huanan seafood market suggest that initial spillover occurred prior to subsequent cases that had contact with the market in mid-to-late December (29). The potential emergence of SARS-CoV-2 across multiple markets mirrors what occurred with the emergence of SARS-CoV with high levels of genetic diversity observed in both cities where SARS-CoV emerged (39–41). This type of emergence pattern suggests SARS-CoV-2 emergence involved multiple contacts with infected animals/traders resulting in multiple spillover events (42). There is evidence to support this with potential infected or susceptible animals transported to/between Wuhan animal markets through supply chains (42). Based on recent models, the first SARS-CoV-2 case in Hubei Province likely occurred between mid-October to mid-November (43). Worobey and colleagues have recently provided supportive evidence that the Huanan market was the geographical epicentre for the pandemic based on both geospatial analysis of the earliest COVID-19 cases in humans as well as spatial analysis of SARS-CoV-2 positive environmental samples with vendors selling live animals (44). There are ongoing investigations into potential intermediate hosts of SARS-CoV-2 which will be discussed in detail in this review (36). The COVID-19 pandemic highlights the continual risks of cross-species transmission and spillover events that can rapidly lead to large-scale outbreaks due to the variability of host-pathogen dynamics and the unpredictability of emerging pathogens.
Virology of Betacoronaviruses
Phylogeny and Genome
Coronaviruses possess the largest genomes of all RNA viruses at 26 – 32 kilobases (kb) in length (45). These are enveloped, single-stranded, positive-sense RNA viruses whose genomes contain at least 6 open reading frames (ORFs) that encode 16 non-structural proteins (NSP), 4 structural proteins, a 5’ cap structure and a 3’ poly (A) tail (46, 47). The family Coronaviridae belongs to the order Nidovirales (48). Within the family Coronaviridae is the subfamily Orthocoronavirinae which is subdivided into four genera, each containing a type species: Alphacoronavirus (alphacoronavirus I), Betacoronavirus (murine coronavirus), Gammacoronavirus (avian coronavirus), and Deltacoronavirus (bulbul coronavirus HKU11) (46). This review will be focused on the Betacoronavirus genera which is divided into five subgenera: Embecovirus, Sarbecovirus, Merbecovirus, Nobecovirus and Hibecovirus with Embecovirus, Sarbecovirus and Merbecovirus relevant to humans, as seen in Figure 1 (10, 26, 49–51).
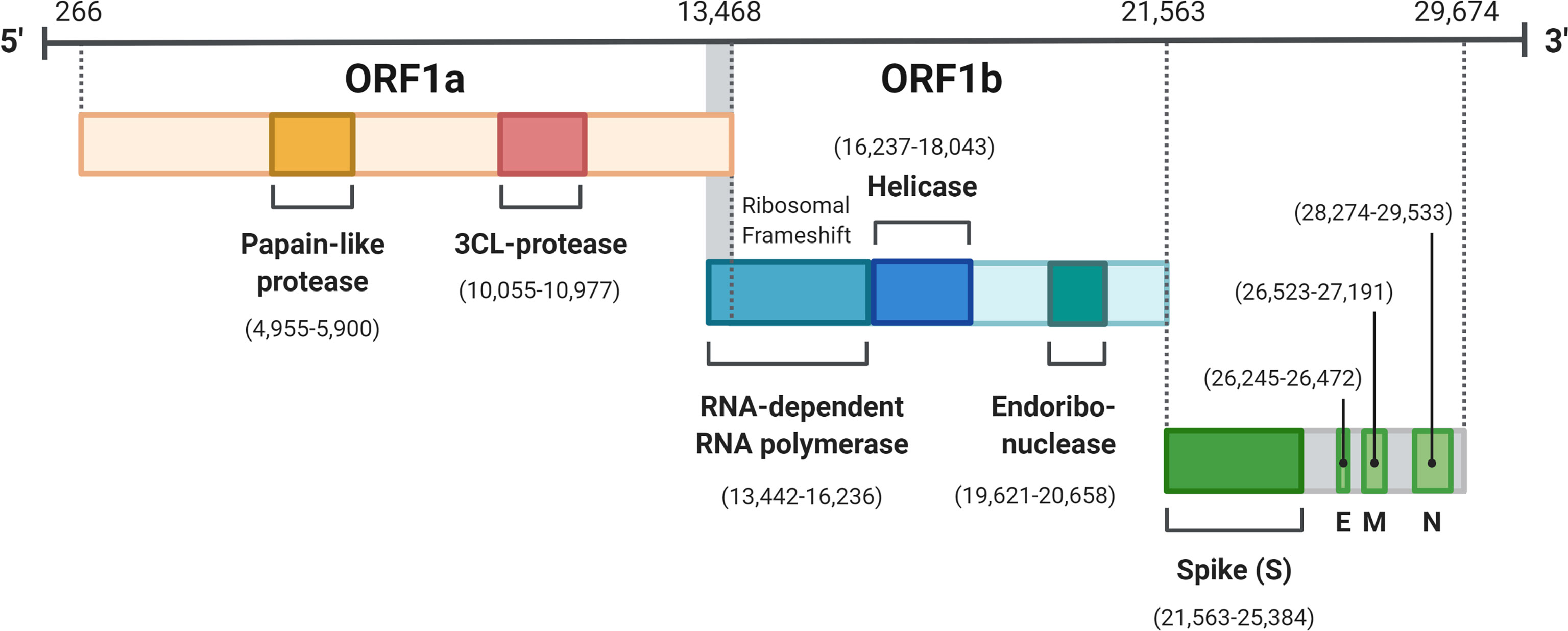
Figure 1 SARS-CoV-2 genome organization. ORF 1a and 1b translated to pp1a and pp1ab are the two polypepdies which are processed into the 16 nonstructual proteins. The four structural proteins are S, spike; E, envelope; M, membrane; N, nucleocapsid. Created with BioRender.com.
Structure and Replication
Betacoronaviruses have a helical nucleocapsid contained within spherical envelopes which are coated with characteristic S glycoproteins protruding from the surface of the envelope (47). When observed via cryo-transmission electron microscopy, the S proteins of the virus have the appearance of a crown, which is where the name coronavirus originates (48). Each spike on the envelope of betacoronaviruses is a homotrimer of the glycosylated S protein. Each monomer S protein contains an S1 binding domain and an S2 fusion domain catalyzing anchoring to the membrane (52). The S protein, a class-1 fusion protein, is processed through the Golgi apparatus where it is heavily glycosylated via an encoded N-terminal signal tag. The M protein, the most abundant protein in the envelope, consists of three transmembrane domains, all of which give the virion its spherical form as well as binding to the nucleocapsid, serving as the scaffold for the virion (53). The E protein consists of 2 domains functioning to assist in the assembly and release of the viral particle from the host cell. Lastly, the N protein consists of two domains allowing binding to the genomic RNA and formation of the nucleocapsid (54). Both the N-terminal and C-terminal domains have RNA binding capacity, however the mechanism by which binding occurs varies. Due to large amounts of observed phosphorylation of the C-terminal domain of the N protein, it is hypothesized that phosphorylation catalyzes a conformational change, increasing the binding affinity between the N protein and viral RNA (55). Phosphorylation of the N protein also acts to tether the replicase-transcriptase complex (RTC) to the viral genome during virion assembly (48). The structural proteins play critical roles in virulence and establishment of infections within reservoirs and other hosts by facilitating viral attachment and entry into host cells.
Betacoronavirus genomes are positive sense and therefore in the same orientation as host mRNA. This allows for direct translation by the host to produce the two viral polyproteins (48) (pp1a and pp1ab) which are then cleaved into the 16 NSPs (Figure 2). Some non-structural proteins exhibit host suppression, for example nsp3 and nsp16 seem to block the interferon-mediated immune response through inhibition of interferon (IFN) pathways (56). Through degradation of host mRNA and suppressing translation of host proteins, nsp1 contributes to the regulation of host cells and the immune response, while also promoting viral production (57). The use of 5’ caps on the viral genome is involved in immune evasion, by disguising as host mRNA which does not activate pattern recognition receptor pathways that would normally lead to the destruction of viral RNA (58, 59). The S protein is critical in viral infection as this is the protein responsible for binding and gaining entry into host cells (60).
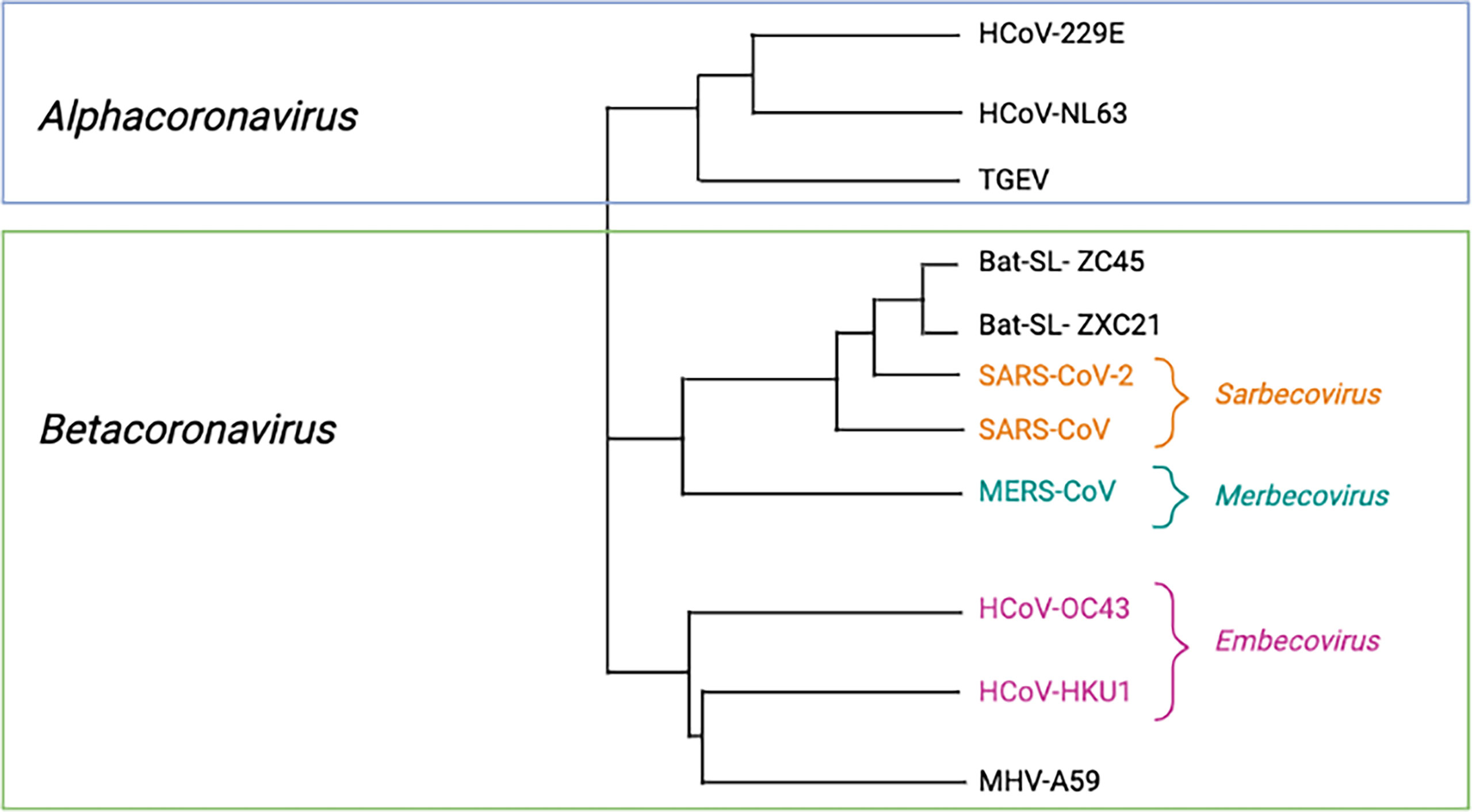
Figure 2 Phylogeneic tree of relevant Orthocoronaviruses. Phylogenetic tree with representative species CoVs. Virus names: HKU, coronavirus identified at Hong Kong University; HCoV, human coronavirus; MERS, Middle Eastern respiratory syndrome; MHV, murine hepatitis virus; SARS, severe acute respiratory syndrome; SL, SARS-like; TGEV, transmissible gastroenteritis virus. Created with BioRender.com.
Betacoronavirus Host Range
The host specificity of betacoronaviruses is determined by their S protein, which binds host receptors that include aminopeptidase N, angiotensin converting enzyme 2 (ACE2) and dipeptidyl peptidase 4 (DPP4) in order to gain entry to host cells (61). Receptor-recognition of the S protein is facilitated by the S1 domain, which is composed of the N-terminal domain (NTD) and C-terminal domain (CTD) (62). The NTD is involved in receptor recognition whereas the CTD contains the receptor-binding domain (RBD) that binds the host cell receptor and determines specificity (63). In the case of SL-CoVs, there is a strong binding affinity between the viral S protein and the host cell receptor ACE2 (52), whereas MERS-like coronaviruses have a strong binding affinity to the DPP4 receptor in the host (64). Reservoir species, such as bats, have a protein homologous to the human ACE2 receptor which may enable transmission of SL-CoVs from bats to humans (65). As different SL-CoVs have varying binding affinity to ACE2 receptors, intermediate host infections can facilitate viral mutation of S protein allowing recognition and binding of ACE2 receptor in humans (66). Following binding of the S protein to its host cell receptor and endosomal uptake of the virus into the cell, the viral genome is subsequently released into the cytoplasm for transcription and replication (67).
ACE2 is the receptor used for cell entry by many Sarbecoviruses, including SARS-CoV and SARS-CoV-2 (65). ACE2 is a type-I transmembrane protein found in epithelial cells of the lung, vascular endothelial cells, and renal tubular epithelium (68, 69). This receptor plays an important role in cardiac pathophysiology acting as a negative regulator of the Renin-angiotensin pathway in the lung, which regulates blood pressure and electrolyte levels (70). Numerous studies have been done to investigate the role of ACE2 in SARS-CoV infection. For example, viral loads in ACE2 knockout mice were far lower than those in the wild-type control mice, suggesting the virus was unable to enter host cells without ACE2 (71). The ability of the S protein to bind ACE2 is largely dependent on the affinity of the viral RBD - which may vary due to mutations in this area of the genome (39, 66, 72). Researchers were able to show that minor variations within the S protein in the RBD of Sarbecoviruses can lead to binding of ACE2 receptors in other animals (intermediate hosts) and humans (73). Investigating the S protein variability within different Sarbecoviruses and SL-CoVs has allowed for greater understanding of how cross-species transmission occurs.
Mechanisms of Betacoronavirus Emergence
Betacoronavirus Spillover Events, Sylvatic Cycles and Synanthropy
There are many examples of coronavirus spillover from intermediate hosts from recent history (23, 26, 47, 74). Many betacoronaviruses are known to originate from bats, including SARS-CoV, BtCoV-WIV1, MERS-CoV, BtCoV-HKU4, BtCoV-HKU5, and Ro-BtCoV-HKU9 (74–77). Since >70% of emerging infectious diseases are zoonotic in origin (78), human-animal interfaces, such as transitional zones bordering wild habitats, are an important factor that should be considered when analyzing viral emergence (79). Li and colleagues assessed bat coronavirus spillover potential in rural districts of Southern China and found serological evidence of SL-CoV antibodies despite the low probability of community exposure to SARS-CoV (79). It was determined that any antibodies detected were likely the result of SL-CoV exposure by cross-species transmission from bats, which are known hosts for these viruses (79). In this example, it had been reported that bats were living within the community which would increase the opportunity for spillover. This is one of many communities that are found within transitional zones, a number that is rapidly increasing due to the encroachment of humans on shrinking wild habitats.
Human incursion into wild habitats is facilitated through activities such as farming, wild animal hunting and rapid transportation (80–82). These activities have a direct effect on the circulation of zoonotic pathogens between their reservoir hosts, intermediate hosts and humans; this is referred to as the sylvatic cycle (83–85). Sylvatic cycles are also affected by climate change as global warming can broaden habitat ranges, allowing species to migrate into geographical locations they previously did not inhabit (25). Broadening of these habitat ranges can lead to increased interactions with humans which increases the probability of a spillover event (86). Human-wildlife interactions will likely continue to increase and therefore the rate of zoonotic pathogen emergence will also increase if these factors are not controlled.
Asymptomatic Nature of Infections in Bats and Implications for Spillover Events
The evidence of bats harbouring and propagating virus while exhibiting little to no signs of disease when considered with the diversity of bat CoVs and close relationship to HCoVs make a case for bats to be considered the reservoir for CoVs (36, 87, 88). Horseshoe bats (Rhinolophus) are the most relevant natural CoV host demonstrated through the diversity of SL-CoVs discovered in several species in Africa, Asia and Europe (89–91). It is not well understood how or why this occurs and therefore, the current research focuses on elucidating the underlying molecular mechanisms through the use of bat cells (15). The work so far suggests that there is early cellular recognition and response to viral replication coupled with moderate suppression of the immune system to tolerate low level infection by these viruses (92). Adaptation of immune system functioning, including variations in the expression levels of type I IFNs, has been demonstrated in different bat species. This may be due to co-evolution resulting from the long-term presence of these viruses among bat species (93). Major histocompatibility complex class one molecules (MHC I) have been found to differ among bat species in both the presentation and structure providing a partial explanation for the different levels of pathogenesis observed in bats (94). Among the differences observed, variations in the MHC I peptide binding groove that recognize distinct peptide epitopes are linked to alterations of bat immune responses (94). Immune suppression by the host in addition to viral evasion of the host’s immune system allows for viral replication to continue uninterrupted, which in turn leads to increased viral shedding (95). These processes are integral to spillover events and thus understanding the complex relationship between the host and the virus is a key part of the transmission dynamic of which our understanding is severely lacking. Further research, specifically in vivo studies, are needed to further our understanding of the molecular mechanisms behind this suppression of symptomatic viral infections observed in numerous bat species.
Evidence for Origins of Betacoronaviruses
Embecoviruses
Human Embecoviruses (previously lineage A Betacoronaviruses) consist of HCoV-OC43 and HCoV-HKU1. Both HCoVs are globally endemic and most often present clinically as the common cold through upper respiratory tract infections (16). Rarely these viruses can cause more severe illnesses such as pneumonia, especially in immunocompromised individuals (96) and detection of HCoV-OC43 in patients with encephalitis hints that this virus has limited neuroinvasive capacity (97–99). Studies suggest both HCoV-OC43 and HCoV-HKU1 originated from rodents (42). This notion has gained support through the discovery of Embecoviruses in rats from Norway and south western China (16, 100–102) in addition to the high degree of sequence homology of HCoV-HKU1 and mouse hepatitis virus (MHV) (16). Further evidence came with the identification of China Rattus CoV HKU24, an Embecovirus found in Norwegian rats in 2015 and it is believed to represent a lineage of CoVs that were present before HCoV-OC43 spilled over into humans in the late 1800s (100). This occupies an early branch of the Embecovirus subgenera and provides more support to suggest that rodents may be an important and understudied reservoir for Embecoviruses.
Sarbecoviruses
SARS-CoV
The Sarbecoviruses consist of SARS-CoV and SARS-CoV-2, two of the most pathogenic coronaviruses identified to date (103). SARS-CoV was originally believed to have emerged from Paguma larvata (masked palm civets) after a case of SARS transmission from masked palm civets to humans (83, 104). However, this changed in 2005 when SL-CoVs were discovered in Chiroptera spp. bats, suggesting that bats may be the true reservoir for SARS-CoV (105) and that civets may instead be an intermediary host. This is not entirely a surprise as there are many coronaviruses which have been identified in various bat species, and SL-CoVs have been found in bat species such as Rhinolophus spp., Hipposideros spp. and Chaerophon spp. (5, 89, 104, 106). More specifically, SL-CoVs that bind ACE2 to mediate cell entry have been found in Rhinolophus sinicus (Chinese horseshoe bats) (65, 107). Further, in 2017 there were 11 new SL-CoVs identified in Rhinolophus sinicus bats from a cave in Yunnan province, China that shared 92-99% sequence homology to SARS-CoV (91). Today, Rhinolophus sinicus is considered the main reservoir of SL-CoVs and should likely be considered the origin point of SARS-CoV as well (108).
SARS-CoV-2
While SARS-CoV-2 has not been identified in bats to date bats are known to be an original source of alpha- and betacoronaviruses, with other Sarbecoviruses similar to SARS-CoV-2 known to be harboured in Rhinolophus spp. bats (109, 110). However there remain only speculations about the origins of SARS-CoV-2 with no direct evidence for the original source, leaving room unfortunately for wild hypotheses around the origins of this virus. The existence of a major virology laboratory (Wuhan Institute of Virology) with a program studying coronaviruses in the city where SARS-CoV-2 was first identified proved to be too large a coincidence for some, subsequently birthing several lab origin hypotheses (14). The first claim is of the virus being of manmade origin involves the observation of human immunodeficiency virus (HIV) sequences in the SARS-CoV-2 genome in a now retracted article by Pradhan et al. and again in another article (111). These findings were quickly refuted through bioinformatic analyses demonstrating the four short sequences occurred at different times and independent of each other and are insufficient evidence for a common ancestor (112, 113). Similarly there have been hypotheses around creation of a virus through gain of function experiments with both recombination and engineered mutations through serial passaging in animal models to obtain these changes to a SL-CoV suggested as possible routes of engineering this recombinant virus (114, 115). However, many of these proposed mutations may be present in other coronaviruses such as the furin cleavage site observed in the RBD of SARS-CoV-2 or a N501Y mutation that would have occurred for efficient replication in animal models (which was not observed in the early stages of the pandemic) and there remains no evidence of engineering within the RBD with the only explanation for their presence being viral evolution (14, 34, 116–121). There has been concern that the emergence of the virus into the human population may have resulted from a laboratory release. The two main hypotheses are, SARS-CoV-2 was created through gain of function experiments on related viruses via serial passaging though this has been refuted (34, 122, 123). The second hypothesis has posited that an accidental laboratory release of SARS-CoV-2 precipitated movement of the virus from the laboratory to the community though this has also been refuted (29, 122). Recent investigation of SARS-CoV-2 genomic diversity by Pekar and colleagues has provided evidence to support the emergence of SARS-CoV-2 through multiple zoonotic events (124). The analysis supports that SARS-CoV-2 lineages A and B resulted from at least two separate spillover events into humans in late 2019.
However, despite these hypotheses there are historical patterns of zoonotic emergence and circulation for coronaviruses as well as the increasing identification of SARS-CoV-2 in numerous nonhuman animal species (14, 125). This is supported by the similarity in the route to human exposure through animal markets as this follows previous coronavirus outbreaks (39). There remains much to be understood about SARS-CoV-2 spillover into humans, and much of our current understanding has relied on epidemiological modeling. Molecular clock modeling of the genome for SARS-CoV-2 and the subsequent mutations suggest a recent emergence, some suggest however, this may not be true due to the highly mutated state of the genome and the effect this may have had on the linearity of the analysis (126–128). As a recent phylogenetic analysis suggests that the lineage of SL-CoVs that SARS-CoV-2 originates from diverged from ancestral bat CoVs sometime between 1948-1982 leaving the door open to the possibility of circulation under the radar (103). It is also unknown if a bat was the only animal involved in the evolution and emergence of SARS-CoV-2. Ongoing and future studies will continue to provide context and nuance for these questions.
In 2020, a group of researchers collected and sequenced samples from Rhinolophus spp. bats in Yunnan province, China, to better understand the zoonotic origins of SARS-CoV-2. Of interest, two bat CoVs had high nucleotide sequence homology to the full-length genome of SARS-CoV-2: RaTG13 (96.1%) and RmYN02 (93.3%) (129, 130). These viruses were collected from Rhinolophus affinis and Rhinolophus malayanus bats, respectively. While RaTG13 maintained high nucleotide sequence homology to the S gene (92.9%) and the RBD (85.3%), RmYN02 showed far lower sequence homology for the S gene (71.9%) and RBD (61.3%). In contrast, they also compared Pangolin viruses GD/2019 and GX/P5L/2017 to SARS-CoV-2 and found lower levels of nucleotide homology for the whole genome (GX/P5L/2017 = 85.2%) but high levels of amino acid sequence homology in the S gene (GX/P5L/2017 = 92.4% and GD/2019 = 90.7%) and RBD (GD/2019 = 97.4% and GX/P5L/2017 = 86.8%) (130, 131). It seems that while pangolins may have been involved in a recombination event affecting the RBD, the high level of homology between the genome sequences of SARS-CoV-2 and bat CoVs suggest that the virus originated from bats rather than pangolins (132, 133).
To further investigate the bat origin hypothesis, investigations have assessed the selective pressures driving viral adaptation and evolution. MacLean and colleagues identified weak purifying selection among SARS-CoV-2 strains from the first 11 months of the pandemic (36). For the spike protein, diversifying selection occurred deeper in the phylogenetic branches of the Sarbecovirus clade, leading to a very generalist SARS-CoV-2 virus and is supported by the wide host range (36, 134). Others have suggested that the closest ancestral divergence of this virus is likely to be approximately four or five decades ago, based on similarities to bat CoVs RmYN02 and RaTG13, respectively (135, 136). Additionally, investigations have looked at the CG suppression within the viral genome (cytosine followed by a guanine in the 5’ to 3’ direction) due to their link to antiviral mechanisms in the host (137). Many vertebrate RNA viruses demonstrate similar patterns of 5’-CG-3’ dinucleotide suppression, where there is a lower number of CG dinucleotides than expected, as found within vertebrate genomes (137). Further, Takata and colleagues suggested that this suppression may highlight an adaptation with RNA viruses to evade host immunity through reduced discrimination of self- and non-self RNA (137). Analysis of the Sarbecovirus clades identified a phylogenetic shift towards CG suppression followed by an elevated substitution rate (36). This suggests an increase in selective pressure in the surrounding environment at the time (138, 139). These evolutionary factors taken together suggest that the virus evolved prior to the spillover event into humans, rather than through human-to-human infection during the pandemic (103). Thus, it is likely that SARS-CoV-2 was highly capable of infecting humans prior to the spillover event which led to the first COVID-19 case (140). Adding to this, the fact that SARS-CoV-2 can transmit readily to other animals (mink, cats, dogs, etc) - and in some cases transmit back to humans (mink) - supports the possibility of a generalist virus, where the virus already contained generalist ACE2 binding properties that could aid in efficient host switch across multiple intermediate animal species (139, 141).
Merbecoviruses
The Merbecoviruses (formerly lineage C betacoronaviruses) consist of MERS-CoV, the only known Merbecovirus to infect humans, as well as several bat CoVs including HKU4 and HKU5 (61). The Egyptian tomb bat, Taphozous perfortus is believed to be the reservoir species for MERS-CoV (142). Fecal samples collected from a trapped Egyptian tomb bat tested positive for MERS-CoV in the same region that the MERS-CoV index patient was found (143). These findings have been supported by studies demonstrating that the bat receptor dipeptidyl-peptidase 4 (DPP4) potentially co-evolved with MERS-CoV, supporting Egyptian tomb bats as an appropriate reservoir for MERS-CoV (102). HK4U and HK5U share over 65% amino acid sequence identity to MERS-CoV (61, 144); HKU4 was first identified in lesser bamboo bats, while HKU5 has been found to circulate in Japanese pipistrelle bats (7). Using molecular clock analysis, studies have shown MERS-CoV, HKU4, and HKU5 have a common ancestor as recently as several centuries ago (100). Due to this phylogenetic ancestry, researchers believe the possibility of a species jump is high enough to warrant further surveillance (61, 145).
Intermediate Hosts of Betacoronaviruses and Their Role in Spillover Events
It is generally accepted that HCoV spillover to humans is often facilitated through an intermediate host (4, 102). Intermediate hosts are animals that are biologically similar to the natural reservoir host and more frequently come in contact with humans; therefore, these hosts allow for opportunity to mutate to a form that is more easily transmissible to humans (146). There is some consensus on the intermediate hosts of three of the five human betacoronaviruses: SARS-CoV (masked palm civets), MERS-CoV (camelids) and HCoV-OC43 (bovine) (21, 74, 147). The same cannot be said for the remaining two HCoVs, HCoV-HKU1 and SARS-CoV-2, where intermediate hosts are the subject of continuing investigation. It is thought that each of these viruses have individually spilled over into intermediate hosts facilitating zoonotic transfer to humans (130, 132). The known and proposed intermediate hosts of human betacoronaviruses are presented in the following sections. Nonhuman animal species that have been reported to be susceptible to Betacoronavirus infection are presented in Table 1.
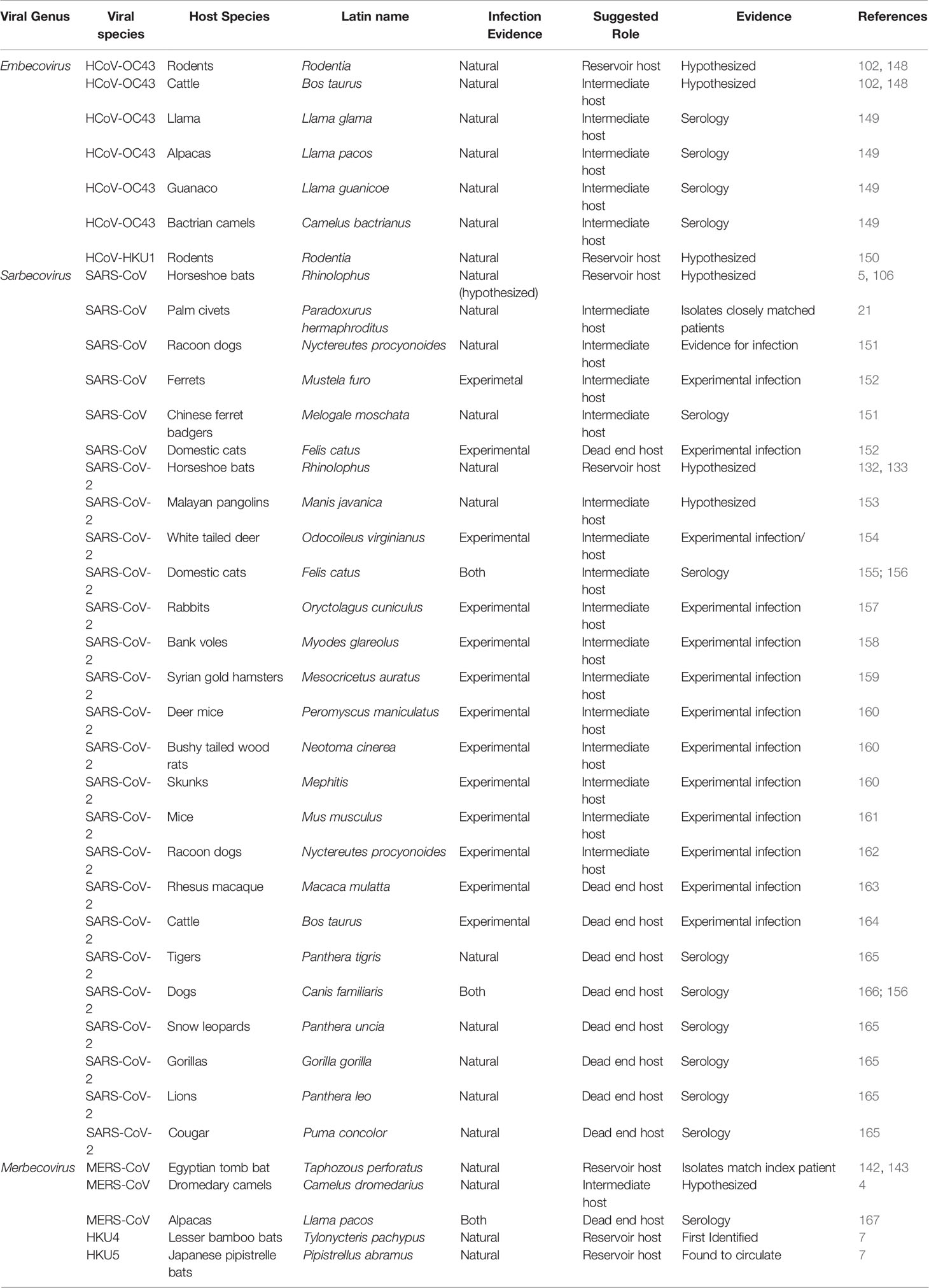
Table 1 Nonhuman animal species identified to be susceptible to Betacoronavirus infection. Source of evidence, confirmation of infection and suggested role in viral transmission are provided.
Embecovirus Intermediate Hosts
Both known human embecoviruses (HCoV-OC43 and HCoV-HKU1) are suspected to use an intermediate host in their emergence. While it is generally accepted that HCoV-HKU1 likely originated from rodents as it is related to MHV (16, 100, 101), there is no evidence that points to an intermediate host for this virus. One hypothesis is that HKU1 was transmitted directly from rodents to humans, either through contact with rodent excrement, exposure to their blood or other biological products, or through consumption of rodents (150). HCoV-OC43 is also believed to have originated in rodents however, this virus has 96.6% sequence identity to Bovine Coronavirus (BCoV) (16, 168) and is therefore thought to have used cattle as an intermediate host between rodents and humans (102, 148). Estimations based on evolutionary rates of betacoronaviruses place HCoV-OC43 spillover from cattle into humans around 1890 (16, 148, 169). Serological studies have shown that HCoV-OC43 antibodies are also present in other animals including llamas, alpacas, guanaco, and Bactrian camels (149, 170). These animals may have been exposed to HCoV-OC43 or BCoV if they had previous close contact with cattle, demonstrating the wide intermediary host range embecoviruses and other betacoronaviruses can have (171).
Sarbecovirus Intermediate Hosts – SARS-CoV
In 2002, the first recorded emergence of a Betacoronavirus that was highly pathogenic in humans occurred (80). The emergence of SARS-CoV and subsequent epidemic ignited interest in the origin of betacoronaviruses as prior to these, HCoVs were not considered global health threats (4). Zoonotic transmission of SARS-CoV was considered early on due to the fact that many of the early SARS cases appeared to have a common connection to an animal market in Shenzhen, China (80, 102). An investigation into animals sold at the market identified both SARS-CoV and another SARS-related coronavirus in palm civets (Paguma larvata) and racoon dogs (Nyctereutes procynoides), and SARS-CoV antibodies in Chinese ferret badgers (Melogale moschata) (80, 151, 172). Evidence of palm civet-to-human transmission arose during a small SARS outbreak in Guangdong Province in 2003-2004 where four individuals tested positive for SARS-CoV and three of these patients had either direct or indirect contact with masked palm civets (21). While this provided evidence that palm civets may have been a source for the 2003-2004 SARS outbreak, it does not indicate that civets were the original source for the jump of SARS-CoV to humans. It was found that SARS-CoV isolates from palm civets at the Shenzhen market had 99.6% sequence identity to SARS-CoV samples collected from infected patients (21); however, there was a 1000-fold difference in their affinity for the human ACE2 receptor (173, 174). Additionally, while ~80% of the animals tested in Guangdong had SARS-CoV antibodies, infectious virus was not recovered from additional samples collected from wild and farmed palm civets (40). These data suggest that SARS-CoV does not naturally circulate in palm civet populations and it was likely introduced in the markets through storage of animals in close quarters (21, 80, 171). The high degree of sequence homology and lack of mutations suggests that SARS-CoV may have recently spilled over into masked palm civets not long before spillover to humans (175).
Additional animal species susceptible to SARS-CoV have been identified including house cats, ferrets, Chinese ferret badgers and racoon dogs; however, limited study has occurred in these species (152, 171). The route and timing of SARS-CoV transmission to raccoon dogs in the Shenzhen market remains unknown (21). Investigation of raccoon dogs at a market in Guangzhou, China, did identify SARS-CoV antibodies (21). To date, available evidence supports the hypothesis that masked palm civets acted as an intermediate host for SARS-CoV.
Sarbecovirus Intermediate Hosts – SARS-CoV-2
Investigations on SARS-CoV-2 origins and potential intermediate hosts have focused on the linkages of patients to the Huanan market in Wuhan (176). Approximately two-thirds of patients from the initial cluster of COVID-19 cases in 2019 had visited this market prior to contracting the virus while others were in contact with people involved in live animal trade (133). There were also similarities to the emergence of SARS-CoV in Foshan and Guangzhou, Guangdong, China in 2002 (177). Yunnan province has been hypothesized as the originating region for SARS-CoV and SARS-CoV-2 since the discovery of animal traders with prevalence of SL-CoVs and high IgG levels there in 2003 (177). Sampling of various animals pointed to bats as one of the early candidates for zoonotic transmission due to the presence of SL-CoVs with high levels of homology to SARS-CoV-2 like viruses (RaTG13 and RmYN02) (178). In-depth phylogenetic analysis suggests that SARS-CoV-2 is a generalist virus which has been circulating in bats for some time, and that there was little mutation and adaptation required to be capable of infecting humans (36). This is highlighted by the fact that many animals can host productive SARS-CoV-2 infections and some animals, such as mink, are capable of transmitting the virus back to humans (141). In the following sections, we will examine the possible intermediate hosts of SARS-CoV-2 and what they may have contributed to the evolution of this virus.
Potential Intermediate Hosts and Animals With Role in Viral Dissemination
We summarize the growing evidence of SARS-CoV-2 circulation and transmission patterns across various animal species in Figure 3. Ferrets (Mustela putorius furo) are instrumental models for studying respiratory pathogenicity and transmission of viruses (181). Experimental studies show that ferrets are susceptible to SARS-CoV-2 infection of the upper respiratory tract early in the disease course (166). However, clinical signs of illness appear to be uncommon with transient fever, and mild respiratory symptoms reported (166, 182–185). There is still substantial viral shedding observed in the respiratory tract during infection making ferrets a useful model for studying transmission. During infection ferrets have been shown to infect healthy ferrets in close contact through the high degree of viral shedding in their feces, nasal secretions, urine and saliva (12). Direct and indirect transmission of SARS-CoV-2 has been demonstrated in ferrets and healthy ferrets become symptomatic following direct contact with infected ferrets, though separation of the animals with maintenance of shared airspace did result in some viral positivity in the absence of symptoms (139). The lack of clinical signs of illness in conjunction with the high amount of viral shedding suggest mostly asymptomatic infection and evidence that mustelids such as ferrets may have played a role as an intermediate host in SARS-CoV-2 emergence. While infection of ferret badgers has not been documented, they have a high degree of similarity to other animals which are permissive to SARS-CoV-2 infection and therefore are still an animal of interest.
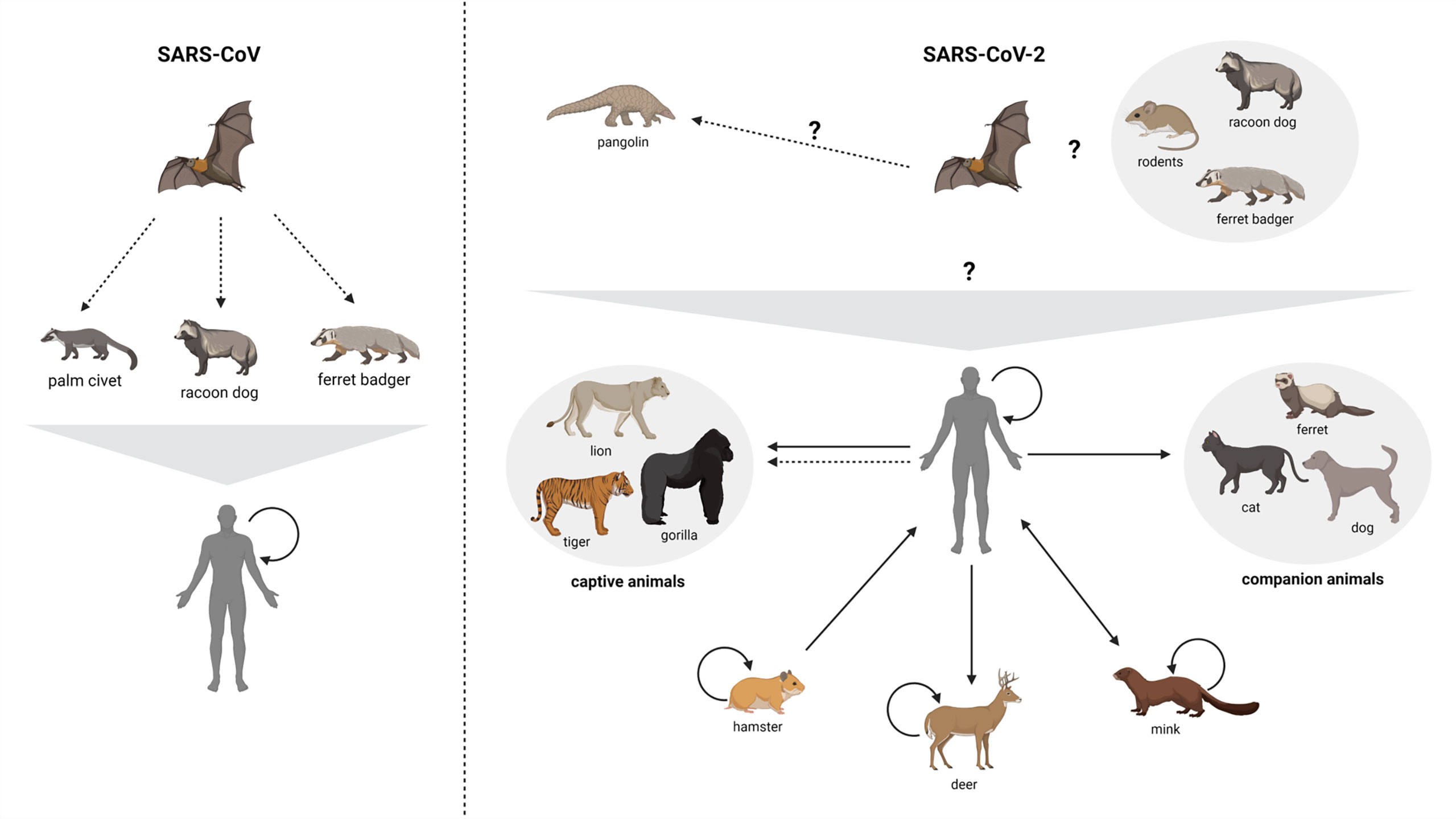
Figure 3 Zoonotic circulation of SARS-CoV and SARS-CoV-2. Suspected and confirmed zoonotic circulation of both SARS-CoV and SARS-CoV-2 are presented. Suspected routes of transmission are presented by dashed arrows. Question marks designate routes that have yet to be demonstrated by direct or indirect methods (though are theorized or probable). Note that there is epidemiological and genetic evidence for some human-to-captive animal transmission events (179, 180) while others are suspected/probable. Solid lines represent confirmed transmission events. Created with BioRender.com.
Mink (Neovison vison) are also a potential SARS-CoV-2 intermediate host given the SARS-CoV-2 detection and onward transmission in mink from two farms in the Netherlands (186). The animals showed respiratory and gastrointestinal symptoms (187), and approximately 1.2-2.4% of animals succumbed to infection, the majority of which were pregnant females (188). Necropsies found signs of interstitial pneumonia and lung lesions (186). There is supportive evidence that SARS-CoV-2 was introduced to mink by farm workers with subsequent transmission between the animals (186). This was supported by sequencing of viral samples from both mink and humans which revealed significant homology between the viruses present in each sample (187). This became a cause for concern as it was also observed that variants of the virus had been transmitted from mink to humans (189), suggesting an intermediate host which could support viral recombination and rapid transmission (190). Transmission between humans and mink was observed in ten countries: Canada, Denmark, France, Greece, Italy, Lithuania, the Netherlands, Spain, Sweden and the USA (190, 191). It was determined early on that the mode of transmission was not direct as mink are housed separately. Viral RNA was also detected in early collection of inhalable dust samples indicating a potential route of exposure (186).
Raccoon dogs (Nyctereutes procyonoides) initially gained attention as possible intermediate hosts of SARS-CoV (21, 80, 151) for two main reasons: i) susceptibility to SARS-CoV infection (192); and ii) high ACE2 sequence similarity between raccoon dogs and humans (193, 194). Raccoon dogs can be productively infected with SARS-CoV-2 through experimental inoculation and transmit the virus to other healthy animals (162). However, clinical signs of illness such as increased body temperature or weight loss were not observed and virus isolated from infected animals had 100% sequence homology to the viral inoculum.
The susceptibility of white tailed deer (Odocoileus virginianus) to experimental infection was assessed in early 2021 (154). Intranasal inoculation of deer resulted in virus shedding through nasal secretions and transmission to naïve deer who later seroconverted. Indirect transmission was also observed as virus was identified in nasal swabs and transiently in fecal samples from naïve fawns housed in separate pens from infected animals, and which included plexiglass barriers (154). Additionally, RNA was detectable in infected animal tissues for up to 21 days post-infection. Other woodland animals such as bushy-tailed wood rats and skunks are capable of shedding virus in respiratory secretions (160). As these animals are shedding the virus and, in some cases, appear capable of transmitting to their surrounding environments, their role as intermediate hosts and potential future sources of spillback of novel SARS-CoV-2 variants to humans needs to be investigated.
Rodents are believed to have played a significant role in the emergence of human Embecoviruses (HCoVs OC43 and HKU1) and have therefore been hypothesized as potential intermediate hosts of SARS-CoV-2. Early studies suggested that mice were unlikely to be an intermediate host candidate for SARS-CoV-2 (130, 195, 196). However, subsequent work by Griffin and colleagues has demonstrated that deer mice are susceptible to infection resulting in asymptomatic or mild disease (197). Infected deer mice could also transmit virus to co-housed naïve mice. More recently, Stone and colleagues demonstrated that the SARS-CoV-2 Alpha and Beta variants could result in productive infection of wild-type C57BL/6 mice via intranasal inoculation (198). Alternatively, Syrian hamsters are susceptible to infection with SARS-CoV-2 presenting with clinical disease that resembles respiratory infection in humans as well as weight loss (159, 199–201). One study demonstrated that Syrian hamsters that had previous infection to SARS-CoV-2 had protection from re-infection with reduced replication in the upper respiratory tract and no observed transmission to naïve contact animals (202). Thus, hamsters have become widely used for investigations of SARS-CoV-2 infection (7, 161, 200). A recent preprint provides evidence for transmission of SARS-CoV-2 to humans from naturally infected hamsters, though the implications of this on the initiation of new human-to-human transmission chains remains to be determined (203).
Susceptibility of Additional Species to SARS-CoV-2
ACE2’s ubiquitous presence within the animal kingdom and its high degree of similarity amongst mammalian species is a major contributor to the spread of betacoronaviruses around the world, most recently with SARS-CoV-2 (204). In silico modelling predicted the following species may exhibit binding affinity for the SARS-CoV-2 S protein: cats, cattle, monkeys, dogs, pigs, horses, sheep, and rabbits (in decreasing order) (205). Many other animals have been infected with SARS-CoV-2 experimentally or in nature and are able to host a productive infection. These cases will be discussed in the following paragraphs.
Initial attention was given to domestic animals such as dogs and cats as they would be high risk to transmit to humans if they could host a productive infection. Reports showed domestic cats had tested positive for SARS-CoV-2 in Europe, Asia, and North America (163, 205). Cats have also been shown to spread SARS-CoV-2 via respiratory droplets (166) though viral RNA has also been detected in nasal, oropharyngeal and rectal swabs (155, 206). This was concerning due to the proximity of cats to humans however, one study showed that cats exposed to the virus did not exhibit any symptoms (88, 207). There is conflicting data as to whether or not cats are asymptomatic throughout the infection (208), but if cats are in fact asymptomatic they may not be effective intermediate hosts. As for dogs, studies show that SARS-CoV-2 replicates poorly in these animals and that healthy dogs who come into contact with SARS-CoV-2-positive dogs remain seronegative (166). Overall, infected dogs showed no obvious signs of infection and most often did not have detectable levels of RNA present in biological samples (88, 166, 206). In an attempt to determine why this occurs, scientists analyzed the ACE2 receptor in dogs and found 5 amino acid substitutions but none within the RBD; therefore, this is believed to have minimal impact on binding of the S protein (196, 209). Based on the studies conducted so far it is unlikely cats or dogs played a role in the emergence of SARS-CoV-2.
Investigations into agriculturally significant animals such as cattle were an important consideration as there are previous bovine links to coronaviruses in addition to an identified bovine coronavirus (210). For the most part, cattle did not seem to be able to host a productive SARS-CoV-2 infection with little to no viral replication or immune response detected in these animals. They also did not exhibit clinical signs of infection or transmit to nearby animals (164). Cattle and other farm animals are important to consider as they are commonly in contact with humans. One study in particular looked at susceptibility to SARS-CoV-2 in poultry and determined that chickens along with turkeys, geese, quail and ducks are not susceptible to infection (166, 211). Similarly, many studies have investigated SARS-CoV-2 infection in pigs, and it seems that they are resistant to infection as there are no signs of infection, no pathology and no viral RNA detected (212–214).
SARS-CoV-2 infections in a variety of zoo animals have been widely reported, including tigers, snow leopards, lions, gorillas and pumas. Animal testing followed signs of respiratory symptoms of disease and while transmission between animals in the same enclosures was reported, widespread transmission was observed in any of these cases (215). It is thought that the animals contracted the virus from asymptomatic workers.
Aside from these examples of natural infection, much of what we know of SARS-CoV-2 infection in animals has come from experimental infections. This has led to the discovery that rhesus monkeys can be experimentally infected (163). Two Rhesus macaque species (M. fascularis and M. mallata) and one common marmoset species (C. jacchus) were able to be infected with SARS-CoV-2 via the intratracheal and intranasal routes and demonstrated clinical signs of infection (216, 217). It has been reported old world monkeys are susceptible to infection and new world monkeys have much lower susceptibility when compared to human ACE2 there are 4 amino acid differences (218). Nonhuman primates have similar clinical presentation of COVID-19 to humans including viral replication in the upper and lower respiratory tracts, inflammation and focal edema among other less frequent signs and symptoms (13). Due to these clinical presentations, the phylogenetic relatedness of nonhuman primates to humans, and previous reports of HCoV-OC43 infections in nonhuman primates, concerns have been raised regarding the potential impacts of SARS-CoV-2 on endangered species, including great apes (88, 219).
Bank voles have been experimentally infected and had viral RNA present in nasal tissue for up to 21 days post infection, though no transmission to animals in direct contact was observed (220). Rabbits have been reported to be susceptible to infection with SARS-CoV-2 (164) however, there is conflicting data stating that in fact white cotton tail rabbits are resistant to infection (160). Additional studies have determined that squirrels (211), raccoons and black-tailed prairie dogs (160) are resistant to SARS-CoV-2 infection [Recommended further readings on the topic of experimental and natural infections of animals. (221–223)].
Evidence Regarding the Role of Pangolins
Malayan pangolins (Manis Javanica) and Chinese pangolins (Manis pentadactyla) are considered vulnerable or critically endangered as they are the most trafficked mammals in the world (224) due to the use of their meat and scales in traditional African and Chinese medicine (225). Pangolins are solitary, nocturnal mammals that dwell in remote sandy forests (226) away from humans (227), and thus, poaching provides the only real opportunity for human contact with wild populations (37). Pangolins were proposed as an intermediate host in the emergence of SARS-CoV-2 in the early days of the pandemic due to a high degree of sequence identity to pangolin-CoVs, their interaction with bat populations and their presence at the Hunan wet market during the time when the first documented cases of SARS-CoV-2 were identified (153).
Sequence similarity was identified by two independent studies that identified SL-CoV in Malayan pangolins confiscated from illegal wildlife traders (77, 228). The identified SL-CoVs had 85.5-92.4% sequence identity to SARS-CoV-2; however, these pangolin-CoVs had 97.4% sequence identity to the RBD of the SARS-CoV-2 S protein (specifically pangolin CoVs GD/P1L and GD/P2S) (77, 131, 132, 228). Specifically, the six amino acid residues in the RBD of the S1 protein identified as critical for binding the host ACE2 receptor are conserved between pangolin-CoVs and SARS-CoV-2 (34, 131, 229). This high degree of sequence identity in the RBDs of SARS-CoV-2 and pangolin-CoVs suggests either a recombination event occurred or these viruses have highly similar RBDs due to convergent evolution (77, 228). For the recombination hypothesis it was posited to have occurred between pangolin-CoVs from Malayan pangolins and RaTG13 from R. affinis bats due to the high degree of similarity in the RBD of pangolin-CoVs to SARS-CoV-2, while SL-CoV RaTG13 is the closest relative to SARS-CoV-2 albeit with a distinct RBD site of the S protein (77, 131, 132, 228). However, when analyzed through alignment there is low nucleotide similarity compared to the high amino acid similarity and many misalignments within the sequence, suggesting recombination is unlikely and merely convergently evolved features of these distinct SARS-CoV-2 and pangolin CoV viruses (77, 103, 172, 229, 230). Although recombination has been observed in CoVs there is no evidence in the S protein of the SARS-CoV-2 lineage and instead appears to be an artifact of the metagenomic analysis that detected recombination initially (103, 123). Supporting the idea of convergent evolution, a recent analysis of SL-CoV RaTG13, pangolin-CoVs and SARS-CoV-2 finding that pangolin-CoVs diverged from the SL-CoVs approximately 150-180 years ago (103). If recombination occurred it would be expected for these viruses to have a higher degree of similarity in specific regions, which has not been observed (29, 132, 174). For recombination to occur it also implies the viruses, in this case SL-CoV RaTG13 and pangolin-CoV GD/P1L or GD/P2S, would co-infect the same cell; however, R. affinis bats (Species SL-CoV RaTG13 was found in) do occupy the same natural range as M. javanica pangolins (122, 231, 232).
To date, pangolin-CoVs have not been found in Chinese pangolins, which share the same habitat range as R. affinis bats (228). There is limited evidence for cohabitation of bats with pangolins of any species and does not appear very common, with one study finding bats and pangolins in Gabon within the same burrows (233). Follow up studies to detect pangolin-CoVs in Malayan pangolins have been unsuccessful with a study of 334 confiscated pangolins finding no sarbecoviruses raising doubts around the role of pangolins in the emergence of SARS-CoV-2 (123, 228, 234). Further to this point, a study of wild Malayan pangolins in Malaysia determined that there is no observed circulation for betacoronaviruses, filoviruses and flaviviruses, suggesting that any detected sarbecoviruses are most likely acquired through contact within the smuggling trade of these animals (234). The animals that pangolin-CoVs have been isolated were found to be either severely ill or already dead (82, 228). Animals becoming severely ill is not what is expected for intermediate hosts as this would greatly limit viral amplification through host immune response and ultimately death, as well as interactions with other species limiting ability to spread the virus to other species (110). Some have presented the possibility for contributing factors to the severe illness and death of the tested animals, including due to the stressful environment they find themselves in in close contact with other animals and humans as well as other viruses that are commonly found in pangolins including Sendai virus (235, 236). While some studies have shown the presence of coronaviruses in captivity (82), there remains no evidence of SARS-CoV-2 or SL-CoVs in wild pangolins. More to that point, Yuan et al. claim that pangolins were not present at the Huanan Wildlife Market during the initial identification of SARS-CoV-2 in 2019 (237). In addition to the lack of circulating coronaviruses, the solitary lifestyle of pangolins makes it difficult for pangolin populations to amplify pangolin-CoVs, which suggests they would be a poor intermediate or reservoir host candidate from an epidemiological standpoint (103). Considering the studies conducted to date on the role of Malayan pangolins in the emergence of SARS-CoV-2 as an intermediate host does not line up with the hypothesis and the initial phylogenetic reports have been refuted.
No Intermediate Host
There is continuing evaluation of the susceptibility and onward transmission potential of various animal species to SARS-CoV-2. However, it has also been hypothesized that SARS-CoV-2 emergence may have occurred in the absence of an intermediate host (36). Here, the authors suggest that diversifying positive selection was limited to the early phase of the pandemic and that SARS-CoV-2 has much weaker purifying selection as compared to related sarbecoviruses. The authors further suggest that the transmission of SARS-CoV-2 to additional nonhuman animal species supports the generation of a generalist virus in a bat reservoir.
Ongoing research continues to actively investigate the hypotheses for the emergence and transmission of SARS-CoV-2 demonstrating the collective effort of the scientific community to determine the origins of this pandemic, there is still much to be known and continued collaboration will be essential.
Merbecoviruses Intermediate Hosts
Dromedary camels were identified as the intermediate host of MERS-CoV after camels tested positive for virus with 100% sequence identity to viral isolates from humans that were infected through close contact with the animals (238). MERS-CoV can be transmitted from dromedary camels to humans via respiratory droplets as well as the fecal/oral route (4). It is postulated that MERS-CoV can spread between camelids when kept in close contact (167) but more studies are needed to confirm this (239). MERS-CoV outbreaks usually occur when an infected camel transmits to a human, who can then transmit to their close contacts (240). During the 2012 outbreak there were multiple lineages of MERS-CoV circulating, indicating that multiple zoonotic transmission events may have contributed to this outbreak (241). Dudas et al. estimate that hundreds of spillover events between camels and humans have resulted in the cases of MERS that we know of today (242). This is supported by the fact that neutralizing MERS-CoV antibodies have been found in dromedary camels in Africa, Asia and the Middle East (102, 243, 244). Experimental and serological studies have also shown that alpacas can be infected with MERS-CoV and may potentially serve as intermediary hosts however, this appears to be restricted to regions where MERS-CoV is endemic in dromedary camels (149, 167, 239, 245). Since MERS-CoV is believed to have spilled over into dromedary camels from bats more than 20 years ago (106, 244), it is thought that the camel coronavirus adapted to the dromedary camel hosts and therefore caused minimal health effects (242, 245, 246). For this reason, dromedary camels have been reclassified as the reservoir hosts of MERS-CoV (242, 245, 246).
Discussion
Human Interactions With Zoonotic Coronavirus Hosts
Understanding how viruses emerge and the role that humans play in these emergence events are of central importance to early detection and prevention of large-scale outbreaks (204). As the human population grows, a greater area of land is being converted into farmland and housing to meet the demand. This naturally means that humans, and the domesticated animals that accompany them, will be overlapping more with wild animals like bats (15). Sharing habitats like this facilitates cross-species transmission of viruses and emergence of infectious diseases (4). However, eliminating all human contact with possible Betacoronavirus hosts is not feasible due to urbanization and in many cases the cultural and economic importance of these animals (247). A recent example of this is the role of dromedary camels in MERS-CoV circulation and spillover. Camels are a central part of the livelihoods of many families, providing a source of transportation, food, and commodity trade (248). Culling dromedary camels to prevent the spread of MERS-CoV would negatively impact the well-being of the people in these communities and the local economy (248). Preventative strategies for MERS instead relies on recognizing illness in camels, rapid testing, national surveillance, international communication, and the development of vaccines for dromedary camels to decrease MERS-CoV transmission to humans (249).
Surveillance and Monitoring for Future Zoonotic Outbreaks
The increase in globalization and urbanization over the last half-century have led to a dramatic change in both the mode and the frequency in which humans and animals come in contact. As we continue to piece together the roles of intermediate hosts in zoonoses, including for SARS-CoV-2, we must continue to examine the magnitude of their role in viral emergence and subsequent public health emergencies. Public health responses to outbreaks have primarily been reactionary in nature (i.e. quarantines, travel restrictions, vaccine and therapeutic development) as opposed to preventative including global surveillance, pandemic prediction, early warnings and control (250, 251). Precautionary rather than reactive responses would seem far more logical given the global health and economic toll of the COVID-19 pandemic. One Health approaches to emerging virus surveillance and preparedness are critical in this regard given that ~ 60% of emerging infectious disease outbreaks are of zoonotic origin (domestic or wildlife), with almost 75% of zoonotic emergence events originating with wildlife (78). However, it is imperative to consider the potential for bidirectional transmission between humans and animals for emerging viruses, such as has been identified for SARS-CoV-2, when considering outbreak response and containment plans (252). Indeed, observations from mink and white-tailed deer during the COVID-19 pandemic highlight the importance of consideration for the complexities of routes of transmission and reservoir-host interactions (141, 253). However, there is ongoing development of emerging infectious disease surveillance systems which utilize wildlife screening techniques to sample and test for various pathogens in healthy animals, and monitor morbidity and mortality rates of regional animal species (254). This information can then be collated and relayed to additional research groups conducting similar surveillance programs across the globe (255). Understanding that the most effective way to combat future outbreaks is with a preventative/precautionary approach as opposed to a responsive/reactive approach, researchers continue to lobby for more holistic approaches to monitor animal-human interfaces (256). There are independently-funded holistic programs such as the One Health Project which takes into consideration environmental, animal, and human factors to understand and monitor disease spread from animals-to-humans as well as from human-to-animal (256). Surveillance work is currently focused on regions that have high potential for inter-species viral transmission. Some of these environmental factors include regions experiencing extreme effects of climate change, or regions with tropical rainforests, high population density, and high numbers of mammalian species (251). Based on these criteria, Sub-Saharan Africa, Southeast-Asia, and Latin America are the focus of current research into surveillance and are considered high risk regions for emerging infectious disease (EID) events to occur (257, 258). It will be of paramount importance to invest into monitoring and surveillance of animals in these regions, ensuring that any future outbreaks are detected early and minimized.
A One Health approach to limiting the exposure and spread of emerging disease is a better model for outbreak/pandemic response because despite the availability of vaccines for SARS-CoV-2 these alone are not enough with the likelihood of endemicity due to demonstrated instances of zooanthroponosis (259). As new variants of SARS-CoV-2 continue to emerge the protective coverage that vaccines have provided will continue to wane with diverging spike proteins and have demonstrated a comprehensive One Health approach is needed to bring the current and future pandemics under control (260). An approach of this kind would encompass public health and human vaccination campaigns already being implemented globally as well as animal vaccination campaigns and wildlife surveillance (259). The development and integration of animal vaccines for zoonotic viruses could have great impacts on zoonoses and zooanthroponoses as well as impacting reservoir establishments. Animal vaccination against SARS-CoV-2 have already been approved and demonstrated to be safe and effective with administration of the Zoetis vaccine being utilized at zoos, on mink farms as well as domestically (261). Early detection and prevention measures should be implemented within a One Health model beyond humans alone as humans are only a part of the story of outbreaks and pandemics (223, 260). Global collaboration and cooperation are necessary in tracing the source and will be necessary for mitigation of outbreaks and pandemics in the future (125).
Conclusions
Many facets of coronaviruses are yet to be uncovered. Here we provide a collection of evidence for the complexities of coronavirus transmission patterns across species. There are some important clarifications that have been identified for sarbecoviruses. For example, embecoviruses likely emerged from rodents with cattle acting as an intermediate host in HCoV-OC43 whereas HCoV-HKU1 is suspected to have used an intermediate host that has yet to be identified. The transmission of SARS-CoV to humans utilized an intermediate host believed to be palm civets or raccoon dogs and MERS-CoV utilizes dromedary camels as a reservoir and intermediate host following an original spillover event from bats to camels. Other human coronaviruses pose a greater challenge. While there is evidence that SARS-CoV-2 originated from bats, and there was likely the involvement of an intermediate host, the specific details of these events have yet to be conclusively determined.
It is critical to appreciate that while spillover events of viruses that rapidly become public health threats occur unpredictably, this should not preclude global investments in robust surveillance and prediction systems, in particular within regions that are ‘hot spots’ for emergence events. For decades infectious disease experts have studied how increased contact with wild animals – whether it be through deforestation, climate change, or other factors – leads to new diseases spilling over to humans. In our fast-growing world, expansion is not slowing (80–82). We should not expect the spillover rate of infectious diseases to humans to slow either. Thus, several strategies need to be utilized to limit the economic, health and social impacts of these events.
● Reduction of transmission risk through preventative hygiene measures and public health education campaigns in place early on following identification of a spillover of a new virus.
● Have a framework in place, infrastructure and trade agreements in place to allow for accelerated development and deployment of therapeutics and vaccines to all countries to shorten the duration of a pandemic through reduced risk of variants.
● Global education campaigns for risks of contact with certain wild and domestic hunted/farmed species as well as the sale and consumption of species. In addition to the appropriate aid work to find safe sustainable alternatives for impacted communities
● The identification of reservoir and intermediate hosts of known infectious diseases is important for the prevention of future viral outbreaks/pandemics through understanding the viral ecology of animal populations and the circulating pathogens within these animal communities.
● Proactively reduce the risk of spillover events through implementing ecosystem stewardship measures in conjunction with prioritizing climate change reduction measures and biodiversity conservation measures. While also ensuring access to proper sanitation, safe and sustainable food sources and clean water sources.
Author Contributions
All authors listed have made a substantial, direct, and intellectual contribution to the work and approved it for publication.
Funding
JK is funded by a Tier 2 Canada Research Chair in the Molecular Pathogenesis of Emerging and Re-Emerging Viruses provided by the Canadian Institutes of Health Research (Grant no. 950-231498), by the Natural Sciences and Engineering Research Council Discovery Grant (RGPIN-2018-06036) and from the Coronavirus Variants Rapid Response Network (FRN# 175622). MA was funded by an internship through the MITACS Accelerate program (FR53425).
Conflict of Interest
The authors declare that the research was conducted in the absence of any commercial or financial relationships that could be construed as a potential conflict of interest.
Publisher’s Note
All claims expressed in this article are solely those of the authors and do not necessarily represent those of their affiliated organizations, or those of the publisher, the editors and the reviewers. Any product that may be evaluated in this article, or claim that may be made by its manufacturer, is not guaranteed or endorsed by the publisher.
References
1. Beaudette FR, Hudson CB. Cultivation of the Virus of Infectious Bronchitis. J Am Vet Med Assoc (1937) 90:51–8.
2. Almeida JD, Tyrrell DA. The Morphology of Three Previously Uncharacterized Human Respiratory Viruses That Grow in Organ Culture. J Gen Virol (1967) 1:175–8. doi: 10.1099/0022-1317-1-2-175
3. Mcintosh K, Becker WB, Chanock RM. Growth in Suckling-Mouse Brain of “IBV-Like” Viruses From Patients With Upper Respiratory Tract Disease. Proc Natl Acad Sci (1967) 58:2268–73. doi: 10.1073/pnas.58.6.2268
4. Ye ZW, Yuan S, Yuen KS, Fung SY, Chan CP, Jin DY. Zoonotic Origins of Human Coronaviruses. Int J Biol Sci (2020) 16:1686–97. doi: 10.7150/ijbs.45472
5. Ar Gouilh M, Puechmaille SJ, Diancourt L, Vandenbogaert M, Serra-Cobo J, Lopez Roig M, et al. SARS-CoV Related Betacoronavirus and Diverse Alphacoronavirus Members Found in Western Old-World. Virology (2018) 517:88–97. doi: 10.1016/j.virol.2018.01.014
6. Fouchier RA, Hartwig NG, Bestebroer TM, Niemeyer B, De Jong JC, Simon JH, et al. A Previously Undescribed Coronavirus Associated With Respiratory Disease in Humans. Proc Natl Acad Sci U.S.A. (2004) 101:6212–6. doi: 10.1073/pnas.0400762101
7. Woo PC, Lau SK, Chu CM, Chan KH, Tsoi HW, Huang Y, et al. Characterization and Complete Genome Sequence of a Novel Coronavirus, Coronavirus HKU1, From Patients With Pneumonia. J Virol (2005) 79:884–95. doi: 10.1128/JVI.79.2.884-895.2005
8. De Wit E, Van Doremalen N, Falzarano D, Munster VJ. SARS and MERS: Recent Insights Into Emerging Coronaviruses. Nat Rev Microbiol (2016) 14:523–34. doi: 10.1038/nrmicro.2016.81
9. European Centre for Disease Prevention and Control. MERS-CoV Situation Update, 7 January 2022 [Online] (2022). European Centre for Disease PRevention and Control. Available at: https://www.ecdc.europa.eu/en/middle-east-respiratory-syndrome-coronavirus-mers-cov-situation-update (Accessed January 28, 2022).
10. Coronaviridae Study Group of the International Committee on Taxonomy of Viruses. The Species Severe Acute Respiratory Syndrome-Related Coronavirus: Classifying 2019-Ncov and Naming it SARS-CoV-2. Nat Microbiol (2020) 5:536–44. doi: 10.1038/s41564-020-0695-z
11. Dong E, Du H, Gardner L. An Interactive Web-Based Dashboard to Track COVID-19 in Real Time. Lancet Infect Dis (2020) 20:533–4. doi: 10.1016/S1473-3099(20)30120-1
12. Zheng J. SARS-CoV-2: An Emerging Coronavirus That Causes a Global Threat. Int J Biol Sci (2020) 16:1678–85. doi: 10.7150/ijbs.45053
13. Korath ADJ, Janda J, Untersmayr E, Sokolowska M, Feleszko W, Agache I, et al. One Health: EAACI Position Paper on Coronaviruses at the Human-Animal Interface, With a Specific Focus on Comparative and Zoonotic Aspects of SARS-Cov-2. Allergy (2021) 77:55–71. doi: 10.1111/all.14991
14. Holmes EC, Goldstein SA, Rasmussen AL, Robertson DL, Crits-Christoph A, Wertheim JO, et al. The Origins of SARS-CoV-2: A Critical Review. Cell (2021) 184:4848–56. doi: 10.1016/j.cell.2021.08.017
15. Han HJ, Wen HL, Zhou CM, Chen FF, Luo LM, Liu JW, et al. Bats as Reservoirs of Severe Emerging Infectious Diseases. Virus Res (2015) 205:1–6. doi: 10.1016/j.virusres.2015.05.006
16. Corman VM, Muth D, Niemeyer D, Drosten C. Hosts and Sources of Endemic Human Coronaviruses. Adv Virus Res (2018) 100:163–88. doi: 10.1016/bs.aivir.2018.01.001
17. Gryseels S, De Bruyn L, Gyselings R, Calvignac-Spencer S, Leendertz FH, Leirs H. Risk of Human-to-Wildlife Transmission of SARS-CoV-2. Mamm Rev (2020) 272–92. doi: 10.20944/preprints202005.0141.v1
18. Goraichuk IV, Arefiev V, Stegniy BT, Gerilovych AP. Zoonotic and Reverse Zoonotic Transmissibility of SARS-CoV-2. Virus Res (2021) 302:198473. doi: 10.1016/j.virusres.2021.198473
19. Mandl JN, Ahmed R, Barreiro LB, Daszak P, Epstein JH, Virgin HW, et al. Reservoir Host Immune Responses to Emerging Zoonotic Viruses. Cell (2015) 160:20–35. doi: 10.1016/j.cell.2014.12.003
20. Cortez MH, Weitz JS. Distinguishing Between Indirect and Direct Modes of Transmission Using Epidemiological Time Series. Am Nat (2013) 181:E43–54. doi: 10.1086/668826
21. Shi Z, Hu Z. A Review of Studies on Animal Reservoirs of the SARS Coronavirus. Virus Res (2008) 133:74–87. doi: 10.1016/j.virusres.2007.03.012
22. Mackay IM, Arden KE. MERS Coronavirus: Diagnostics, Epidemiology and Transmission. Virol J (2015) 12:222. doi: 10.1186/s12985-015-0439-5
23. Azhar EI, El-Kafrawy SA, Farraj SA, Hassan AM, Al-Saeed MS, Hashem AM, et al. Evidence for Camel-to-Human Transmission of MERS Coronavirus. N Engl J Med (2014) 370:2499–505. doi: 10.1056/NEJMoa1401505
24. Prada D, Boyd V, Baker ML, O’dea M, Jackson B. Viral Diversity of Microbats Within the South West Botanical Province of Western Australia. Viruses (2019) 11:1157. doi: 10.3390/v11121157
25. Mcmichael C. Climate Change-Related Migration and Infectious Disease. Virulence (2015) 6:548–53. doi: 10.1080/21505594.2015.1021539
26. Wong ACP, Li X, Lau SKP, Woo PCY. Global Epidemiology of Bat Coronaviruses. Viruses (2019) 11:174. doi: 10.3390/v11020174
27. Who. Pneumonia of Unknown Cause - China (2020). Available at: https://www.who.int/csr/don/05-january-2020-pneumonia-of-unkown-cause-china/en/ (Accessed October 10 2020).
28. Adil MT, Rahman R, Whitelaw D, Jain V, Al-Taan O, Rashid F, et al. SARS-CoV-2 and the Pandemic of COVID-19. Postgrad Med J (2021) 97:110–6. doi: 10.1136/postgradmedj-2020-138386
29. World Health Organization. “WHO-Convened Global Study of Origins of SARS-CoV-2: Joint WHO-China Study 14 January-10 February 2021 Joint Report”. Geneva: World Health Organization (2021).
30. Zhang YZ, Holmes EC. A Genomic Perspective on the Origin and Emergence of SARS-CoV-2. Cell (2020) 181:223–7. doi: 10.1016/j.cell.2020.03.035
31. Irving AT, Welburn SC. SARS-CoV-2 and Zoonotic Preparedness: Unknown Knowns? Infect Microb Dis (2021) 3:30–1. doi: 10.1097/IM9.0000000000000051
32. Arora S, Bhaukhandi KD, Mishra PK. Coronavirus Lockdown Helped the Environment to Bounce Back. Sci Total Environ (2020) 742:140573. doi: 10.1016/j.scitotenv.2020.140573
33. Falcinelli SD, Chertow DS, Kindrachuk J. Integration of Global Analyses of Host Molecular Responses With Clinical Data To Evaluate Pathogenesis and Advance Therapies for Emerging and Re-Emerging Viral Infections. ACS Infect Dis (2016) 2:787–99. doi: 10.1021/acsinfecdis.6b00104
34. Andersen KG, Rambaut A, Lipkin WI, Holmes EC, Garry RF. The Proximal Origin of SARS-CoV-2. Nat Med (2020) 26:450–2. doi: 10.1038/s41591-020-0820-9
35. Wu F, Zhao S, Yu B, Chen YM, Wang W, Song ZG, et al. A New Coronavirus Associated With Human Respiratory Disease in China. Nature (2020) 579:265–9. doi: 10.1038/s41586-020-2008-3
36. Maclean OA, Lytras S, Weaver S, Singer JB, Boni MF, Lemey P, et al. Natural Selection in the Evolution of SARS-CoV-2 in Bats Created a Generalist Virus and Highly Capable Human Pathogen. PloS Biol (2021) 19:e3001115. doi: 10.1371/journal.pbio.3001115
37. Lu R, Zhao X, Li J, Niu P, Yang B, Wu H, et al. Genomic Characterisation and Epidemiology of 2019 Novel Coronavirus: Implications for Virus Origins and Receptor Binding. Lancet (2020) 395:565–74. doi: 10.1016/S0140-6736(20)30251-8
38. Alanagreh L, Alzoughool F, Atoum M. The Human Coronavirus Disease COVID-19: Its Origin, Characteristics, and Insights Into Potential Drugs and Its Mechanisms. Pathogens (2020) 9:331. doi: 10.3390/pathogens9050331
39. Guan Y, Zheng BJ, He YQ, Liu XL, Zhuang ZX, Cheung CL, et al. Isolation and Characterization of Viruses Related to the SARS Coronavirus From Animals in Southern China. Science (2003) 302:276–8. doi: 10.1126/science.1087139
40. Tu CC, Crameri G, Kong X, Chen J, Sun Y, Yu M, et al. Antibodies to SARS Coronavirus in Civets. Emerg Infect Dis (2004) 10:2244–8. doi: 10.3201/eid1012.040520
41. Yaqing HE. Surveillance of SARS Coronavirus Among Wild Animal Sold in Dongmen Market in Shenzhen City. Dis Surveillance (2004) 19:287–91. doi: 10.3784/j.issn.1003-9961.2004.8.287
42. Xiao X, Newman C, Buesching CD, Macdonald DW, Zhou ZM. Animal Sales From Wuhan Wet Markets Immediately Prior to the COVID-19 Pandemic. Sci Rep (2021) 11:11898. doi: 10.1038/s41598-021-91470-2
43. Pekar J, Worobey M, Moshiri N, Scheffler K, Wertheim JO. Timing the SARS-CoV-2 Index Case in Hubei Province. Science (2021) 372:412–7. doi: 10.1126/science.abf8003
44. Worobey M, Levy JI, Mallpica Serrano LM, Crits-Christoph A, Pekar JE, Goldstein SA, et al. The Huanan Market was the Epicenter of SARS-CoV-2 Emergence. Zenodo (2022) 1–67. doi: 10.5281/zenodo.6299600
45. Mousavizadeh L, Ghasemi S. Genotype and Phenotype of COVID-19: Their Roles in Pathogenesis. J Microbiol Immunol Infect (2021) 54:159–63. doi: 10.1016/j.jmii.2020.03.022
46. Brian DA, Baric RS. Coronavirus Genome Structure and Replication. Curr Top Microbiol Immunol (2005) 287:1–30. doi: 10.1007/3-540-26765-4_1
47. Su S, Wong G, Shi W, Liu J, Lai ACK, Zhou J, et al. Epidemiology, Genetic Recombination, and Pathogenesis of Coronaviruses. Trends Microbiol (2016) 24:490–502. doi: 10.1016/j.tim.2016.03.003
48. Fehr AR, Perlman S. Coronaviruses: An Overview of Their Replication and Pathogenesis. Methods Mol Biol (2015) 1282:1–23. doi: 10.1007/978-1-4939-2438-7_1
49. Chen Y, Liu Q, Guo D. Emerging Coronaviruses: Genome Structure, Replication, and Pathogenesis. J Med Virol (2020) 92:418–23. doi: 10.1002/jmv.25681
50. International Committee on Taxonomy of Viruses. ICTV Master Species List 2020.V1 (2020). Available at: https://talk.ictvonline.org/files/master-species-lists/m/msl/12314 (Accessed 6 December, 2021).
51. Llanes A, Restrepo CM, Caballero Z, Rajeev S, Kennedy MA, Lleonart R. Betacoronavirus Genomes: How Genomic Information has Been Used to Deal With Past Outbreaks and the COVID-19 Pandemic. Int J Mol Sci (2020) 21:4546. doi: 10.3390/ijms21124546
52. Sun J, He WT, Wang L, Lai A, Ji X, Zhai X, et al. COVID-19: Epidemiology, Evolution, and Cross-Disciplinary Perspectives. Trends Mol Med (2020) 26:483–95. doi: 10.1016/j.molmed.2020.02.008
53. Barcena M, Oostergetel GT, Bartelink W, Faas FG, Verkleij A, Rottier PJ, et al. Cryo-Electron Tomography of Mouse Hepatitis Virus: Insights Into the Structure of the Coronavirion. Proc Natl Acad Sci U.S.A. (2009) 106:582–7. doi: 10.1073/pnas.0805270106
54. Bhat EA, Khan J, Sajjad N, Ali A, Aldakeel FM, Mateen A, et al. SARS-CoV-2: Insight in Genome Structure, Pathogenesis and Viral Receptor Binding Analysis - An Updated Review. Int Immunopharmacol (2021) 95:107493. doi: 10.1016/j.intimp.2021.107493
55. Chang CK, Chen CM, Chiang MH, Hsu YL, Huang TH. Transient Oligomerization of the SARS-CoV N Protein–Implication for Virus Ribonucleoprotein Packaging. PloS One (2013) 8:e65045. doi: 10.1371/journal.pone.0065045
56. De Wilde AH, Raj VS, Oudshoorn D, Bestebroer TM, Van Nieuwkoop S, Limpens R, et al. MERS-Coronavirus Replication Induces Severe In Vitro Cytopathology and is Strongly Inhibited by Cyclosporin A or Interferon-Alpha Treatment. J Gen Virol (2013) 94:1749–60. doi: 10.1099/vir.0.052910-0
57. Kamitani W, Huang C, Narayanan K, Lokugamage KG, Makino S. A Two-Pronged Strategy to Suppress Host Protein Synthesis by SARS Coronavirus Nsp1 Protein. Nat Struct Mol Biol (2009) 16:1134–40. doi: 10.1038/nsmb.1680
58. Kindler E, Thiel V. To Sense or Not to Sense Viral RNA–essentials of Coronavirus Innate Immune Evasion. Curr Opin Microbiol (2014) 20:69–75. doi: 10.1016/j.mib.2014.05.005
59. Kasuga Y, Zhu B, Jang KJ, Yoo JS. Innate Immune Sensing of Coronavirus and Viral Evasion Strategies. Exp Mol Med (2021) 53:723–36. doi: 10.1038/s12276-021-00602-1
60. Verma J, Subbarao N. A Comparative Study of Human Betacoronavirus Spike Proteins: Structure, Function and Therapeutics. Arch Virol (2021) 166:697–714. doi: 10.1007/s00705-021-04961-y
61. Wang Q, Qi J, Yuan Y, Xuan Y, Han P, Wan Y, et al. Bat Origins of MERS-CoV Supported by Bat Coronavirus HKU4 Usage of Human Receptor CD26. Cell Host Microbe (2014) 16:328–37. doi: 10.1016/j.chom.2014.08.009
62. Samrat SK, Tharappel AM, Li Z, Li H. Prospect of SARS-CoV-2 Spike Protein: Potential Role in Vaccine and Therapeutic Development. Virus Res (2020) 288:198141. doi: 10.1016/j.virusres.2020.198141
63. Li F. Structure, Function, and Evolution of Coronavirus Spike Proteins. Annu Rev Virol (2016) 3:237–61. doi: 10.1146/annurev-virology-110615-042301
64. Luo CM, Wang N, Yang XL, Liu HZ, Zhang W, Li B, et al. Discovery of Novel Bat Coronaviruses in South China That Use the Same Receptor as Middle East Respiratory Syndrome Coronavirus. J Virol (2018) 92:e00116-18. doi: 10.1128/JVI.00116-18
65. Wan Y, Shang J, Graham R, Baric RS, Li F. Receptor Recognition by the Novel Coronavirus From Wuhan: An Analysis Based on Decade-Long Structural Studies of SARS Coronavirus. J Virol (2020) 94:e00127-20. doi: 10.1128/JVI.00127-20
66. Jafary F, Jafari S, Ganjalikhany MR. In Silico Investigation of Critical Binding Pattern in SARS-CoV-2 Spike Protein With Angiotensin-Converting Enzyme 2. Sci Rep (2021) 11:6927. doi: 10.1038/s41598-021-86380-2
67. Naqvi A, Fatima K, Mohammad T, Fatima U, Singh IK, Singh A, et al. Insights Into SARS-CoV-2 Genome, Structure, Evolution, Pathogenesis and Therapies: Structural Genomics Approach. Biochim Biophys Acta Mol Basis Dis (2020) 1866:165878. doi: 10.1016/j.bbadis.2020.165878
68. Jia HP, Look DC, Shi L, Hickey M, Pewe L, Netland J, et al. ACE2 Receptor Expression and Severe Acute Respiratory Syndrome Coronavirus Infection Depend on Differentiation of Human Airway Epithelia. J Virol (2005) 79:14614–21. doi: 10.1128/JVI.79.23.14614-14621.2005
69. Jiang F, Yang J, Zhang Y, Dong M, Wang S, Zhang Q, et al. Angiotensin-Converting Enzyme 2 and Angiotensin 1-7: Novel Therapeutic Targets. Nat Rev Cardiol (2014) 11:413–26. doi: 10.1038/nrcardio.2014.59
70. Santos RA, Ferreira AJ, Verano-Braga T, Bader M. Angiotensin-Converting Enzyme 2, Angiotensin-(1-7) and Mas: New Players of the Renin-Angiotensin System. J Endocrinol (2013) 216:R1–R17. doi: 10.1530/JOE-12-0341
71. Kuba K, Imai Y, Rao S, Gao H, Guo F, Guan B, et al. A Crucial Role of Angiotensin Converting Enzyme 2 (ACE2) in SARS Coronavirus-Induced Lung Injury. Nat Med (2005) 11:875–9. doi: 10.1038/nm1267
72. Imai Y, Kuba K, Rao S, Huan Y, Guo F, Guan B, et al. Angiotensin-Converting Enzyme 2 Protects From Severe Acute Lung Failure. Nature (2005) 436:112–6. doi: 10.1038/nature03712
73. Ortega JT, Serrano ML, Pujol FH, Rangel HR. Role of Changes in SARS-CoV-2 Spike Protein in the Interaction With the Human ACE2 Receptor: An in Silico Analysis. EXCLI J (2020) 19:410–7. doi: 10.17179.excli2020-1167
74. Salata C, Calistri A, Parolin C, Palu G. Coronaviruses: A Paradigm of New Emerging Zoonotic Diseases. Pathog Dis (2019) 77:ftaa006. doi: 10.1093/femspd/ftaa006
75. Woo PC, Lau SK, Wernery U, Wong EY, Tsang AK, Johnson B, et al. Novel Betacoronavirus in Dromedaries of the Middle Eas. Emerg Infect Dis (2014) 20:560–72. doi: 10.3201/eid2004.131769
76. Cuan-Baltazar JY, Munoz-Perez MJ, Robledo-Vega C, Perez-Zepeda MF, Soto-Vega E. Misinformation of Covid-19 on the Internet:Infodemiology Study. JMIR Public Health Surveill (2020) 6:e18444. doi: 10.2196/18444
77. Lam TT, Jia N, Zhang YW, Shum MH, Jiang JF, Zhu HC, et al. Identifying SARS-CoV-2-Related Coronaviruses in Malayan Pangolins. Nature (2020) 583:282–5. doi: 10.1038/s41586-020-2169-0
78. Jones KE, Patel NG, Levy MA, Storeygard A, Balk D, Gittleman JL, et al. Global Trends in Emerging Infectious Diseases. Nature (2008) 451:990–3. doi: 10.1038/nature06536
79. Li H, Mendelsohn E, Zong C, Zhang W, Hagan E, Wang N, et al. Human-Animal Interactions and Bat Coronavirus Spillover Potential Among Rural Residents in Southern China. Biosaf Health (2019) 1:84–90. doi: 10.1016/j.bsheal.2019.10.004
80. Bell D, Robertson S, Hunter PR. Animal Origins of SARS Coronavirus:Possible Links With the International Trade in Small Carnivores. Philos Trans R Soc Lond B Biol Sci (2004) 359:1107–14. doi: 10.1098/rstb.2004.1492
81. Krahling V, Dolnik O, Kolesnikova L, Schmidt-Chanasit J, Jordan I, Sandig V, et al. Establishment of Fruit Bat Cells (Rousettus Aegyptiacus) as a Model System for the Investigation of Filoviral Infection. PloS Negl Trop Dis (2010) 4:e802. doi: 10.1371/journal.pntd.0000802
82. Liu P, Jiang J-Z, Wan X-F, Hua Y, Wang X, Hou F, et al. Are Pangolins the Intermediate Host of the 2019 Novel Coronavirus (SARS-CoV-2)? PloS Pathog (2020) 16:e1008421. doi: 10.1371/journal.ppat.1008421
83. Wang LF, Eaton BT. “Bats, Civets and the Ermergence of SARS,”. In: Childs JE, Mackenzie JS, Richt JA, editors. Wildlife and Emerging Zoonotic Diseases: The Biology, Circumstances and Consequences of Cross-Species Transmission. Berlin, Heidelberg: Springer-Verlag (2007).
84. Valentine MJ, Murdock CC, Kelly PJ. Sylvatic Cycles of Arboviruses in non-Human Primates. Parasit Vectors (2019) 12:463. doi: 10.1186/s13071-019-3732-0
85. Wang LF, Anderson DE. Viruses in Bats and Potential Spillover to Animals and Humans. Curr Opin Virol (2019) 34:79–89. doi: 10.1016/j.coviro.2018.12.007
86. Zinsstag J, Crump L, Schelling E, Hattendorf J, Maidane YO, Ali KO, et al. Climate Change and One Health. FEMS Microbiol Lett (2018) 365:fny085. doi: 10.1093/femsle/fny085
87. Drexler JF, Corman VM, Drosten C. Ecology, Evolution and Classification of Bat Coronaviruses in the Aftermath of SARS. Antiviral Res (2014) 101:45–56. doi: 10.1016/j.antiviral.2013.10.013
88. Jo WK, De Oliveira-Filho EF, Rasche A, Greenwood AD, Osterrieder K, Drexler JF. Potential Zoonotic Sources of SARS-CoV-2 Infections. Transbound Emerg Dis (2021) 68:1824–34. doi: 10.1111/tbed.13872
89. Li W, Shi Z, Yu M, Ren W, Smith C, Epstein JH, et al. Bats are Natural Reservoirs of SARS-Like Coronaviruses. Science (2005) 310:676–9. doi: 10.1126/science.1118391
90. Drexler JF, Gloza-Rausch F, Glende J, Corman VM, Muth D, Goettsche M, et al. Genomic Characterization of Severe Acute Respiratory Syndrome-Related Coronavirus in European Bats and Classification of Coronaviruses Based on Partial RNA-Dependent RNA Polymerase Gene Sequences. J Virol (2010) 84:11336–49. doi: 10.1128/JVI.00650-10
91. Hu B, Zeng LP, Yang XL, Ge XY, Zhang W, Li B, et al. Discovery of a Rich Gene Pool of Bat SARS-Related Coronaviruses Provides New Insights Into the Origin of SARS Coronavirus. PloS Pathog (2017) 13:e1006698. doi: 10.1371/journal.ppat.1006698
92. Chan JF, To KK, Tse H, Jin DY, Yuen KY. Interspecies Transmission and Emergence of Novel Viruses: Lessons From Bats and Birds. Trends Microbiol (2013) 21:544–55. doi: 10.1016/j.tim.2013.05.005
93. Banerjee A, Kulcsar K, Misra V, Frieman M, Mossman K. Bats and Coronaviruses. Viruses (2019) 11:41. doi: 10.3390/v11010041
94. Lu D, Liu K, Zhang D, Yue C, Lu Q, Cheng H, et al. Peptide Presentation by Bat MHC Class I Provides New Insight Into the Antiviral Immunity of Bats. PloS Biol (2019) 17:e3000436. doi: 10.1371/journal.pbio.3000436
95. Subudhi S, Rapin N, Misra V. Immune System Modulation and Viral Persistence in Bats: Understanding Viral Spillover. Viruses (2019) 11:192. doi: 10.3390/v11020192
96. Dijkman R, Jebbink MF, Gaunt E, Rossen JW, Templeton KE, Kuijpers TW, et al. The Dominance of Human Coronavirus OC43 and NL63 Infections in Infants. J Clin Virol (2012) 53:135–9. doi: 10.1016/j.jcv.2011.11.011
97. St-Jean JR, Jacomy H, Desforges M, Vabret A, Freymuth F, Talbot PJ. Human Respiratory Coronavirus OC43: Genetic Stability and Neuroinvasion. J Virol (2004) 78:8824–34. doi: 10.1128/JVI.78.16.8824-8834.2004
98. Morfopoulou S, Brown JR, Davies EG, Anderson G, Virasami A, Qasim W, et al. Human Coronavirus OC43 Associated With Fatal Encephalitis. N Engl J Med (2016) 375:497–8. doi: 10.1056/NEJMc1509458
99. Nilsson A, Edner N, Albert J, Ternhag A. Fatal Encephalitis Associated With Coronavirus OC43 in an Immunocompromised Child. Infect Dis (Lond) (2020) 52:419–22. doi: 10.1080/23744235.2020.1729403
100. Lau SK, Woo PC, Li KS, Tsang AK, Fan RY, Luk HK, et al. Discovery of a Novel Coronavirus, China Rattus Coronavirus HKU24, From Norway Rats Supports the Murine Origin of Betacoronavirus 1 and has Implications for the Ancestor of. J Virol (2015) 89:3076–92. doi: 10.1128/JVI.02420-14
101. Ge XY, Yang WH, Zhou JH, Li B, Zhang W, Shi ZL, et al. Detection of Alpha- and Betacoronaviruses in Rodents From Yunnan, China. Virol J (2017) 14:98. doi: 10.1186/s12985-017-0766-9
102. Cui J, Li F, Shi ZL. Origin and Evolution of Pathogenic Coronaviruses. Nat Rev Microbiol (2019) 17:181–92. doi: 10.1038/s41579-018-0118-9
103. Boni MF, Lemey P, Jiang X, Lam TT-Y, Perry B, Castoe T, et al. Evolutionary Origins of the SARS-CoV-2 Sarbecovirus Lineage Responsible for the COVID-19 Pandemic. Nat Microbiol (2020) 5:1408–17. doi: 10.1038/s41564-020-0771-4
104. Luk HKH, Li X, Fung J, Lau SKP, Woo PCY. Molecular Epidemiology, Evolution and Phylogeny of SARS Coronavirus. Infect Genet Evol (2019) 71:21–30. doi: 10.1016/j.meegid.2019.03.001
105. Paakkari L, Okan O. COVID-19: Health Literacy is an Underestimated Problem. Lancet Public Health (2020) 5:e249–250. doi: 10.1016/S2468-2667(20)30086-4
106. Hu B, Ge X, Wang LF, Shi Z. Bat Origin of Human Coronaviruses. Virol J (2015) 12:221. doi: 10.1186/s12985-015-0422-1
107. Ge XY, Li JL, Yang XL, Chmura AA, Zhu G, Epstein JH, et al. Isolation and Characterization of a Bat SARS-Like Coronavirus That Uses the ACE2 Receptor. Nature (2013) 503:535–8. doi: 10.1038/nature12711
108. Lau SK, Woo PC, Li KS, Huang Y, Tsoi HW, Wong BH, et al. Severe Acute Respiratory Syndrome Coronavirus-Like Virus in Chinese Horseshoe Bats. Proc Natl Acad Sci U.S.A. (2005) 102:14040–5. doi: 10.1073/pnas.0506735102
110. Banerjee A, Doxey AC, Mossman K, Irving AT. Unraveling the Zoonotic Origin and Transmission of SARS-CoV-2. Trends Ecol Evol (2021) 36:180–4. doi: 10.1016/j.tree.2020.12.002
111. Perez JC, Montagnier L. Covid-19, Sars and Bats Coronaviruses Genomes Peculiar Homologous Rna Sequences. Int J Res GRANTHAALAYAH (2020) 8:217–63. doi: 10.29121/granthaalayah.v8.i7.2020.678
112. Sallard E, Halloy J, Casane D, Van Helden J, Decroly É. Retrouver Les Origines Du SARS-CoV-2 Dans Les Phylogénies De Coronavirus [Tracing the Origins of SARS-COV-2 in Coronavirus Phylogenies]. Med Sci (2020) 36:783–96. doi: 10.1051/medsci/2020123
113. Xiao C, Li X, Liu S, Sang Y, Gao SJ, Gao F. HIV-1 did Not Contribute to the 2019-Ncov Genome. Emerg Microbes Infect (2020) 9:378–81. doi: 10.1080/22221751.2020.1727299
114. Becker MM, Graham RL, Donaldson EF, Rockx B, Sims AC, Sheahan T, et al. Synthetic Recombinant Bat SARS-Like Coronavirus is Infectious in Cultured Cells and in Mice. Proc Natl Acad Sci U.S.A. (2008) 105:19944–9. doi: 10.1073/pnas.0808116105
115. Menachery VD, Dinnon KH 3rd, Yount BL Jr., Mcanarney ET, Gralinski LE, Hale A, et al. Trypsin Treatment Unlocks Barrier for Zoonotic Bat Coronavirus Infection. J Virol (2020) 94:e01774-19. doi: 10.1128/JVI.01774-19
116. Huang IC, Bosch BJ, Li F, Li W, Lee KH, Ghiran S, et al. SARS Coronavirus, But Not Human Coronavirus NL63, Utilizes Cathepsin L to Infect ACE2-Expressing Cells. J Biol Chem (2006) 281:3198–203. doi: 10.1074/jbc.M508381200
117. Yamada Y, Liu DX. Proteolytic Activation of the Spike Protein at a Novel RRRR/S Motif is Implicated in Furin-Dependent Entry, Syncytium Formation, and Infectivity of Coronavirus Infectious Bronchitis Virus in Cultured Cells. J Virol (2009) 83:8744–58. doi: 10.1128/JVI.00613-09
118. Coutard B, Valle C, De Lamballerie X, Canard B, Seidah NG, Decroly E. The Spike Glycoprotein of the New Coronavirus 2019-Ncov Contains a Furin-Like Cleavage Site Absent in CoV of the Same Clade. Antiviral Res (2020) 176:104742. doi: 10.1016/j.antiviral.2020.104742
119. Hao P, Zhong W, Song S, Fan S, Li X. Is SARS-CoV-2 Originated From Laboratory? A Rebuttal to the Claim of Formation via Laboratory Recombination. Emerg Microbes Infect (2020) 9:545–7. doi: 10.1080/22221751.2020.1738279
120. Liu SL, Saif LJ, Weiss SR, Su L. No Credible Evidence Supporting Claims of the Laboratory Engineering of SARS-CoV-2. Emerg Microbes Infect (2020) 9:505–7. doi: 10.1080/22221751.2020.1733440
121. Othman H, Bouslama Z, Brandenburg JT, Da Rocha J, Hamdi Y, Ghedira K, et al. Interaction of the Spike Protein RBD From SARS-CoV-2 With ACE2: Similarity With SARS-CoV, Hot-Spot Analysis and Effect of the Receptor Polymorphism. Biochem Biophys Res Commun (2020) 527:702–8. doi: 10.1016/j.bbrc.2020.05.028
122. Cohen J. Wuhan Coronavirus Hunter Shi Zhengli Speaks Out. Science (2020) 369:487–8. doi: 10.1126/science.369.6503.487
123. Frutos R, Gavotte L, Devaux CA. Understanding the Origin of COVID-19 Requires to Change the Paradigm on Zoonotic Emergence From the Spillover to the Circulation Model. Infect Genet Evol (2021) 95:104812. doi: 10.1016/j.meegid.2021.104812
124. Pekar JE, Magee A, Parker E, Moshiri N, Izhikevich K, Havens JL, et al. SARS-CoV-2 Emergence Very Likely Resulted From at Least Two Zoonotic Events. Zenodo (2022) 1–82. doi: 10.5281/zenodo.6291628
125. Wu Z, Jin Q, Wu G, Lu J, Li M, Guo D, et al. SARS-CoV-2’s Origin Should be Investigated Worldwide for Pandemic Prevention. Lancet (2021) 398:1299–303. doi: 10.1016/S0140-6736(21)02020-1
126. Lai A, Bergna A, Acciarri C, Galli M, Zehender G. Early Phylogenetic Estimate of the Effective Reproduction Number of SARS-CoV-2. J Med Virol (2020) 92:675–9. doi: 10.1002/jmv.25723
127. Matyasek R, Kovarik A. Mutation Patterns of Human SARS-CoV-2 and Bat RaTG13 Coronavirus Genomes Are Strongly Biased Towards C>U Transitions, Indicating Rapid Evolution in Their Hosts. Genes (Basel) (2020) 11:761. doi: 10.3390/genes11070761
128. Zehender G, Lai A, Bergna A, Meroni L, Riva A, Balotta C, et al. Genomic Characterization and Phylogenetic Analysis of SARS-COV-2 in Italy. J Med Virol (2020) 92:1637–40. doi: 10.1002/jmv.25794
129. Zhou H, Chen X, Hu T, Li J, Song H, Liu Y, et al. A Novel Bat Coronavirus Closely Related to SARS-CoV-2 Contains Natural Insertions at the S1/S2 Cleavage Site of the Spike Protein. Curr Biol (2020) 30:2196–2203.e2193. doi: 10.1016/jcub.2020.05.023
130. Zhou P, Yang XL, Wang XG, Hu B, Zhang L, Zhang W, et al. A Pneumonia Outbreak Associated With a New Coronavirus of Probable Bat Origin. Nature (2020) 579:270–3. doi: 10.1038/s41586-020-2012-7
131. Zhang T, Wu Q, Zhang Z. Probable Pangolin Origin of SARS-CoV-2 Associated With the COVID-19 Outbreak. Curr Biol (2020) 30:1346–1351.e2. doi: 10.1016/j.cub.2020.03.022
132. Li X, Zai J, Zhao Q, Nie Q, Li Y, Foley BT, et al. Evolutionary History, Potential Intermediate Animal Host, and Cross-Species Analyses of SARS-CoV-2. J Med Virol (2020) 92:602–11. doi: 10.1002/jmv.25731
133. Hassanin A, Grandcolas P, Veron G. Covid-19: Natural or Anthropic Origin? Mammalia (2021) 85:1–7. doi: 10.1515/mammalia-2020-0044
134. Lytras S, Hughes J, Martin D, De Klerk A, Lourens R, Kosakovsky Pond SL, et al. “Exploring the Natural Origins of SARS-CoV-2 in the Light of Recombination”. (bioRxiv) (2021) 14:1–14. doi: 10.1101/2021.01.22.427830
135. Wang H, Pipes L, Nielsen R. Synonymous Mutations and the Molecular Evolution of SARS-CoV-2 Origins. Virus Evol (2020) 7:veaa098. doi: 10.1101/2020.04.20.052019
136. Lytras S, Hughes J, Martin D, Swanepoel P, De Klerk A, Lourens R, et al. Exploring the Natural Origins of SARS-CoV-2 in the Light of Recombination. Genome Biol Evol (2022) 14:evac018. doi: 10.1093/gbe/evac018
137. Takata MA, Goncalves-Carneiro D, Zang TM, Soll SJ, York A, Blanco-Melo D, et al. CG Dinucleotide Suppression Enables Antiviral Defence Targeting non-Self RNA. Nature (2017) 550:124–7. doi: 10.1038/nature24039
138. Xia S, Lan Q, Su S, Wang X, Xu W, Liu Z, et al. The Role of Furin Cleavage Site in SARS-CoV-2 Spike Protein-Mediated Membrane Fusion in the Presence or Absence of Trypsin. Signal Transduct Target Ther (2020) 5:92. doi: 10.1038/s41392-020-0184-0
139. Hossain MG, Javed A, Akter S, Saha S. SARS-CoV-2 Host Diversity: An Update of Natural Infections and Experimental Evidence. J Microbiol Immunol Infect (2021) 54:175–81. doi: 10.1016/j.jmii.2020.06.006
140. Benvenuto D, Giovanetti M, Ciccozzi A, Spoto S, Angeletti S, Ciccozzi M. The 2019-New Coronavirus Epidemic: Evidence for Virus Evolution. J Med Virol (2020) 92:455–9. doi: 10.1002/jmv.25688
141. Munnink BBO, Sikkema R, Nieuwenhuijse DF, Molenaar RJ, Munger E, Molenkamp R, et al. Transmission of SARS-CoV-2 on Mink Farms Between Humans and Mink and Back to Humans. Science (2021) 371:172–7. doi: 10.1126/science.abe5901
142. Al-Tawfiq JA, Memish ZA. Middle East Respiratory Syndrome Coronavirus: Epidemiology and Disease Control Measures. Infect Drug Resist (2014) 7:281–7. doi: 10.2147/IDR.S51283
143. Memish ZA, Mishra N, Olival KJ, Fagbo SF, Kapoor V, Epstein JH, et al. Middle East Respiratory Syndrome Coronavirus in Bats, Saudi Arabia. Emerg Infect Dis (2013) 19:1819–23. doi: 10.3201/eid1911.131172
144. Agnihothram S, Yount BL Jr., Donaldson EF, Huynh J, Menachery VD, Gralinski LE, et al. A Mouse Model for Betacoronavirus Subgroup 2c Using a Bat Coronavirus Strain HKU5 Variant. mBio (2014) 5:e00047–00014. doi: 10.1128/mBio.00047-14
145. Moreno A, Lelli D, De Sabato L, Zaccaria G, Boni A, Sozzi E, et al. Detection and Full Genome Characterization of Two Beta CoV Viruses Related to Middle East Respiratory Syndrome From Bats in Italy. Virol J (2017) 14:239. doi: 10.1186/s12985-017-0907-1
146. Royce K, Fu F. Mathematically Modeling Spillovers of an Emerging Infectious Zoonosis With an Intermediate Host. PloS One (2020) 15:e0237780. doi: 10.1371/journal.pone.0237780
147. Li X, Luk HK, Lau SKP, Woo PC. Human Coronaviruses: General Features. Ref Collection Biomed Sci (2019) 1-6. doi: 10.1016/B978-0-12-801238-3.95704-0
148. Vijgen L, Keyaerts E, Moes E, Thoelen I, Wollants E, Lermey P, et al. Molecular Clock Analysis Suggets a Relatively Recent Zoonotic Coronavirus Transmission Event. J Virol (2005) 79:1595–604. doi: 10.1128/JVI.79.3.1595-1604.2005
149. Reusken CBEM, Haagmans BL, Muller MA, Gutierrez C, Godeke G-J, Meyer B, et al. Middle East Respiratory Syndrome Coronavirus Neutralising Serum Antibodies in Dromedary Camels: A Comparative Serological Study. Lancet Infect Dis (2013) 13:859–66. doi: 10.1016/S1473-3099(13)70164-6
150. Mciver DJ, Silithammavong S, Theppangna W, Gillis A, Douangngeun B, Khammavong K, et al. Coronavirus Surveillance of Wildlife in the Lao People’s Democratic Republic Detects Viral RNA in Rodents. Arch Virol (2020) 165:1869–75. doi: 10.1007/s00705-020-04683-7
151. Guan Y, Zheng BJ, He YQ, Liu ZL, Zhuang ZX, Cheung CL, et al. Isolation and Characterization of Viruses Related to SARS-CoV From Animals in Southern China. Science (2003) 302:276–8. doi: 10.1126/science.1087139
152. Martina BEE, Haagmans BL, Kuiken T, Fouchier R, Rimmelzwaan GF, Van Amerongen G, et al. SARS Virus Infection of Cats and Ferrets. Nature (2003) 425:915. doi: 10.1038/425915a
153. Li L, Wang X, Hua Y, Liu P, Zhou J, Chen J, et al. Epidemiological Study of Betacoronaviruses in Captive Malayan Pangolins. Front Microbiol (2021) 12:657439. doi: 10.3389/fmicb.2021.657439
154. Palmer MV, Martins M, Falkenberg S, Buckley A, Caserta LC, Mitchell PK, et al. Susceptibility of White-Tailed Deer (Odocoileus Virginianus) to SARS-CoV-2. J Virol (2021) 95:1–16. doi: 10.1101/2021.01.13.426628
155. Gaudreault NN, Trujillo JD, Carossino M, Meekins DA, Morozov I, Madden DW, et al. SARS-CoV-2 Infection, Disease and Transmission in Domestic Cats. Emerg Microbes Infect (2020) 9:2322–32. doi: 10.1080/22221751.2020.1833687
156. Sreenivasan CC, Thomas M, Wang D, Li F. Susceptibility of Livestock and Companion Animals to COVID-19. J Med Virol (2021) 93(3):1351–60. doi: 10.1002/jmv.26621
157. Mykytyn AZ, Lamers MM, Okba NMA, Breugem TI, Schipper D, van den Doel PB, et al. Susceptibility of rabbits to SARS-CoV-2. Emerg Microbes Infect (2021) 10(1):1-7. doi:10.1080/22221751.2020.1868951
158. Ulrich L, Wernike K, Hoffmann D, Mettenleiter TC, Beer M. Experimental Infection of Cattle with SARS-CoV-2. Emerg Infect Dis (2020) 26(12):2979–81. doi: 10.3201/eid2612.203799
159. Sia SF, Yan LM, Chin AWH, Fung K, Choy KT, Wong AYL, et al. Pathogenesis and Transmission of SARS-CoV-2 in Golden Hamsters. Nature (2020) 583:834–8. doi: 10.1038/s41586-020-2342-5
160. Bosco-Lauth AM, Root JJ, Porter SM, Walker AE, Guilbert L, Hawvermale D, et al. Survey of Peridomestic Mammal Susceptibility to SARS-CoV-2 Infection. bioRxiv (2021) 27:2073–2080. doi: 10.3201/eid2708.210180
161. Bao L, Deng W, Huang B, Gao H, Liu J, Ren L, et al. The Pathogenicity of SARS-CoV-2 in Hace2 Transgenic Mice. Nature (2020) 583:830–3. doi: 10.1038/s41586-020-2312-y
162. Freuling CM, Breithaupt A, Muller T, Sehl J, Balkema-Buschmann A, Rissmann M, et al. Susceptibility of Raccoon Dogs for Experimental SARS-CoV-2 Infection. Emerg Infect Dis (2020) 26:2982–5. doi: 10.3201/eid2612.203733
163. Damas J, Hughes GM, Keough KC, Painter CA, Persky NS, Corbo M, et al. Broad Host Range of SARS-CoV-2 Predicted by Comparative and Structural Analysis of ACE2 in Vertebrates. bioRxiv (2020) 117:2311–22322. doi: 10.1073/pnas.2010146117
164. Ulrich L, Wernike K, Hoffmann D, Mettenleiter TC, Beer M. Experimental Infection of Cattle With SARS-CoV-2. Emerg Infect Dis (2020) 26:2979–81. doi: 10.3201/eid2612.203799
165. Boklund A, Gortazar C, Pasquali P, Roberts H, Nielsen SS, Stahl K, et al. Monitoring of SARS-CoV-2 Infection in Mustelids. EFSA J (2021) 19(3):e06459. doi: 10.2903/j.efsa.2021.6459
166. Shi J, Wen Z, Zhong G, Yang H, Wang C, Huang B, et al. Susceptibility of Ferrets, Cats, Dogs, and Other Domesticated Animals to SARS-Coronavirus 2. Science (2020) 368:1016–20. doi: 10.1126/science.abb7015
167. Adney DR, Bielefeldt-Ohmann H, Hartwig AE, Bowen RA. Infection, Replication, and Transmission of Middle East Respiratory Syndrome Coronavirus in Alpacas. Emerg Infect Dis (2016) 22:1031–7. doi: 10.3201/eid2206.160192
168. Erku DA, Belachew SA, Abraha S, Sinnollareddy M, Thomas J, Steadman KJ, et al. When Fear and Misinformation Go Viral: Pharmacists’ Role in Deterring Medication Misinformation During the ‘Infodemic’ Surrounding COVID-19. Res Soc Admin Pharm (2021) 17:1954–63. doi: 10.1016/j.sapharm.2020.04.032
170. Vijgen L, Keyaerts E, Lemey P, Maes P, Van Reeth K, Nauwynck H, et al. Evolutionary History of the Closely Related Group 2 Coronaviruses: Porcine Hemagglutinating Encephalomyelitis Virus, Bovine Coronavirus, and Human Coronavirus OC43. J Virol (2006) 80:7270–4. doi: 10.1128/JVI.02675-05
171. Pearlman S, Netland J. Coronaviruses Post-SARS: Update on Replication and Pathogenesis. Nat Rev Microbiol (2009) 7:439–50. doi: 10.1038/nrmicro2147
172. Paraskevis D, Kostaki EG, Magiorkinis G, Panayiotakopoulos G, Sourvinos G, Tsiodras S. Full-Genome Evolutionary Analysis of the Novel Corona Virus (2019-Ncov) Rejects the Hypothesis of Emergence as a Result of a Recent Recombination Event. Infect Genet Evol (2020) 79:104212. doi: 10.1016/j.meegid.2020.104212
173. Woo PCY, Lau SKP, Yuen K. Infectious Diseases Emerging From Chinese Wet-Markets: Zoonotic Origins of Severe Respiratory Viral Infections. Curr Opin Infect Dis (2006) 19:401–7. doi: 10.1097/01.qco.0000244043.08264.fc
174. Wrobel AG, Benton DJ, Xu P, Roustan C, Martin SR, Rosenthal PB, et al. SARS-CoV-2 and Bat RaTG13 Spike Glycoprotein Structures Inform on Virus Evolution and Furin-Cleavage Effects. Nat Struct Mol Biol (2020) 27:763–7. doi: 10.1038/s41594-020-0468-7
175. Song HD, Tu CC, Zhang GW, Wang SY, Zheng K, Lei LC, et al. Cross-Host Evolution of Severe Acute Respiratory Syndrome Coronavirus in Palm Civet and Human. Proc Natl Acad Sci U.S.A. (2005) 102:2430–5. doi: 10.1073/pnas.0409608102
176. Huang C, Wang Y, Li X, Ren L, Zhao J, Hu Y, et al. Clinical Features of Patients Infected With 2019 Novel Coronavirus in Wuhan, China. Lancet (2020) 395:497–506. doi: 10.1016/S0140-6736(20)30183-5
177. Xu RH, He JF, Evans MR, Peng GW, Field HE, Yu DW, et al. Epidemiologic Clues to SARS Origin in China. Emerg Infect Dis (2004) 10:1031–7. doi: 10.3201/eid1006.030852
178. Li X, Giorgi EE, Marichannegowda MH, Foley B, Xiao C, Kong XP, et al. Emergence of SARS-CoV-2 Through Recombination and Strong Purifying Selection. Sci Adv (2020) 6:eabb9153. doi: 10.1126/sciadv.abb9153
179. Mcaloose D, Laverack M, Wang L, Killian ML, Caserta LC, Yuan F, et al. From People to Panthera: Natural SARS-CoV-2 Infection in Tigers and Lions at the Bronx Zoo. mBio (2020) 11:e02220-20. doi: 10.1128/mBio.02220-20
180. Bartlett SL, Diel DG, Wang L, Zec S, Laverack M, Martins M, et al. Sars-Cov-2 Infection and Longitudinal Fecal Screening in Malayan Tigers (Panthera Tigris Jacksoni), Amur Tigers (Panthera Tigris Altaica), and African Lions (Panthera Leo Krugeri) at the Bronx Zoo, New York, USA. J Zoo Wildl Med (2021) 51:733–44. doi: 10.1638/2020-0171
181. Enkirch T, Von Messling V. Ferret Models of Viral Pathogenesis. Virology (2015) 479-480:259–70. doi: 10.1016/j.virol.2015.03.017
182. Blanco-Melo D, Nilsson-Payant BE, Liu WC, Uhl S, Hoagland D, Moller R, et al. Imbalanced Host Response to SARS-CoV-2 Drives Development of COVID-19. Cell (2020) 181:1036–1045 e1039. doi: 10.1016/j.cell.2020.04.026
183. Kim YI, Kim SG, Kim SM, Kim EH, Park SJ, Yu KM, et al. Infection and Rapid Transmission of SARS-CoV-2 in Ferrets. Cell Host Microbe (2020) 27:704–709.e702. doi: 10.1016/j.chom.2020.03.023
184. Schlottau K, Rissmann M, Graaf A, Schön J, Sehl J, Wylezich C, et al. SARS-CoV-2 in Fruit Bats, Ferrets, Pigs, and Chickens: An Experimental Transmission Study. Lancet Microbe (2020) 1:e218–25. doi: 10.1016/S2666-5247(20)30089-6
185. Ryan KA, Bewley KR, Fotheringham SA, Slack GS, Brown P, Hall Y, et al. Dose-Dependent Response to Infection With SARS-CoV-2 in the Ferret Model and Evidence of Protective Immunity. Nat Commun (2021) 12:81. doi: 10.1038/s41467-020-20439-y
186. Oreshkova N, Molenaar RJ, Vreman S, Harders F, Oude Munnink BB, Hakze-Van Der Honing RW, et al. SARS-CoV-2 Infection in Farmed Minks, the Netherlands, April and May 2020. Euro Surveill (2020) 25:2001005. doi: 10.2807/1560-7917.ES.2020.25.23.2001005
187. Hammer AS, Quaade ML, Rasmussen TB, Fonager J, Rasmussen M, Mundbjerg K, et al. SARS-CoV-2 Transmission Between Mink (Neovison Vison) and Humans, Denmark. Emerg Infect Dis (2021) 27:547–51. doi: 10.3201/eid2702.203794
188. Abdel-Moneim AS, Abdelwhab EM. Evidence for SARS-CoV-2 Infection of Animal Hosts. Pathogens (2020) 9:529. doi: 10.3390/pathogens9070529
189. Mallapaty S. The Hunt for Coronavirus Carriers. Nature (2021) 591:26–8. doi: 10.1038/d41586-021-00531-z
190. World Health Organization, Food and Agriculture Organization of the United Nations, World Organisation for Animal Health. SARS-CoV-2 in Animals Used for Fur Farming: GLEWS+ Risk Assessment, 20 January 2021. Rome: World Health Organization (2021). Available at: https://apps.who.int/iris/handle/10665/339626.
191. Díaz AV, Walker M, Webster JP. Surveillance and Control of SARS-CoV-2 in Mustelids: An Evolutionary Perspective. Evol Appl (2021) 14:2715–2725. doi: 10.1111/eva.13310
192. Gautam A, Kaphle K, Shrestha B, Phuyal S. Susceptibility to SARS, MERS, and COVID-19 From Animal Health Perspective. Open Vet J (2020) 10:164–77. doi: 10.4314/ovj.v10i2.6
193. Xu L, Zhang Y, Liu Y, Chen Z, Deng H, Ma Z, et al. Angiotensin-Converting Enzyme 2 (ACE2) From Raccoon Dog can Serve as an Efficient Receptor for the Spike Protein of Severe Acute Respiratory Syndrome Coronavirus. J Gen Virol (2009) 90:2695–703. doi: 10.1099/vir.0.013490-0
194. Zhai X, Sun J, Yan Z, Zhang J, Zhao J, Zhao Z, et al. Comparison of Severe Acute Respiratory Syndrome Coronavirus 2 Spike Protein Binding to ACE2 Receptors From Human, Pets, Farm Animals, and Putative Intermediate Hosts. J Virol (2020) 94:e00831-20. doi: 10.1128/JVI.00831-20
195. Luan J, Lu Y, Jin X, Zhang L. Spike Protein Recognition of Mammalian ACE2 Predicts the Host Range and an Optimized ACE2 for SARS-CoV-2 Infection. Biochem Biophys Res Commun (2020) 526:165–9. doi: 10.1016/j.bbrc.2020.03.047
196. Zhai X, Sun J, Yan Z, Zhang J, Zhao J, Zhao Z, et al. Comparison of Severe Acute Respiratory Syndrome Coronavirus 2 Spike Protein Binding to ACE2 Receptors From Human, Pets, Farm Animals, and Putative Intermediate Hosts. J Virol (2020) 94:e00831–00820. doi: 10.1128/JVI.00831-20
197. Griffin BD, Chan M, Tailor N, Mendoza EJ, Leung A, Warner BM, et al. SARS-CoV-2 Infection and Transmission in the North American Deer Mouse. Nat Commun (2021) 12:3612. doi: 10.1038/s41467-021-23848-9
198. Stone S, Rothan HA, Natekar JP, Kumari P, Sharma S, Pathak H, et al. SARS-CoV-2 Variants of Concern Infect the Respiratory Tract and Induce Inflammatory Response in Wild-Type Laboratory Mice. Viruses (2021) 14:27. doi: 10.3390/v14010027
199. Gao WH, Lin XD, Chen YM, Xie CG, Tan ZZ, Zhou JJ, et al. Newly Identified Viral Genomes in Pangolins With Fatal Disease. Virus Evol (2020) 6:veaa020. doi: 10.1093/ve/veaa020
200. Shang J, Wan Y, Luo C, Ye G, Geng Q, Auerbach A, et al. Cell Entry Mechanisms of SARS-CoV-2. Proc Natl Acad Sci U.S.A. (2020) 117:11727–34. doi: 10.1073/pnas.2003138117
201. Francis ME, Goncin U, Kroeker A, Swan C, Ralph R, Lu Y, et al. SARS-CoV-2 Infection in the Syrian Hamster Model Causes Inflammation as Well as Type I Interferon Dysregulation in Both Respiratory and non-Respiratory Tissues Including the Heart and Kidney. PloS Pathog (2021) 17:e1009705. doi: 10.1371/journal.ppat.1009705
202. Selvaraj P, Lien CZ, Liu S, Stauft CB, Nunez IA, Hernandez M, et al. SARS-CoV-2 Infection Induces Protective Immunity and Limits Transmission in Syrian Hamsters. Life Sci Alliance (2021) 4:e202000886. doi: 10.26508/lsa.202000886
203. Yen HL, Sit THC, Brackman CJ, Chuk SSY, Gu H, Tam KWS, et al. Transmission of SARS-CoV-2 (Variant Delta) From Pet Hamsters to Humans and Onward Human Propagation of the Adapted Strain: A Case Study. Lancet (2022) 399(10329):1070–78. doi: 10.1016/S0140-6736(22)00326-9
204. Liu Z, Xiao X, Wei X, Li J, Yang J, Tan H, et al. Composition and Divergence of Coronavirus Spike Proteins and Host ACE2 Receptors Predict Potential Intermediate Hosts of SARS-CoV-2. J Med Virol (2020) 92:595–601. doi: 10.1002/jmv.25726
205. Shen M, Liu C, Xu R, Ruan Z, Zhao S, Zhang H, et al. SARS-CoV-2 Infection of Cats and Dogs? Preprints (2020) 2020040116.
206. Sreenivasan CC, Thomas M, Wang D, Li F. Susceptibility of Livestock and Companion Animals to COVID-19. J Med Virol (2021) 93:1351–60. doi: 10.1002/jmv.26621
207. Halfmann P, Hatta M, Chiba S, Maemura T, Fan S. Transmission of SARS-CoV-2 in Domestic Cats. N Engl J Med (2020) 383:590–2. doi: 10.1056/NEJMc2013400
208. Li X. Cats Under the Shadow of the SARS-CoV-2 Pandemic. Transbound Emerg Dis (2020) 67:1416–7. doi: 10.1111/tbed.13599
209. Sit THC, Brackman CJ, Ip SM, Tam KWS, Law PYT, To EMW, et al. Infection of Dogs With SARS-CoV-2. Nature (2020) 586:776–8. doi: 10.1038/s41586-020-2334-5
210. Ellis J. What is the Evidence That Bovine Coronavirus is a Biologically Significant Respiratory Pathogen in Cattle? Can Vet J (2019) 60:147–52.
211. Suarez DL, Pantin-Jackwood MJ, Swayne DE, Lee SA, Deblois SM, Spackman E. Lack of Susceptibility to SARS-CoV-2 and MERS-CoV in Poultry. Emerg Infect Dis (2020) 26:3074–6. doi: 10.3201/eid2612.202989
212. Meekins DA, Morozov I, Trujillo JD, Gaudreault NN, Bold D, Carossino M, et al. Susceptibility of Swine Cells and Domestic Pigs to SARS-CoV-2. Emerg Microbes Infect (2020) 9:2278–88. doi: 10.1080/22221751.2020.1831405
213. Vergara-Alert J, Rodon J, Carrillo J, Te N, Izquierdo-Useros N, Rodriguez de la Concepcion ML, et al. Pigs are Not Susceptible to SARS-CoV-2 Infection But are a Model for Viral Immunogenicity Studies. Transbound Emerg Dis (2020) 68:1721–1725. doi: 10.1111/tbed.13861
214. Pickering BS, Smith G, Pinette MM, Embury-Hyatt C, Moffat E, Marszal P, et al. Susceptibility of Domestic Swine to Experimental Infection With Severe Acute Respiratory Syndrome Coronavirus 2. Emerg Infect Dis (2021) 27:104–12. doi: 10.3201/eid2701.203399
215. European Food Safety, A, European Centre for Disease, P., Control, Boklund A, Gortazar C, Pasquali P, Roberts H, et al. Monitoring of SARS-CoV-2 Infection in Mustelids. EFSA J (2021) 19(3):e06459. doi: 10.2903/j.efsa.2021.6459.
216. Lu S, Zhao Y, Yu W, Yang Y, Gao J, Wang J, et al. Comparison of SARS-CoV-2 Infections Among 3 Species of non-Human Primates. bioRxiv (2020) 031807. doi: 10.2139/ssrn.3578773
217. Rockx B, Kuiken T, Herfst S, Bestebroer T, Lamers MM, Oude Munnink BB, et al. Comparative Pathogenesis of COVID-19, MERS, and SARS in Nonhuman Primate Model. Science (2020) 368:1012–5. doi: 10.1126/science.abb7314
218. Lu S, Zhao Y, Yu W, Yang Y, Gao J, Wang J, et al. Comparison of Nonhuman Primates Identified the Suitable Model for COVID-19. Signal Transduct Target Ther (2020) 5:157. doi: 10.1038/s41392-020-00269-6
219. Patrono LV, Samuni L, Corman VM, Nourifar L, Rothemeier C, Wittig RM, et al. Human Coronavirus OC43 Outbreak in Wild Chimpanzees, Cote D Ivoir. Emerg Microbes Infect (2018) 7:118. doi: 10.1038/s41426-018-0121-2
220. Mykytyn AZ, Lamers MM, Okba NMA, Breugem TI, Schipper D, Van Den Doel PB, et al. Susceptibility of Rabbits to SARS-CoV-2. Emerg Microbes Infect (2021) 10:1–7. doi: 10.1080/22221751.2020.1868951
221. Munoz-Fontela C, Dowling WE, Funnell SGP, Gsell PS, Riveros-Balta AX, Albrecht RA, et al. Animal Models for COVID-19. Nature (2020) 586:509–15. doi: 10.1038/s41586-020-2787-6
222. Meekins DA, Gaudreault NN, Richt JA. Natural and Experimental SARS-CoV-2 Infection in Domestic and Wild Animals. Viruses (2021) 13:1993. doi: 10.3390/v13101993
223. Murphy HL, Ly H. Understanding the Prevalence of SARS-CoV-2 (COVD-19) Exposure in Companion, Captive, Wild, and Farmed Animals. Virulence (2021) 12:2777–2786. doi: 10.1080/21505594.2021.1996519
224. Lau SK, Poon RW, Wong BH, Wang M, Huang Y, Xu H, et al. Coexistence of Different Genotypes in the Same Bat and Serological Characterization of Rousettus Bat Coronavirus HKU9 Belonging to a Novel Betacoronavirus Subgroup. J Virol (2010) 84:11385–94. doi: 10.1128/JVI.01121-10
225. Cheng W, Xing S, Bonebrake TC. Recent Pangolin Seizures in China Reveal Priority Areas for Intervention. Conserv Lett (2017) 10:757–64. doi: 10.1111/conl.12339
226. Giovanetti M, Benedetti F, Campisi G, Ciccozzi A, Fabris S, Ceccarelli G, et al. Evolution Patterns of SARS-CoV-2: Snapshot on its Genome Variants. Biochem Biophys Res Commun (2021) 538:88–91. doi: 10.1016/j.bbrc.2020.10.102
227. Perera P, Karawita H. An Update of Distribution, Habitats and Conservation Status of the Indian Pangolin (Manis Crassicaudata) in Sri Lanka. Global Ecol Conserv (2020) 21:e00799. doi: 10.1016/j.gecco.2019.e00799
228. Xiao K, Zhai J, Feng Y, Zhou N, Zhang X, Zou J-J, et al. Isolation of SARS-CoV-2-Related Coronavirus From Malayan Pangolins. Nature (2020) 583:286–9. doi: 10.1038/s41586-020-2313-x
229. Tang X, Wu C, Li X, Song Y, Yao X, Wu X, et al. On the Origin and Continuing Evolution of SARS-CoV-2. Nat Sci Rev (2020) 7:1012–23. doi: 10.1093/nsr/nwaa036
230. Chen N, Li X, Li S, Xiao Y, Ye M, Yan X, et al. How Related is SARS-CoV-2 to Other Coronaviruses? Vet Rec (2020) 186:496. doi: 10.1136/vr.m1452
231. Ith S, Bumrungsri S, Furey NM, Bates PJ, Wonglapsuwan M, Khan F, et al. Taxonomic Implications of Geographical Variation in Rhinolophus Affinis (Chiroptera: Rhinolophidae) in Mainland Southeast Asia. Zool Stud (2015) 54:e31. doi: 10.1186/s40555-015-0109-8
232. Ge XY, Wang N, Zhang W, Hu B, Li B, Zhang YZ, et al. Coexistence of Multiple Coronaviruses in Several Bat Colonies in an Abandoned Mineshaft. Virol Sin (2016) 31:31–40. doi: 10.1007/s12250-016-3713-9
233. Lehmann D, Halbwax ML, Makaga L, Whytock R, Ndindiwe Malata LL, Bombenda Mouele W, et al. Pangolins and Bats Living Together in Underground Burrows in Lope National Park, Gabon. Afr J Ecol (2020) 1–3. doi: 10.1111/aje.12759
234. Lee J, Hughes T, Lee M-H, Field H, Rovie-Ryan JJ, Sitam FT, et al. No Evidence of Coronaviruses or Other Potentially Zoonotic Viruses in Sunda Pangolins (Manis Javanica) Entering the Wildlife Trade via Malaysia. Ecohealth (2020) 17:406–18. doi: 10.1007/s10393-020-01503-x
235. Liu P, Chen W, Chen JP. Viral Metagenomics Revealed Sendai Virus and Coronavirus Infection of Malayan Pangolins (Manis Javanica). Viruses (2019) 11:979. doi: 10.3390/v11110979
236. Han GZ. Pangolins Harbor SARS-CoV-2-Related Coronaviruses. Trends Microbiol (2020) 28:515–7. doi: 10.1016/j.tim.2020.04.001
237. Yuan S, Jiang SC, Li ZL. Analysis of Possible Intermediate Hosts of the New Coronavirus SARS-CoV-2. Front Vet Sci (2020) 7:379. doi: 10.3389/fvets.2020.00379
238. Haagmans BL, Al Dhahiry SHS, Reusken CBEM, Stalin R, Galiano M, Meyers R, et al. Middle East Respiratory Syndrome Coronavirus in Dromedary Camels: An Outbreak Investigation. Lancet Infect Dis (2014) 14:140–5. doi: 10.1016/S1473-3099(13)70690-X
239. Reusken CB, Schilp C, Raj VS, De Bruin E, Kohl RH, Farag EA, et al. MERS-CoV Infection of Alpaca in a Region Where MERS-CoV is Endemic. Emerg Infect Dis (2016) 22:1129–31. doi: 10.3201/eid2206.152113
240. Dudas G, Rambaut A. MERS-CoV Recombination: Implications About the Reservoir and Potential for Adaptation. Virus Evol (2016) 2:vev023. doi: 10.1093/ve/vev023
241. Cotten M, Watson SJ, Kellam P, Al-Rabeeah AA, Makhdoom HQ, Assiri A, et al. Transmission and Evolution of the Middle East Respirtory Syndrome Coronavirus in Saudi Arabia: A Descriptive Genomic Study. Lancet (2013) 382:1993–2002. doi: 10.1016/S0140-6736(13)61887-5
242. Dudas G, Carvalho LM, Rambaut A, Bedford T. MERS-CoV Spillover at the Camel-Human Interface. Elife (2018) 7:e31257. doi: 10.7554/eLife.31257
243. Corman VM, Ithete NL, Richards LR, Schoeman MC, Preiser W, Drosten C, et al. Rooting the Phylogenetic Tree of Middle East Respiratory Syndrome Coronavirus by Characterization of a Conspecific Virus From an African Bat. J Virol (2014) 88:11297–303. doi: 10.1128/JVI.01498-14
244. Lu G, Wang Q, Gao GF. Bat-To-Human: Spike Features Detrmining ‘Host Jump’ of Coronaviruses SARS-VoV, MERS-CoV, and Beyond. Trends Microbiol (2015) 23:468–78. doi: 10.1016/j.tim.2015.06.003
245. Mohd HA, Al-Tawfiq JA, Memish ZA. Middle East Respiratory Syndrome Coronavirus (MERS-CoV) Origin and Animal Reservoir. Virol J (2016) 13:87. doi: 10.1186/s12985-016-0544-0
246. Cotten M, Lam TT, Watson SJ, Palser AL, Petrova V, Grant P, et al. Full-Genome Deep Sequencing and Phylogenetic Analysis of Novel Human Betacoronavirus. Emerg Infect Dis (2013) 19:736–742B. doi: 10.3201/eid1905.130057
247. Kumar B, Manuja A, Gulati BR, Virmani N, Tripathi BN. Zoonotic Viral Diseases of Equines and Their Impact on Human and Animal Health. Open Virol J (2018) 12:80–98. doi: 10.2174/1874357901812010080
248. Younan M, Bornstein S, Gluecks IV. MERS and the Dromedary Camel Trade Between Africa and the Middle East. Trop Anim Health Prod (2016) 48:1277–82. doi: 10.1007/s11250-016-1089-3
249. Donnelly CA, Malik MR, Elkholy A, Cauchemez S, Van Kerkhove MD. Worldwide Reduction in MERS Cases and Deaths Since 2016. Emerg Infect Dis (2019) 25:1758–60. doi: 10.3201/eid2509.190143
250. Bisson IA, Ssebide BJ, Marra PP. Early Detection of Emerging Zoonotic Diseases With Animal Morbidity and Mortality Monitoring. Ecohealth (2015) 12:98–103. doi: 10.1007/s10393-014-0988-x
251. Allen T, Murray KA, Zambrana-Torrelio C, Morse SS, Rondinini C, Di Marco M, et al. Global Hotspots and Correlates of Emerging Zoonotic Diseases. Nat Commun (2017) 8:1124. doi: 10.1038/s41467-017-00923-8
252. Tiwari R, Dhama K, Sharun K, Iqbal Yatoo M, Malik YS, Singh R, et al. COVID-19: Animals, Veterinary and Zoonotic Links. Vet Q (2020) 40:169–82. doi: 10.1080/01652176.2020.1766725
253. Kuchipudi SV, Surendran-Nair M, Ruden RM, Yon M, Nissly RH, Nelli RK, et al. Multiple Spillovers and Onward Transmission of SARS-CoV-2 in Free-Living and Captive White-Tailed Deer (Odocoileus Virginianus). bioRxiv (2021) 119:1–8. doi: 10.1101/2021.10.31.466677
254. Artois M, Bengis R, Delahay RJ, Duchene M-J, Duff JP, Ferroglio E, et al. “Wildlife Disease Surveillance and Monitoring,”. In: Delahay RJ, Smith GC, Hutchings MR, editors. Managment of Diseases in Wild Mammals. Tokyo, Japan: Springer (2009). p. 187–213.
255. Bruckner GK. The Role of the World Organisation for Animal Health (OIE) to Facilitate the International Trade in Animals and Animal Products. Onderstepoort J Vet Res (2009) 76:141–6. doi: 10.4102/ojvr.v76i1.78
256. Kelly TR, Karesh WB, Johnson CK, Gilardi KV, Anthony SJ, Goldstein T, et al. One Health Proof of Concept: Bringing a Transdisciplinary Approach to Surveillance for Zoonotic Viruses at the Human-Wild Animal Interface. Prev Vet Med (2017) 137:112–8. doi: 10.1016/j.prevetmed.2016.11.023
257. Paull SH, Song S, Mcclure KM, Sackett LC, Kilpatrick AM, Johnson PT. From Superspreaders to Disease Hotspots: Linking Transmission Across Hosts and Space. Front Ecol Environ (2012) 10:75–82. doi: 10.1890/110111
258. Van Doorn HR. Emerging Infectious Diseases. Med (Abingdon) (2014) 42:60–3. doi: 10.1016/j.mpmed.2013.10.014
259. El-Sayed A, Abdel-Daim MM, Kamel M. Zoonotic and Anthropozoonotic Potential of COVID-19 and its Implications for Public Health. Environ Sci pollut Res Int (2021) 28:52599–609. doi: 10.1007/s11356-021-16415-8
260. Sharun K, Tiwari R, Natesan S, Dhama K. SARS-CoV-2 Infection in Farmed Minks, Associated Zoonotic Concerns, and Importance of the One Health Approach During the Ongoing COVID-19 Pandemic. Vet Q (2021) 41:50–60. doi: 10.1080/01652176.2020.1867776
261. Zoetis. Zoetis’ Emerging Infectious Disease Capabilities Support COVID-19 Solutions for Great Apes and Minks (2021). Available at: https://www.zoetis.com/news-and-media/feature-stories/posts/zoetis-emerging-infectious-disease-capabilities-support-covid-19-solutions-for-great-apes-and-minks.aspx (Accessed Nov 25 2021).
Keywords: SARS-CoV-2, intermediate hosts, emergence, coronavirus, spillover, variants, zoonosis, reverse zoonosis
Citation: Schindell BG, Allardice M, McBride JAM, Dennehy B and Kindrachuk J (2022) SARS-CoV-2 and the Missing Link of Intermediate Hosts in Viral Emergence - What We Can Learn From Other Betacoronaviruses. Front.Virol. 2:875213. doi: 10.3389/fviro.2022.875213
Received: 13 February 2022; Accepted: 06 April 2022;
Published: 07 July 2022.
Edited by:
Martin H. Groschup, Friedrich-Loeffler-Institute, GermanyReviewed by:
Ivan Toplak, University of Ljubljana, SloveniaAdam Cockrell, AavantiBio, Inc., United States
Copyright © 2022 Schindell, Allardice, McBride, Dennehy and Kindrachuk. This is an open-access article distributed under the terms of the Creative Commons Attribution License (CC BY). The use, distribution or reproduction in other forums is permitted, provided the original author(s) and the copyright owner(s) are credited and that the original publication in this journal is cited, in accordance with accepted academic practice. No use, distribution or reproduction is permitted which does not comply with these terms.
*Correspondence: Jason Kindrachuk, SmFzb24uS2luZHJhY2h1a0B1bWFuaXRvYmEuY2E=